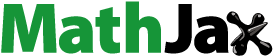
Abstract
Since double-suction centrifugal pumps consume quantities of energy, revealing critical factors for energy dissipation is helpful for energy-saving design. This study aims to investigate the relationship between energy dissipation and vortex based on Rortex method in a double-suction centrifugal pump. Detached eddy simulation was applied to obtain the flow field. Enstrophy was used to present the strength of the local rigid vortex and shear. The results indicate that the local shear dominates energy dissipation in the pump. Owing to jet flows, the energy loss on blade leading edges (LE) and trailing edges (TE) were 102-103 times that of the middle region at 0.4Qd and ten times at 1.4Qd. The energy dissipation on pressure sides (PS) was ten times greater than that on suction sides (SS) at the TE, while flow separation at the middle of SS caused by wake flow increased energy dissipation to nearly ten times that of PS. Jet-wake flow near volute inlet was the dominant factor for energy dissipation at part-load, while separation flows in volute discharge was more significant at overload. The induced high local shear strength was responsible for energy dissipation. Therefore, reducing local shear is a potential energy-saving approach in pumps.
Nomenclature
Symbols
D1 | = | Impeller inlet diameter [mm] |
D2 | = | Impeller outlet diameter [mm] |
D3 | = | Volute inlet diameter [mm] |
Dd | = | Volute outlet diameter [mm] |
Ds | = | Suction inlet diameter [mm] |
E | = | Enstrophy [s−2] |
H | = | Head [m] |
N | = | Grid node number [-] |
P | = | Power [W] |
Q | = | Flowrate [m3/h] |
Spro | = | Entropy dissipation rate [W·m−3·K−1] |
T | = | Temperature [K] |
Z | = | Blades number [-] |
b2 | = | Impeller outlet width [mm] |
b3 | = | Volute inlet width [mm] |
g | = | Gravitational acceleration [m·s−2] |
h | = | Grid element height [μm] |
n | = | Rotating speed of impeller [r/min] |
p | = | Pressure [Pa] |
s | = | Specific entropy [J·kg−1·K−1] |
t | = | Time [s] |
u | = | velocity in x-direction [m/s] |
v | = | velocity in y-direction [m/s] |
w | = | velocity in z-direction [m/s] |
ϵ | = | Dissipation rate of turbulent kinetic energy [m2·s−3] |
η | = | Efficiency [%] |
μ | = | Dynamic viscosity [kg·m−1·s−1] |
ρ | = | Density [kg/m3] |
Matrix symbol
R | = | Local rotational part of vorticity |
S | = | Local shear part of vorticity |
r | = | real unit eigenvector of the velocity gradient |
ω | = | Vorticity |
Abbreviations
CFD | = | Computational fluid dynamics |
DES | = | Detached eddy simulation |
LES | = | Large eddy simulation |
PS | = | Pressure side of the blade |
RANS | = | Reynolds-averaged Navier – Stokes |
SS | = | Suction side of the blade |
1. Introduction
Pumps consume over one-fifth of the energy generated, serving as the core of energy conversion in a fluid transportation system. The energy-saving orientation makes effective energy utilization and stable operation progressively important (Gan et al., Citation2022a; Gevorkov et al., Citation2022; Shankar et al., Citation2016). Undoubtedly, the hydraulic loss will directly lead to the performance drop of pumps (Gülich, Citation2008; Wang et al., Citation2017). Analyzing hydraulic loss and revealing the pump energy dissipation mechanism is essential to improve pump performance. The double-suction centrifugal pump is a special type of centrifugal pump with a distinct asymmetric suction chamber and double entrance impeller. With the advantages of large flowrate, high head, and inherent structure durability, these pumps have gained worldwide usage in domestic and industrial fields. However, their complex internal flow pattern might complicate the hydraulic loss inside. To improve efficiency, conducting a profound study on energy dissipation inside double-suction centrifugal pumps is essential.
Benefiting from the development of computational fluid dynamics (CFD) technology, the improved numerical method contributed to the accurate prediction of pump internal flow patterns. The hydraulic loss analysis method has developed from the primary empirical equations to the quantification and visualization approaches (Deng et al., Citation2021; Gu et al., Citation2019; Miao et al., Citation2018). Scholars have recognized that incidence, mixing of wake flow, friction in the near-wall region, and flow separations are the main sources of hydraulic loss (Liu et al., Citation2019; Lu et al., Citation2022; Posa & Lippolis, Citation2018; Qian et al., Citation2019; Tang et al., Citation2022; Tao et al., Citation2022). In addition, the vortex is another significant cause of hydraulic loss (Khait et al., Citation2018; Wang et al., Citation2022), and the relationship between energy dissipation and the vortex structure has been identified.
Considerable studies have been devoted to the perspective of vortex in hydraulic machinery in recent years. Cavazzini et al. (Citation2018) investigated the unstable flow pattern in a pump turbine under pump mode. They reported that the vortices in the vaned return channel blocked the passage and were responsible for the unstable behavior. Still, the detailed vortical structures were not investigated in the research. Ji et al. (Citation2020) indicated that vortices due to rotating stall and separation vortices in the annular volute casing of a mixed flow pump were strongly correlated with hydraulic loss represented by entropy production. Sun et al. (Citation2022) investigated the hydraulic loss in a bulb tubular pump using entropy production and provided a novel insight into hydraulic loss from energy balance equations. They stated that the tip leakage vortex was a weighty inducement of hydraulic loss in the impeller. However, the direct use of vorticity and streamline resulted in limited local vortex information and inaccurate identification. Considered as the kinetic energy of vorticity, Lin et al. (Citation2021) introduced the dissipation of enstrophy to describe the hydraulic loss in a pump-as-turbine (PAT), providing a new perspective on how vortical structures influence energy dissipation. However, the mathematic formulas of ‘enstrophy dissipation’ regarding RANS models were homogeneous with the entropy production. The rationality and feasibility of its mathematic and physical principles for quantifying hydraulic loss are still doubtful.
Obviously, the vortex is one of the dominant factors for hydraulic loss, and many researchers reported their findings about the effects of vortical structures on hydraulic loss and pump performance. Gan et al. (Citation2022b) optimized the curved inlet of a centrifugal pump and found that vortices at the impeller inlet section worsened pump performance. The suppressed vortices could improve impeller performance. Ghorani et al. (Citation2020) and Zhao et al. (Citation2022) indicated that the reduction of vortices in the impeller would effectively decrease hydraulic loss and improve pump efficiency in their optimization works. Thus, vortices influence the pump performance, and the vortex reduction design can effectively improve pump efficiency. The influence mechanism of vortical structures is not clarified by the ‘global method’ using streamline (Chakraborty et al., Citation2005). The detailed vortex structures are ignored because a series of adjacent nodes is necessary to build the streamline. Hence, to address the identification of local vortical structures, criteria such as Q (Hunt et al., Citation1988), λ2 (Jeong & Hussain, Citation1995), and Omega (Liu et al., Citation2016) were developed and proven practical in identifying vortical structures in hydraulic machinery (Qian et al., Citation2022; Zhang et al., Citation2020; Zhao et al., Citation2021). Guan et al. (Citation2020) reported that the mixing of wake vortices near the volute cutwater was one source of hydraulic loss in the double-suction centrifugal pump. Zhao et al. (Citation2023) found that the viscous effect due to the vortex evolution was the dominant factor for hydraulic loss based on the Q-criterion.
At present, the Rortex method has been proposed to describe the rigid rotation of fluid elements (Liu et al., Citation2019; Liu & Liu, Citation2019). The vorticity was decomposed into the local rigid vortex and local shear, and the real eigenvector of the velocity gradient tensor is considered the rotation axis of the local vortex. This method has been proven effective in hydraulic machinery (Wang et al., Citation2021), and the correlation between local rigid vortex and energy dissipation can be analyzed through this perspective. Yuan et al. (Citation2021) recommended the Omega and Rortex methods for accurate vortex structure identification. The energy loss in vortex regions was lower than in the surroundings. They also pointed out the local shear effect was more significant in energy loss (Yuan et al., Citation2022). Qin et al. (Citation2022) also indicated that energy dissipation was highly correlated with the local shear from the perspective of Rortex and enstrophy. On this basis, applying Rortex and enstrophy methods is practical in analyzing the correlation between vortical structure and hydraulic loss in turbomachinery.
The purpose of this paper is to reveal the relationship between energy dissipation and vortical structures inside a double-suction centrifugal pump. The detached eddy simulation (DES) method solves the internal flow field, and the experiment validates numerical results. The entropy production method is used for visualizing energy dissipation. The vortex identification is based on the Rortex by decomposing vorticity into the local rotational and shear parts. The correlation between energy dissipation, local rigid vortex, and shear in each hydraulic component is revealed with a special emphasis on enstrophy. Ultimately, a potential approach to loss reduction is proposed.
2. Definitions of energy dissipation and vortex
2.1. Definition of energy dissipation
2.1.1. Total pressure drop
The energy loss inside the pump can be defined as the difference between input and output power. The energy loss in centrifugal pumps consists of hydraulic loss, disk friction loss, and volumetric loss, and all three types of losses can be directly calculated in the simulation. The disk friction loss results from the friction of impeller sidewalls. Volumetric loss is caused by the circulation from the impeller outlet to the inlet through the sidewall gaps and the leakage into the outside of the pump (ignored in the simulation). The hydraulic loss can be defined by Eq.(1), where Ps is the pump shaft power; Pd and Pv are disk friction loss and volumetric loss, individually.
(1)
(1) Since the pump head is defined as the total pressure difference between the inlet and outlet, the hydraulic loss in each component can also be estimated by calculating the total pressure drop from the inlet to the outlet. The amount of hydraulic loss is also written in the form of head in this research. That of the stationery domain is calculated using Eq.(2). The amount of hydraulic loss in the impeller is defined by Eq.(3), where the first part is the input power of the impeller. The second part is the output power of the impeller calculated by the total pressure difference. The energy loss in a component is calculated using Eq. (4).
(2)
(2)
(3)
(3)
(4)
(4) In the equations, pT1 and pT2 are total pressure at the inlet and outlet. The total pressure calculation is based on the mass flow average value at each section;
is the mass flowrate. ρ is density; g is the gravitational acceleration.
2.1.2. Entropy production theory
According to the second thermodynamic law, entropy is the state variable representing the irreversible degree of any system. Entropy production is to determine energy dissipation in an irreversible process. For incompressible fluid and ignoring temperature change, the entropy production rate (Spro) is calculated using Eq.(5) (Kock & Herwig, Citation2004, Citation2005).
(5)
(5) In the above equation, T is the temperature; u, v, and w are velocity components in x, y, and z directions; μ is dynamic viscosity. Regarding RANS equations adopted in numerical simulations, the entropy production rate is decomposed into the time-averaged and fluctuating parts, shown in Eq.(6). The first term on the right is viscous dissipation, and the second is turbulent dissipation.
(6)
(6) All time-averaged variables can be calculated directly in the CFD post-process to solve the viscous dissipation. Kock and Herwig (Citation2005) used Eq.(7) as a practical calculation approach to solving the turbulent dissipation because RANS methods cannot calculate fluctuating variables.
(7)
(7) In the equation, ϵ is the turbulent kinetic energy dissipation rate. The quantity of entropy production in a particular domain is calculated using volume integral, shown in Eq.(8).
(8)
(8) Kock and Herwig (Citation2004) also found that RANS methods underestimated the entropy production in near-wall regions (y+<50). In order to overcome the shortage, Eq.(9) was proposed to calculate the near-wall entropy production rate (Li et al., Citation2017). The correction of entropy production in near-wall regions is still useful in DES method because the RANS method solves the flow field in near-wall regions. Its area integral is the near-wall entropy production, shown in Eq. (10).
(9)
(9)
(10)
(10) Where τW is the shear stress on the wall, and vW is the velocity close to the wall. Finally, the total energy loss from entropy production is the combination of Ppro,D and Ppro,W, shown in Eq.(11).
(11)
(11)
2.2. Definition of vortex
In this research, we adopted the principle of Rortex proposed by Liu et al. (Citation2019) for identifying vortical structures, by decomposing the vorticity to the local rigid rotation part R and the non-rotational shear part S, shown in Eqs.(12) and (13), where r is the real unit eigenvector of the velocity gradient (∇v).
(12)
(12)
(13)
(13) The magnitude R is calculated using Eq.(14), where λci is the imaginary part of the complex eigenvalue of the velocity gradient tensor (Wang et al., Citation2019).
(14)
(14) The normalized Rortex identification method is introduced by calculating variable ΩR, defined in Eq.(15).
(15)
(15) Where λcr is the real part of the complex eigenvalue of ∇v, and λr is the real eigenvalue of ∇v; ϵ0 is a small value added to avoid being divided by zero.
This study introduces the concept of enstrophy to quantify the kinetic energy that a vortex structure contains, defined in Eq.(16) (Qin et al., Citation2022; Wu et al., Citation1999). The kinetic energy that R and S contain can be expressed in this form, shown in Eqs.(17) and (18).
(16)
(16)
(17)
(17)
(18)
(18)
3. Numerical simulation and experimental validation
3.1. Pump model
The research was conducted on a double-suction centrifugal pump with the specific speed of 91.6 (ns = 3.65n (0.5Qd)0.5/Hd0.75) and nominal pump shaft power (Ps) of 65 kW. The main parameters are listed in Table . The overall computational domain included an asymmetric suction chamber, a double-suction impeller without a central rib and staggered arrangement, and a volute casing, as described in Figure . The three-dimensional Cartesian coordinate system illustrated in Figure (b) was established for description. The circumferential position θ was defined as the angle with the x direction, and the impeller rotated along the positive θ direction.
Table 1. Main parameters of model pump.
3.2. Mesh generation and grid convergence analysis
The pre-treatment was conducted using ICEM CFD for mesh generation. Due to the complexity of the suction chamber, a hybrid mesh of tetrahedral and hexahedral elements was generated. The near-wall mesh was refined by 20 layers of trigonal prisms. Hexahedral elements were generated for the impeller and volute using the multi-block strategy to refine near-wall elements.
The sensitivity of the grid on numerical simulation is extremely significant to ensure accuracy and reliability in solving complex situations in turbomachinery. We adopted the grid convergence index (GCI) based on the Richardson extrapolation method to quantify the uncertainties and errors from discretization in CFD (Celik et al., Citation2008; Roache, Citation1997). Following this method, three grid schemes were generated, and the details of each scheme are listed in Table . The h1 (in μm) is the height of the first layer grid elements; N (in million) is the node number. The grid refinements of each scheme were carried out with a constant ratio (h1,i + 1/h1,i = 1.3, i = 1,2) and the same growth ratio in the normal direction of the wall. Furthermore, the elements in the core region are also generated with the same ratio of hi + 1/hi = 1.3 to achieve constant refinement.
Table 2. Grid number of each component.
The grid convergence situation is shown in Table based on steady-state simulations. The pump head was used for calculating the GCI. The relative error of the fine grid (Grid 1) was 0.23%, and the GCI21 was 0.29%. Finally, the fine grid with 19.168 million nodes was selected for the following numerical investigation. The average value of y + was 14.8 for the impeller, 5.1 for the volute casing, and 1.3 for the suction chamber. The mesh structures are shown in Figure .
Table 3. Grid convergence analysis.
3.3. Numerical setup
In this study, ANSYS CFX was adopted to solve the complex flow characteristics in the model pump. SST k-ω turbulence model was adopted to solve the RANS equations under steady state. The hybrid RANS/LES method, detached eddy simulation (DES) with SST k-ω turbulence model, was used to simulate the transient state. In the simulation, SST k-ω model solved the near wall region, and LES solved the main flow region to obtain high-fidelity dynamics coherent structures via the Smagorinsky model (ANSYS, Citation2020; Posa, Citation2021; Yuan et al., Citation2022). Compared with RANS models and LES, more accurate flow details can be obtained, and the requirement for near-wall grid quantities is lower.
Boundary conditions and computational setup are listed in Table . The inlet boundary was considered as Total Pressure. The outlet condition was set as Mass Flow Rate to control the operating condition. The roughness of hydraulic surfaces was Ra12.5 for the impeller sidewalls and Ra50 for others. The equivalent sand grain diameters were calculated following Adams et al. (Citation2012). Interfaces between rotational and stationary domains were ‘Frozen Rotor’ under steady state and were changed to ‘Transient rotor-stator’ under transient state to satisfy the transient rotor-stator interaction at sliding interfaces. The steady state simulations iterated 500 steps until they met the target, and then the results were taken to initialize the transient simulation. The maximum iteration number of each step was 5 for time consumption and accuracy.
Table 4. Boundary conditions and computational setup.
3.4. Experimental setup
The model pump was tested experimentally to confirm the accuracy of the numerical simulation. The experiments were carried out on an open test rig. Figure illustrates the 3-D schematic diagram of the test rig. A motor drove the tested pump with a nominal power of 90 kW and a rotation speed of 1480 r/min. The motor power was measured in the experiment, and the current and voltage measurement precisions were 0.5% of the full scale. The precision of the electromagnetic flowmeter was 0.25% of the measured value, and those of the inlet and outlet pressure transmitters were 0.1% of the full scale. Furthermore, the lengths of all pipes between these instruments were long enough to ensure uniform flow in these sensors.
4. Result and discussion
4.1. Comparison between experiment and simulation
The comparison between experimental and numerical performance characteristics and the error analysis is illustrated in Figure . The errors between experimental and numerical values were calculated by Eq.(19).
(19)
(19) The minimum error of the head existed at 1.2Qd with the value of – 1.20%. The maximum value was – 4.67% under the design condition. The maximum errors of efficiency and power occurred under 1.4Qd with values of 8.63% and – 4.46%, respectively. The large efficiency deviation might result from the increasing mechanical and leakage losses, such as friction and leakage, which were not considered in the numerical simulation. As a key index for numerical accuracy, the head deviation was within the reasonable range (Gan et al., Citation2022b; Gülich, Citation2008). Thus, numerical simulation results are accurate enough for analyzing internal flow fields.
4.2. Energy dissipation analysis
Before analyzing entropy production, the practicability of this method was verified by comparing the hydraulic loss calculated by different methods following Section 2.1. The comparison of the hydraulic loss calculated by entropy production and total pressure drop is illustrated in Figure (a). The hydraulic loss estimated by entropy production has a similar variation trend as that by total pressure drop. The suction chamber lost the least power compared with impeller and volute. The gap between the impeller and volute was not huge in the range 0.4-1.2Qd, but the energy loss in volute increased dramatically at 1.4Qd. The entropy production contributed more than 74.6% of the total pressure drop in the suction chamber, 83.0%−92.4% in the volute casing, and 74.1%−88.3% in the impeller.
Figure 5. Validation of entropy method. (a) Entropy production in each component, compared with total pressure drop; (b) Total hydraulic loss calculated by different methods.

Figure (b) compares entropy production (Eq.(11)), total pressure drop (Eq.(4)), and power loss (Eq.(1)). The presented three energy loss evaluation methods obtained the same variation trend. The hydraulic loss calculated using total pressure drop and power loss was similar under most operating conditions. Thus, these two methods were proven almost equivalent in calculating the hydraulic loss of this kind of pump. Hydraulic loss calculated using entropy production was 82.19% of the power loss at 0.4Qd, and maximum value was 88.49% under the design condition. The deviation between entropy production and these losses was within the acceptable range. Therefore, entropy production generated from the numerical results can be further used for visualized energy dissipation analysis.
4.3. Dissipation and local rigid vortex in the suction chamber
As the suction chamber obtained its highest energy loss at 0.4Qd, while the loss was tiny when the flowrate increased, the energy dissipation and vortex distribution at 0.4Qd is analyzed in detail. The comparison of energy dissipation and vortex on plane z = 100 mm is shown in Figure . As illustrated in Figure (a), energy dissipation was generated near the outlet of the suction chamber in an annual region, and global vortices can be observed near the outlet. The regions in the negative x region seemed to have slightly higher energy dissipation than the other part.
Figure 6. Distribution of energy dissipation and vortex in the suction chamber at 0.4Qd. (a) Entropy production; (b) ΩR; (c) Enstrophy of local rigid vortex; (d) Enstrophy of shear.

As the vorticity decomposition enhances the concepts of local rigid vortex and shear, the relationship of energy dissipation, vortex, and shear was further revealed. Comparing Figure (b) – (d), the energy dissipation near the suction rib was the combined action of vortex and shear. Nevertheless, the local shear was the dominant factor for energy dissipation. Although regions upstream of the outlet and 315°<θ<360° presented high relative vortex strength, the enstrophy of the local rigid vortex was negligible, which meant the absolute strength of vortices in this region was weak and did not dissipate too much kinetic energy.
It is clear that entropy production and enstrophy of shear concentrated near θ = 90°, 180°, and 270°, so we selected two planes for further analysis. Figure (a) illustrates the position of Planes SA1 and SA2. As shown in Figure (b), energy dissipation generally appeared at the outer margin of the outlet, where backflows occurred. Backflows were the main factor for the energy dissipation here, and vortices were generated between the main flows and backflows. Vortices did not cover the high energy dissipation regions, comparing Figure (b) and (c). As shown in Figure (d), the effects of the local rotation were too weak to be captured on Plane SA1. The vortex on Plane SA2 marked in red did not present high rotation strength, while that in black was of higher strength. The energy dissipation might result from the interaction between the two vortices. Figure (e) indicates that high local shear strength was generated between the vortices and the wall. The energy dissipation on the two sections highly correlated with local shear, even though the regions with high local shear strength near the hub did not present high dissipation. The high local shear intensity in the backflow regions is strongly connected with energy dissipation, while the local rigid rotational intensity is weak.
Figure 7. Distribution of energy dissipation and vortex on Planes SA1 and SA2. (a) Plane SA1 and SA2; (b) Entropy production; (c) ΩR; (d) Enstrophy of local rigid vortex; (e) Enstrophy of shear.

Figure shows the three-dimensional vortex core distribution in the suction chamber with the threshold of ΩR = 0.8. The vortex core regions appeared where the velocity streamline of backflows swirled, and it also proved the accuracy of the Rortex method of the local rigid vortex. The swirling recirculation from the impeller coincided with the high energy dissipation, proving these backflows were the main reason for energy loss. Although the x > 0 part of the suction chamber appeared with more swirling streamlines and vortex cores, they contribute little to the energy dissipation here. Thus, the backflows from the outlet in the suction chamber and the induced high shear strength caused the energy dissipation.
4.4. Dissipation and local rigid vortex in impeller
The situation in the impeller is presented on the plane z = 13 mm. The energy dissipation in the impeller is illustrated in Figure (a). Energy dissipation was mainly generated near the impeller outlet at 0.4Qd, and relatively higher dissipation appeared near the inlet of the blade suction side (SS). Vortices and backflows occurred at the impeller outlet, leading to higher energy dissipation. As the flowrate increased, the high energy dissipation near the impeller outlet disappeared, and that near the blade suction side increased. Figured out by the velocity streamlines, obvious jet-wake flow structures can be found in all blade channels. Fluid elements separated from the SS, and the separation point moved towards the blade trailing edge. The separation flows caused high energy dissipation, especially in the blade channel close to the volute cutwater. It seems that both backflows at the outlet and jet flow near the blade trailing edge resulted in the high energy dissipation at 0.4Qd, while the weakened backflows at 1.0Qd and 1.4Qd led to the relief of energy dissipation here.
Figure 9. Distribution of energy dissipation and vortex in impeller. (a) Entropy production; (b) ΩR; (c) Enstrophy of local rigid vortex; (d) Enstrophy of shear.
As shown in Figure (b), vortices can be identified near the separation flows and pressure side (PS) of blade trailing edges at 0.4Qd, which might lead to a rise in energy dissipation. The vortices marked by red decayed when the blade moved away from the volute cutwater along with the dissipation rate. Thus, the local rigid vortex strength and energy dissipation are influenced by the rotor-stator interaction. Compared with the enstrophy contours, both rotational and shear effects caused energy dissipation, while the shear effect might be dominant.
With the increasing flowrate, an interesting phenomenon occurs: higher local rigid vortex intensity can be observed at 1.0Qd, but fewer vortical structures can be captured at 1.4Qd. The absolute local rigid vortex intensity represented by the enstrophy indicated that although the area of high-value regions increased at 1.0Qd, the extreme high enstrophy regions decreased, and that of the shear effects increased. Still, both effects seemed not to influence energy dissipation in the investigated region. The separated wake flows did not generate vortical structures at 1.4Qd, and no apparent vortical structures were identified except marked by yellow circles in the center of the blade passage. The high absolute strength of the local rigid vortex and shear strongly correlated with the energy dissipation here. But such a high dissipation region disappeared in other channels. Conclusively, the combined effects of local rigid vortex and shear contributed to the energy dissipation, and the shear effect was the dominant factor.
Figure shows the averaged energy dissipation in one rotating period on the blade surface in the streamwise direction on their middle span location. The energy dissipation at the blade leading edge (LE) and trailing edge (TE) is nearly 102-103 of the middle region at 0.4Qd, and ten times at 1.4Qd. At the LE, the SS dissipated ten times more energy than the PS at 0.4Qd, while the opposite occurred at 1.4Qd. It might result from the incidence angle variation at the LE, which was positive (blade angle larger than flow angle) at part-load and negative at overload. The incidence on the blade surface probably increased energy dissipation. The PS always had more dissipation near the TE under both conditions, nearly ten times at 0.4Qd and thirty times at 1.4Qd. The jet flow with high velocity might lead to a higher dissipation rate near the trailing edge. Still, a large area of high dissipation existed in the wake flow region, slightly different from the previous research (Yuan et al., Citation2022). The dissipation rate on the PS increased substantially when close to the trailing edge. A high dissipation rate on the SS also existed in the streamwise range of 0.4 to 0.6, marked by red, which might result from the separated flow on the SS compared with Figure .
4.5. Dissipation and local rigid vortex in the volute casing
Figure shows the situation in volute on plane z = 0 under the flowrate of 0.4Qd and 1.4Qd, as the highest hydraulic loss under these two operating conditions. At 0.4Qd, energy dissipation was mainly generated near the volute inlet, and the extremely high dissipation rate was concentrated at the inside of the volute cutwater. Furthermore, high dissipation regions were mainly located near blade trailing edges and might result from the jet-wake flow. On the contrary, the volute discharge appeared with high energy dissipation at 1.4Qd.
Figure 11. Distribution of energy dissipation and vortex in volute. (a) Entropy production; (b) ΩR; (c) Enstrophy of local rigid vortex; (d) Enstrophy of shear.
Comparing Figure (b) – (d), high intensity of both local rigid vortex and shear was found in the volute channel at 0.4Qd. Previous research indicated that energy dissipation could be connected with the vortex, especially near the volute cutwater (Guan et al., Citation2020). Here a detailed explanation could be raised that the shear effect might be more dominant in energy dissipation compared with the rotational part from the perspective of the local rigid vortex. The high dissipation region caused by jet-wake flow did not lead to high intensity of local rigid vortex, but high local shear existed. For the situation at 1.4Qd, high vortex and shear intensity concentrated in the volute discharge and volute channel within the – 70°<θ<90°. It could be illustrated clearly that the high intensity of local rotation and shear did not lead to the increasing energy dissipation in the volute channel under the presented condition. Still, the flow separation at the outside of the volute cutwater caused high local rigid vortex and shear strength and resulted in significant energy dissipation. The vortices near the outlet provided another explanation: momentum exchange between the vortices, backflows, and main flows led to high shear intensity, resulting in energy dissipation growth. Furthermore, three planes in volute discharge were chosen to illustrate energy dissipation inside.
Figure (a) shows that high energy dissipation is concentrated near the volute cutwater at 0.4Qd. The detailed flow field and contours in this region are illustrated in Figure . Marked in the red circle, high energy dissipation appeared with high velocity and backflows near the volute inlet, and high intensity of local shear was located at the same position. Flow separation occurred at the volute cutwater and increased local shear and rigid vortex. Marked in the black circle, high dissipation region existed as a high-velocity region near the blade trailing edge. However, the local rigid vortex and shear can not explain the high energy dissipation here. The high velocity might result from the recirculation in the clearance between the impeller tip and volute cutwater. The blade accelerated the fluid and increased the velocity gradient owing to the jet flow. Furthermore, extremely high energy dissipation was found in the near-wall region with high velocity gradient and local shear intensity. The reduction of velocity gradient in the near-wall region might effectively reduce hydraulic loss inside the volute.
Figure 12. Distribution of energy dissipation, velocity, and vortex near volute cutwater at 0.4Qd. (a) Entropy production; (b) Velocity; (c) Enstrophy of local rigid vortex (d) Enstrophy of shear.

The distribution of energy dissipation in volute discharge is shown in Figure (a). The high dissipation region was close to the volute cutwater with separated flows from the wall in the whole section on Plane VD1. Twisted streamlines seemed to result from the vortices, but they seemed not strong enough to generate more energy loss according to the enstrophy distribution. Only the high strength of the local rigid vortex, marked by red in Figure (c), seemed to influence energy dissipation but was still weaker than the shear. When the fluids approached the outlet of volute discharge, swirling streamlines appeared on Planes VD2 and VD3 in circles, and distinct vortical structures could be identified. High dissipation was also in coincidence with these vortices. Vortices 1 and 2 were less effective than the shear compared with vortex 3, whose contribution seemed equal to the shear effect. Additionally, the high dissipation regions near the wall also appeared with high local shear intensity. The shear effect in the near-wall region would result in more energy dissipation, and the reduction of local shear intensity in the near-wall region can improve pump performance. In brief, although the local rigid vortex might have contributed more to the energy dissipation in volute discharge than in the volute channel, the correlation between the shear effect and energy dissipation was more significant.
Figure 13. Distribution of energy dissipation and vortex in volute discharge at 1.4Qd. (a) Entropy production; (b) ΩR; (c) Enstrophy of local rigid vortex; (d) Enstrophy of shear.
4. Conclusion
With the application of the hybrid RANS/LES method to solving the internal flow field of a double-suction centrifugal pump, the energy dissipation was visualized by entropy production, and vortex structure was identified by the Rortex method as the decomposition of vorticity. The correlation between energy dissipation and local rigid vortex and shear was revealed. Enstrophy was employed to represent the strength of the local rigid vortex and shear. Based on the findings, conclusions are drawn:
Backflows near the outlet and the induced vortices are the main causes of energy dissipation in the suction chamber. The local shear part is more significant in energy dissipation in the backflow region. Still, it does not represent higher energy dissipation if high local shear is out of the backflow region.
High local shear intensity from jet-wake flow and backflows correlates more significantly with the dissipation rate. The energy dissipation near the blade leading edge is influenced by flowrate owing to the incidence angle variation. The trailing edge of the pressure side consumes ten times the energy of the suction side at 0.4Qd and thirty times at 1.4Qd, owing to the jet-wake flow structure. The flow separation of wake flow from the suction side increases energy loss in the middle region by approximately ten times.
Jet-wake flow near the volute inlet at part-load and separated flow in volute discharge at overload were two main factors for the energy dissipation in the volute casing. The induced high local shear is dominant in energy loss. Furthermore, the local shear in the near-wall region of the volute casing plays a crucial role in energy dissipation, owing to the high velocity gradient in the near-wall region. Reducing local shear is a potential energy-saving approach in pumps, especially in the collector, such as a volute casing.
Conclusively, local shear is crucial for energy dissipation inside the investigated pump. Two practical ways could improve performance: optimizing blade profiles to reduce flow separations inside the impeller and reducing velocity gradient in the near-wall regions by reducing wall roughness.
Disclosure statement
No potential conflict of interest was reported by the author(s).
Data availability
The data that support the findings of this study are available from the corresponding author upon reasonable request.
Additional information
Funding
References
- Adams, T., Grant, C., & Watson, H. (2012). A simple algorithm to relate measured surface roughness to equivalent sand-grain roughness. International Journal of Mechanical Engineering and Mechatronics, 1(2), 66–71. https://doi.org/10.11159/ijmem.2012.008
- ANSYS. (2020). ANSYS CFX-Solver theory guide release 2020 R2. ANSYS, Inc.
- Cavazzini, G., Houdeline, J. B., Pavesi, G., Teller, O., & Ardizzon, G. (2018). Unstable behaviour of pump-turbines and its effects on power regulation capacity of pumped-hydro energy storage plants. Renewable and Sustainable Energy Reviews, 94, 399–409. https://doi.org/10.1016/j.rser.2018.06.018
- Celik, I. B., Ghia, U., Roache, P. J., & Freitas, C. J. (2008). Procedure for estimation and reporting of uncertainty due to discretization in CFD applications. Journal of Fluids Engineering-Transactions of the ASME, 130, 7.
- Chakraborty, P., Balachandar, S., & Adrian, R. J. (2005). On the relationships between local vortex identification schemes. Journal of Fluid Mechanics, 535, 189–214. https://doi.org/10.1017/S0022112005004726
- Deng, Q., Pei, J., Wang, W., Lin, B., Zhang, C., & Zhao, J. (2021). Energy loss and radial force variation caused by impeller trimming in a double-suction centrifugal pump. Entropy, 23(9), 1228. https://doi.org/10.3390/e23091228
- Gan, X., Pavesi, G., Pei, J., Yuan, S., Wang, W., & Yin, T. (2022b). Parametric investigation and energy efficiency optimization of the curved inlet pipe with induced vane of an inline pump. Energy, 240, 122824. https://doi.org/10.1016/j.energy.2021.122824
- Gan, X., Pei, J., Pavesi, G., Yuan, S., & Wang, W. (2022a). Application of intelligent methods in energy efficiency enhancement of pump system: A review. Energy Reports, 8, 11592–11606. https://doi.org/10.1016/j.egyr.2022.09.016
- Gevorkov, L., Domínguez-García, J. L., & Romero, L. T. (2022). Review on solar photovoltaic-powered pumping systems. Energies, 16(1), 94. https://doi.org/10.3390/en16010094
- Ghorani, M. M., Haghighi, M. H. S., & Riasi, A. (2020). Entropy generation minimization of a pump running in reverse mode based on surrogate models and NSGA-II. International Communications in Heat and Mass Transfer, 118, 104898. https://doi.org/10.1016/j.icheatmasstransfer.2020.104898
- Gu, Y., Pei, J., Yuan, S., Wang, W., Zhang, F., Wang, P., & Liu, Y. (2019). Clocking effect of vaned diffuser on hydraulic performance of high-power pump by using the numerical flow loss visualization method. Energy, 170, 986–997. https://doi.org/10.1016/j.energy.2018.12.204
- Guan, H., Jiang, W., Yang, J., Wang, Y., Zhao, X., & Wang, J. (2020). Energy loss analysis of the double-suction centrifugal pump under different flow rates based on entropy production theory. Proceedings of the Institution of Mechanical Engineers, Part C: Journal of Mechanical Engineering Science, 234(20), 4009–4023. https://doi.org/10.1177/0954406220919795
- Gülich, J. F. (2008). Centrifugal pumps. Springer.
- Hunt, J. C., Wray, A. A., & Moin, P. (1988). Eddies, streams, and convergence zones in turbulent flows. Studying Turbulence Using Numerical Simulation Databases, 2. Proceedings of the 1988 summer program.
- Jeong, J., & Hussain, F. (1995). On the identification of a vortex. Journal of Fluid Mechanics, 285, 69–94. https://doi.org/10.1017/S0022112095000462
- Ji, L., Li, W., Shi, W., Tian, F., & Agarwal, R. (2020). Diagnosis of internal energy characteristics of mixed-flow pump within stall region based on entropy production analysis model. International Communications in Heat and Mass Transfer, 117, 104784. https://doi.org/10.1016/j.icheatmasstransfer.2020.104784
- Khait, A., Noskov, A., Alekhin, V., & Bianco, V. (2018). Analysis of the local entropy generation in a double-circuit vortex tube. Applied Thermal Engineering, 130, 1391–1403. https://doi.org/10.1016/j.applthermaleng.2017.11.136
- Kock, F., & Herwig, H. (2004). Local entropy production in turbulent shear flows: A high-reynolds number model with wall functions. International Journal of Heat and Mass Transfer, 47(10-11), 2205–2215. https://doi.org/10.1016/j.ijheatmasstransfer.2003.11.025
- Kock, F., & Herwig, H. (2005). Entropy production calculation for turbulent shear flows and their implementation in CFD codes. International Journal of Heat and Fluid Flow, 26(4), 672–680. https://doi.org/10.1016/j.ijheatfluidflow.2005.03.005
- Li, D., Wang, H., Qin, Y., Han, L., Wei, X., & Qin, D. (2017). Entropy production analysis of hysteresis characteristic of a pump-turbine model. Energy Conversion and Management, 149, 175–191. https://doi.org/10.1016/j.enconman.2017.07.024
- Lin, T., Li, X., Zhu, Z., Xie, J., Li, Y., & Yang, H. (2021). Application of enstrophy dissipation to analyze energy loss in a centrifugal pump as turbine. Renewable Energy, 163, 41–55. https://doi.org/10.1016/j.renene.2020.08.109
- Liu, C., Gao, Y. S., Dong, X. R., Wang, Y. Q., Liu, J. M., Zhang, Y. N., & Gui, N. (2019). Third generation of vortex identification methods: Omega and liutex/rortex based systems. Journal of Hydrodynamics, 31(2), 205–223. https://doi.org/10.1007/s42241-019-0022-4
- Liu, C., Wang, Y., Yang, Y., & Duan, Z. (2016). New omega vortex identification method. Science China Physics. Mechanics & Astronomy, 59, 1–9. https://doi.org/10.1007/s11433-016-0022-6
- Liu, J., & Liu, C. (2019). Modified normalized rortex/vortex identification method. Physics of Fluids, 31(6), 061704. https://doi.org/10.1063/1.5109437
- Liu, M., Tan, L., & Cao, S. (2019). Theoretical model of energy performance prediction and BEP determination for centrifugal pump as turbine. Energy, 172, 712–732. https://doi.org/10.1016/j.energy.2019.01.162
- Lu, Z., Xiao, R., Tao, R., Li, P., & Liu, W. (2022). Influence of guide vane profile on the flow energy dissipation in a reversible pump-turbine at pump mode. Journal of Energy Storage, 49, 104161. https://doi.org/10.1016/j.est.2022.104161
- Miao, S., Yang, J., Shi, F., Wang, X., & Shi, G. (2018). Research on energy conversion characteristic of pump as turbine. Advances in Mechanical Engineering, 10(4), 168781 401877083. https://doi.org/10.1177/1687814018770836
- Posa, A. (2021). LES study on the influence of the diffuser inlet angle of a centrifugal pump on pressure fluctuations. International Journal of Heat and Fluid Flow, 89, 108804. https://doi.org/10.1016/j.ijheatfluidflow.2021.108804
- Posa, A., & Lippolis, A. (2018). A LES investigation of off-design performance of a centrifugal pump with variable-geometry diffuser. International Journal of Heat and Fluid Flow, 70, 299–314. https://doi.org/10.1016/j.ijheatfluidflow.2018.02.011
- Qian, B., Cai, Y., Ding, Q., Zhao, D., Sun, W., & Wang, L. (2022). Investigation of Tip leakage vortex structure and trajectory in a centrifugal pump with a New omega vortex identification method. Applied Sciences, 12(10), 5270. https://doi.org/10.3390/app12105270
- Qian, B., Chen, J., Wu, P., Wu, D., Yan, P., & Li, S. (2019). Investigation on inner flow quality assessment of centrifugal pump based on Euler head and entropy production analysis. In IOP conference series: Earth and environmental science (Vol. 240, No. 9, p. 092001). IOP Publishing. https://doi.org/10.1088/1755-1315/240/9/092001.
- Qin, Y., Li, D., Wang, H., Liu, Z., Wei, X., & Wang, X. (2022). Investigation on the relationship between hydraulic loss and vortex evolution in pump mode of a pump-turbine. Journal of Hydrodynamics, 34(4), 555–569. https://doi.org/10.1007/s42241-022-0053-0
- Roache, P. J. (1997). Quantification of uncertainty in computational fluid dynamics. Annual Review of Fluid Mechanics, 29(1), 123–160. https://doi.org/10.1146/annurev.fluid.29.1.123
- Shankar, V. K. A., Umashankar, S., Paramasivam, S., & Hanigovszki, N. (2016). A comprehensive review on energy efficiency enhancement initiatives in centrifugal pumping system. Applied Energy, 181, 495–513. https://doi.org/10.1016/j.apenergy.2016.08.070
- Sun, L., Pan, Q., Zhang, D., Zhao, R., & van Esch, B. B. (2022). Numerical study of the energy loss in the bulb tubular pump system focusing on the off-design conditions based on combined energy analysis methods. Energy, 258, 124794. https://doi.org/10.1016/j.energy.2022.124794
- Tang, X., Jiang, W., Li, Q., Hou, G., Zhang, N., Wang, Y., & Chen, D. (2022). Analysis of hydraulic loss of the centrifugal pump as turbine based on internal flow feature and entropy generation theory. Sustainable Energy Technologies and Assessments, 52, 102070. https://doi.org/10.1016/j.seta.2022.102070
- Tao, R., Li, P., Yao, Z., & Xiao, R. (2022). Investigation of the flow energy dissipation law in a centrifugal impeller in pump mode. Proceedings of the Institution of Mechanical Engineers, Part A: Journal of Power and Energy, 236(2), 260–272. https://doi.org/10.1177/09576509211034976
- Wang, C., Shi, W., Wang, X., Jiang, X., Yang, Y., Li, W., & Zhou, L. (2017). Optimal design of multistage centrifugal pump based on the combined energy loss model and computational fluid dynamics. Applied Energy, 187, 10–26. https://doi.org/10.1016/j.apenergy.2016.11.046
- Wang, C., Zeng, Y., Yao, Z., & Wang, F. (2021). Rigid vorticity transport equation and its application to vortical structure evolution analysis in hydro-energy machinery. Engineering Applications of Computational Fluid Mechanics, 15(1), 1016–1033. https://doi.org/10.1080/19942060.2021.1938685
- Wang, Y., Gao, Y., Liu, J., & Liu, C. (2019). Explicit formula for the liutex vector and physical meaning of vorticity based on the liutex-shear decomposition. Journal of Hydrodynamics, 31(3), 464–474. https://doi.org/10.1007/s42241-019-0032-2
- Wang, Z., Xie, B., Xia, X., Yang, H., Zuo, Q., & Liu, Z. (2022). Energy loss of radial inflow turbine for organic rankine cycle using mixture based on entropy production method. Energy, 245, 123312. https://doi.org/10.1016/j.energy.2022.123312
- Wu, J., Zhou, Y., & Fan, M. (1999). A note on kinetic energy, dissipation and enstrophy. Physics of Fluids, 11(2), 503–505. https://doi.org/10.1063/1.869866
- Yuan, Z., Zhang, Y., Wang, C., & Lu, B. (2021). Study on characteristics of vortex structures and irreversible losses in the centrifugal pump. Proceedings of the Institution of Mechanical Engineers, Part A: Journal of Power and Energy, 235(5), 1080–1093. https://doi.org/10.1177/0957650920983061
- Yuan, Z., Zhang, Y., Zhou, W., & Wang, C. (2022). Hydraulic loss analysis in a pump-turbine with special emphasis on local rigid vortex and shear. Physics of Fluids, 34(12), 125101. https://doi.org/10.1063/5.0124552
- Zhang, N., Jiang, J., Gao, B., Liu, X., & Ni, D. (2020). Numerical analysis of the vortical structure and its unsteady evolution of a centrifugal pump. Renewable Energy, 155, 748–760. https://doi.org/10.1016/j.renene.2020.03.182
- Zhao, J., Pei, J., Yuan, J., & Wang, W. (2022). Energy-saving oriented optimization design of the impeller and volute of a multi-stage double-suction centrifugal pump using artificial neural network. Engineering Applications of Computational Fluid Mechanics, 16(1), 1974–2001. https://doi.org/10.1080/19942060.2022.2127913
- Zhao, X., Chen, J., Huang, B., & Wang, G. (2021). The identification of tip leakage vortex of an axial flow waterjet pump by using omega method and liutex. In C. Liu & Y. Wang (Eds.), Liutex and third generation of vortex definition and identification: An invited workshop from chaos 2020 (pp. 419–427). Springer International Publishing. https://doi.org/10.1007/978-3-030-70217-5_28
- Zhao, X., Shen, X., Zhang, D., Xu, B., & van Esch, B. B. (2023). Numerical investigation on hydrodynamic performance of a pre-swirl stator pump-jet propulsor with special emphasis on energy loss mechanism. Ocean Engineering, 272, 113836. https://doi.org/10.1016/j.oceaneng.2023.113836