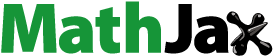
Abstract
Insufficiently studied, the phenomenon of twisted wind is prevalent in mountainous regions. This study focuses on investigating the characteristics and aerodynamics of a single high-rise building subjected to a twisted wind field (TWF). By comparing the 0° conventional wind field (CWF) with two TWFs (TWF15 and TWF 30) with maximum twisted angle of 15° and 30°, both wind tunnel testing and computational fluid dynamics (CFD) are employed. A comprehensive analysis is conducted to examine the differences between TWFs and CWF in terms of wind profiles, turbulence, aerodynamic characteristics of single high-rise buildings, and wake vortex morphology. The results demonstrate notable disparities between TWF and CWF. Particularly, the twisted wind induces inflection in the wind profile, leading to significant acceleration or deceleration. Moreover, the twisted effect generates an asymmetric distribution of average building surface pressure, thereby introducing the potential for asymmetric or torsional loading extremes. Phenomenologically, the twisted effect weakens shear layer separation, creates squeezed separation bubbles, and promotes premature reattachment, resulting in distinct flow field morphologies compared to CWF. Furthermore, as the wind twisted angle increases, the energy concentration associated with Bernard-Karman shedding diminishes, thereby significantly altering the wake characteristics.
Notations
v | = | Lateral velocity (m/s) |
u | = | Streamwise velocity (m/s) |
w | = | Velocity in directions z (m/s) |
Vgr | = | Resultant velocity of incoming flow (m/s) |
CWF | = | Conventional wind field |
TWF15 | = | Twisted wind field 15° |
TWF30 | = | Twisted wind field 30° |
CFD | = | Computational fluid dynamics |
LES | = | Large eddy simulation |
WT | = | Wind tunnel |
t | = | Time (s) |
= | Pressure coefficient at measurement node i at time instant t | |
= | Wind pressure at measurement point i at time t (Pa) | |
= | Total pressure (Pa) | |
= | Static pressure (Pa) | |
H | = | Structural height (mm) |
CL(z) | = | RMS lift coefficient |
= | RMS lift (N) | |
= | Air density (kg/m3) | |
= | Velocity at the top of structure (m/s) | |
A(z) | = | Projected area of the windward surface (mm2) |
z | = | Height (mm) |
S | = | Fluctuating spectrum |
f | = | Frequency (Hz) |
= | Variance of lift | |
A | = | Symmetric tensor |
B | = | Antisymmetric tensor |
θ | = | Twisted angle (°) |
1. Introduction
Nowadays, towering edifices that progressively punctuate a city's skyline are often regarded as the embodiment of its economic and technological prosperity. The slender and flexible high aspect-ratio, ultra-tall civil structures make wind load one of the most pivotal indices of building safety and comfort (Ng, Citation2017). Furthermore, China boasts an expansive expanse of land, encompassing a vast territory characterized by sprawling mountainous terrain spanning nearly 6.66 million square kilometers, constituting approximately 69% of the overall terrestrial expanse. Numerous urban centers have been meticulously established upon these majestic mountains, with an excess of 600 cities adorning the Chinese landscape, among which over 300 are designated as mountain cities. Remarkably, the populace residing within these elevated realms constitutes approximately 50% of the total national population, thus veritably endowing China with the distinct appellation of a bona fide ‘nation of mountains’.
In the realm of natural circumstances, encompassing topography and climatic influences, the emergence of twisted winds becomes a conspicuous tendency within mountainous regions. The intricate domain of twisted wind unveils a mutable and intricate nature, characterized by pronounced three-dimensional attributes. Both the wind profile and the distribution of wind speed spectra diverge significantly from the conventional wind patterns. Moreover, the phenomenon of gaseous flow, separation, and reattachment encircling towering edifices, subject to the effects of the twisted wind, becomes conspicuously accentuated. This heightened phenomenon serves to render the endeavor of wind resistance design for lofty mountainous skyscrapers an exponentially intricate task. Thus, the imperative pertinence of delving into the realm of aerodynamics pertaining to lofty structures under the sway of twisted wind conditions becomes unequivocally evident.
1.1. The twist of wind
Over the past several decades, scholars have undertaken a multitude of investigations concerning the wind-induced loads imposed upon high rise buildings, employing methodologies including wind tunnel experimentation under typical wind conditions (Kareem, Citation1992; Marukawa et al., Citation1992; Ming, Citation2009; Ming et al., Citation2009; Tanaka, Citation2012). Despite their notable contributions, the aforementioned studies were all conducted under the premise that the direction of the inflow wind remains unchanged with altitude.
The atmospheric boundary layer is commonly stratified into the Surface layer, Ekman layer, and Free atmosphere, as depicted in Figure . Zheng et al. (Zheng et al., Citation2019) asserted that the deviation of wind direction is negligible in the range of 3-100 m, thus rendering the effect of wind deflection insignificant in this interval. Indeed, the wind direction within the entire Surface layer can typically be regarded as constant, which validates the assumption for the lower atmospheric boundary layer (ABL) – the range that pertains to moderate-rise buildings.
However, as high-rise edifices soar into the Ekman layer, it becomes a matter of significance to investigate whether the wind directions remain constant with altitude. Existing mathematical models (Rossby & Montgomery, Citation1935; Taylor, Citation1916) and field-measured data (Mendenhall, Citation1967; Peña et al., Citation2014) demonstrate that due to the combined influence of factors such as earth rotation (i.e. the Coriolis effect), surface friction, and atmospheric pressure gradients, the direction of the natural wind varies considerable with height above 200 m. This variation gives rise to a helical wind profile that can be described by the Ekman spiral (refer to Figure ). In addition to these mesoscale effects, certain local factors, such as topography of mountainous regions (where many modern metropolises are situated), significantly contribute to the formation of twisted wind profiles. The theoretical cumulative angle of twist can be as substantial as 45° (Ekman, Citation1905), which implies that the streamwise and lateral components, based on the most rudimentary trigonometric estimate with cosine 45°, can be diminished and intensified by as much as 30% and 70%, respectively. These twisted wind profiles exert a significant impact on the aerodynamics of high-rise structures, the surrounding flow phenomena, and the wind environment to which residents are subjected.
1.2. Research on twisted wind profile
Tremendous efforts have been dedicated by scholars towards analyzing twisted wind profiles. A pioneering effort was made by Flay and colleagues from the University of Auckland (Flay, Citation1996), who conducted a series of wind tunnel tests on a yacht’s sails under twisted wind conditions. Additionally, they introduced a methodology for simulating twisted winds in the wind tunnel by using guiding vanes. Subsequently, Hedges et al. (Citation1996) conducted a series of numerical simulations to examine the twisted effects on the dynamics of a yacht’s sails. Their findings emphasized the significance of including the twisted effect in wind tunnel experiments, especially when there is an appreciable vertical variation of mean wind direction.
Despite having insights into sail aerodynamics, it was only recently that a systematic study on the twisted wind field was conducted by Tse, Weerasuriya and colleagues (Tse et al., Citation2016, Citation2017a, Citation2017b; Weerasuriya et al., Citation2018; Weerasuriya et al., Citation2018; Zhang et al., Citationn.d.) through a series of wind tunnel tests. Among the 256 wind profiles that were tested in 18 actual topographic sites in Hong Kong, a mountainous metropolis, more than 40% of the measured wind profiles exhibited twisted effects. If we define the maximum angle of twist as the discrepancy between the mean wind directions measured at the ground and at measurement height, 40% of all cases exhibited an angle of twist of at least 8°, 20% of at least 15°, and a maximum of 35° – thus, the topography-driven twisted wind is a common and significant concern for building design. Subsequently, Tse et al. conducted a series of wind tunnel tests to study the pedestrian-level wind environment around an isolated building and building arrays under twisted winds. The results showed that the pedestrian-level wind environment changed significantly with twisted winds. While they have conducted extensive research on the impact of twisted wind on the pedestrian-level wind environment, it is important to note that their studies did not encompass the investigation of aerodynamic loads on high-rise buildings under twisted wind conditions.
1.3. Research on building aerodynamics subjected to twisted winds
Building upon previous studies, a natural progression is to evaluate the aerodynamic loads impose upon high-rise structures when subjected to twisted winds. Certain researchers adopted strategies to simplify the simulation of such winds. For instance, Yeo (Citation2012) assumed that the angle of twist remained uniform throughout the height of the building, rather than gradually increasing. This simplification is equivalent to altering the building’s orientation, causing the building to spiral by the same angle as the twisted wind. Inaccuracies are inherently embedded in this method. Zhang (Zhang, Citation2013), on the other hand, employed a different approach by dividing the building into segments based on height and assumed constant twisted angles for each segment, with varying angles across segments. As expected, the accuracy of this discretization-like approach is determined by the resolution and total number of segments. These simplification strategies constitute a commendable initial endeavor to investigate the impact of twisted wind on the wind-induced response of high-rise structures, and possesses the potential to instigate subsequent pertinent research endeavors. Nevertheless, its scope is constrained by its inability to engender authentic twisted wind patterns.
In recent years, while there has been some research conducted on the aerodynamic characteristics of high-rise buildings under real twisted wind conditions, it is true that there is still a lack of comprehensive studies in this area. Twisted wind profiles with twisted angles of 15° and 25° were replicated by Liu et al.(Liu et al., Citation2019) through the utilization of guide vanes system. These profiles were employed to investigate the surface wind pressure and aerodynamic effects on high-rise structures featuring square sections when subjected to the influence of said twisted wind profiles. Yan et al.(Yan et al., Citation2022) conducted wind tunnel tests to investigate the impact of twisted wind profile with twisted angles of 20° and 30° on typical super high-rise structures (square columns with a height of 500 m). The study delved into intricate aspects such as the distribution of extreme pressure, as well as the interplay and correlation among local wind loads. Remarkably, the findings revealed a profound influence of twisted wind on the mechanism governing the dynamic response of the structures. Despite the aforementioned research's comprehensive exploration of the impact of twisted wind on high-rise buildings via wind tunnel tests, it falls short in terms of numerical simulation studies, which would allow for observing the alterations in the flow field surrounding high-rise structures under the influence of twisted wind. Yuan et al. (Yuan et al., Citation2022) simulated twisted wind inflow based on Reynolds-averaged Navier-Stokes (RANS) and predicted the aerodynamic force acting on square high-rise buildings. While RANS simulations can provide flow field information around high-rise buildings, its simulation accuracy is relatively lower when compared to Large Eddy Simulation (LES). Thus, there is a need for further research to bridge this gap and provide a more thorough understanding of the aerodynamic behavior of high-rise buildings subjected to realistic twisted wind conditions.
To this end, in this study, wind tunnel experiments utilizing guide vanes and passive simulation devices are conducted to replicate the twisted wind field and compared against conventional winds. The aerodynamic characteristics of an isolated high-rise building subjected to twisted winds are then examined. Finally, LES is employed to simulate twisted winds in high-fidelity numerical simulations, allowing for an analysis of the fluid phenomenology around the building through the lens of fluid mechanics. The results of this study are expected to provide an informative reference for the design of high-rise buildings, particularly in mountainous regions, and future investigations utilizing wind tunnel and CFD techniques for analyzing twisted winds.
2. Methodology
2.1. The twisted wind fields
It is necessary to consider the twisted effect of wind direction on high-rise structures. According to the Ekman spiral theory, the angle of twist can be defined as.
(1)
(1) where v denotes the lateral velocity, u denotes the streamwise velocity, and Vgr denotes the resultant velocity of the incoming flow.
In consideration of previous research (Tse et al., Citation2016), which revealed that the maximum angle of twist in the ABL below 500 m is 35°, this study employs angles of 15° and 30° to represent winds with moderate and extreme twisting, respectively, in the ensuing investigation. In comparison, the twisted winds are evaluated against the conventional benchmark (i.e. an angle of twist of 0°) in accordance with the type B landform of the ‘Load code for the design of building structures’ (GB50009, Citation2012). It is worth noting that type B landform encompasses areas such as fields, villages, jungles, hills, and towns with a low density of houses, and the power exponent of the wind profile is α = 0.15. For ease of reference, the three wind conditions are denoted as the conventional wind field (CWF), twisted wind field 15° (TWF15), and twisted wind field 30° (TWF30) in all upcoming discussions.
2.2. Wind tunnel experiment
2.2.1. Facility and apparatus
The DC Wind Tunnel Laboratory of Chongqing University, Chongqing, China (refer to Figure ), served as the location for all wind tunnel assessments. The dimensions of the wind tunnel extend to a total length of 37.69 m, a maximum width of 4.4 m, and the maximum height of 3.8 m. The test section measures 15 m in length, 2.4 m in width, and 1.8 m in height, respectively. The two side walls of the test section feature a 0.4° diffused angle aimed to diminish the axial static pressure gradient. The diameter of the turntable reaches 2 m, while the minimum operating wind velocity measures at 0.5 m/s, and the maximum operating wind velocity registers at 35 m/s.
The TFI Cobra three-dimensional pulsating anemometers were utilized for the measurement of the simulated ABL. These anemometers consist of a cobra probe, a 4-hole pressure probe, that concurrently measures three-dimensional wind velocities and static pressures. The data acquisition duration extends to 60 s, with a sampling frequency of 500 Hz. As for the wind pressures recorded during the rigid pressure test, they were gauged utilizing PSI (ESP-64) miniature electronic pressure scanning valves and a DCT Initium network electronic pressure scanner. Each scanning valve has the capacity to collect data from up to 64 measuring points simultaneously. The data acquisition duration for this test was set at 45 s, with a sampling frequency of 330 Hz. The data acquisition length for each measuring point is 15000, with a wind speed ratio of 1/5 and a time ratio of 1/80.
Throughout the experiment, a three-displacement side frame and an electronic control system were utilized to stabilize the cobra probe, thereby positioning the sampling node at the center of the turntable for the collection of wind velocity data at varying heights. The sampled elevations were taken at heights measuring 15, 30 mm, 60-300 mm (at 30 mm intervals), 350-600 mm (at 50 mm intervals), and 700-900 mm (at 100 mm intervals), amounting to a total of 20 sampled elevations.
2.2.2. Experimental design and test model
To manipulate the turbulence characteristics of the incoming wind, passive simulation devices were employed during the wind tunnel tests. However, conventional devices such as wedges, rough elements, and carpets lacked the capability to alter the direction of the incoming wind. As a result, guiding vanes were fabricated and implemented to generate the intended angles of twist (refer to Figure ). The vanes, which possess a height of 900 mm and are constructed using colloid-bonded, stacked 30mm ×1000 mm wooden blocks, were preferred over traditional smooth-surface prototypes because they provide a more accurate simulation of uneven topographies present in real-world settings. In strict adherence to the Ekman spiral model, two vanes were produced featuring twists of 15° and 30°, respectively. Additionally, a straight transition zone measuring 400 mm was incorporated atop the vanes to prevent the occurrence of phenomena such as free-surface separation and associated vortex streets.
It is important to note that an excessive degree of twisting may result in undesired reflection of the twisted flow off the wind tunnel wall. Given that wind tunnels cannot be infinitely wide, our preliminary test constrained the maximum angle of twist to roughly 30° for the present test section. Furthermore, due to limitations of test section, turning vanes positioned too close to the inlet may hinder the complete development of turbulence. Consequently, after preliminary testing and calibration, the guiding vanes were located 0.7 m downstream of the inlet for the current experiment. These vanes were mounted onto wind tunnel walls by a rigid, slidable frame and anchored in place by bolts to ensure their stability and safety. The vanes were strategically positioned such that the twisted wind flow was directed straight onto the turntable. Careful attention was paid to the boundary conditions to avoid vibrations in the guiding blades and ensure that the simulated inflow was accurate. Figure depicts the wind tunnel floor plan and apparatus setup.
Figure 5. (a) A schematic diagram of the wind tunnel layout (unit: m); (b) Photo footage of the apparatus setup.

In this study, a cantilevered prism with an aspect ratio of 6.25:1 was chosen as the isolated high-rise building. The geometric similarity principle was applied to scale the model down with a ratio of 1:400, resulting in a model size of 96mm×96mm×600 mm (length×width×height). The wind tunnel blockage ratio was maintained at a low 1.33%, which is well below conventional standards. In order to measure the pressure distribution, nodes were placed on all four walls of the model, which was divided into seven layers based on its height. A total of 112 nodes were placed equidistantly on each layer, resulting in an overall coverage of 16 nodes per layer (refer to Figure ).
2.3. Numerical simulation
In addition to the wind tunnel experiment, this study also carried out Computational Fluid Dynamics (CFD) simulations to analyze the altered fluid morphology around an isolated high-rise building. These simulations provide an economical and accurate alternative to the prohibitively expensive planar Particle Image Velocimetry (PIV) measurements and allow for three-dimensionally omniscient flow field visualization.
2.3.1. Computational domain and grid
A hexahedral volume of 8m×6m×3 m (length×width×height) comprises the computational domain. The building, situated 5H downstream from the inlet, 8.3H upstream from the outlet, 4.92H away from the lateral sides, and 4H beneath the domain roof, is of height H, as demonstrated in Figure a. The aforementioned dimensions lead to a blockage ratio of 0.32%, which is approximately tenfold lower than the standards recommended by the AIJ guideline (Tamura et al., Citation2008).
The computational domain was discretized by the ANSYS ICEM 19.0 package, and the numerical model is 1:1 with the wind tunnel model. The grid meshing was done using a hybrid grid scheme, as shown in Figure b. A refinement region in the immediate vicinity of the structure adopted tetrahedral and pentahedral cells. The exterior blocks surrounding the refinement region adopted hexahedral cells to enhance computational accuracy. The interfaces between the structured and non-structured cells were set to interior by node merging for efficient data transmission. The growth rate is restricted to under 1.05. 20 layers were used to resolve the near-wall viscous sublayer with a first-layer thickness of 4 × 10−5 m and a maximum thickness of 9.6 mm, yielding y+<1. The final grid contains about 6 million cells.
2.3.2. Boundary conditions and the twisted inflow
Establishing appropriate boundary conditions (BCs) is of utmost importance in CFD numerical simulation. For CWF, the boundary condition at the computational domain’s inlet was designated as velocity-inlet, with the inlet flow reproducing the u and turbulence intensity profiles as measured in the wind tunnel test. The outlet condition was established as outflow. All remaining four faces of the computational domain and model walls were defined as wall to replicate the conditions observed in the wind tunnel experiments. Regarding TWF, the inlet and lateral faces of the computational domain were established as velocity-inlet to generate the twisted effect. The expressions were derived from the streamwise velocity u, the crosswind velocity v, and the turbulence intensity, which were obtained from the wind tunnel experiments. All other BCs were identical to those of CWF.
2.3.3. Solution method
CFD numerical simulation was performed by the FLUENT 19.0 software, and the Large Eddy Simulations with Near-Wall Resolution (LES-NWR) was selected as the numerical scheme. For concision, the solution methods and settings have been summarized in Table .
Table 1. LES solution condition setting.
3. Validation
3.1. Grid resolution
This section is dedicated to the verification of grid independence in CFD simulations. The LESIQ index was adopted to quantify the resolution of the LES grid. It is an advanced, authoritative method to validate LES, proving the complete resolution of the energy-containing range. For this reason, RANS-based grid convergence can be spared. Celik et al.(Celik et al., Citation2005) proposed the LESIQ index as:
(2)
(2) where,
and
are subgrid and molecular kinematic viscosities, respectively. LESIQ > 75% is an indicator of sufficient resolution, signaling that the subgrid filter sits in the inertial subrange and all the energy-carrying (i.e. large) eddies have been resolved by the Navier-Stokes functions (true LES-NWR). Figure showcases the LESIQ on the Z = 0.5H plane. The outcomes conclusively demonstrate that the current grid attains LESIQ values exceeding 82% across all relevant fluid regions. This comprehensive evaluation of LESIQ substantiates the superior quality and resolution of the employed LES grid.
In order to concentrate on the time-averaged flow field, it is imperative to commence sampling after the flow attains statistical steadiness. To fulfill this requirement, global statistics were employed as a criterion to determine the steady-state condition. As shown in Figure , despite the vast instabilities observed in the early, the RMS, and the mean lift reached statistical stationarity after t* = 2.125×102 (t* = tUref/D is dimensionless time). Hence, t* = 2.125×102 was selected as the initial sampling time.
3.2. Wind tunnel-replicated wind fields
This section validates the wind fields generated by the wind tunnel experiments. All discussions in this section solely focus on the wind field in the absence of the structure model.
Figure a displays the wind velocity measurements taken at five distinct locations, designated as A, B, C, D, and E. Point C is set as the reference point, with points A and B located 50 and 25 cm to the left (indicated by a negative sign) of point C, while points D and E serve as counterparts to the right (indicated by a positive sign). The normalized mean profile at points A to E is presented in Figure . The reference height Zr remains consistent with the structural height (H), at a value of 600 mm.
Figure 10. Comparison of mean wind profiles at different locations under different wind fields: (a) CWF (b) TWF15; (c) TWF30.

Observing Figure a, it is apparent that the wind profiles for CWF adhere to the Type B wind profile defined in GB50009-2012. This concurrence confirms the authenticity of the wind tunnel experiment, and more significantly, emphasizes the insubstantial three-dimensional influence of non-twisted, conventional wind fields, as demonstrated by the curve’ nearly perfect overlap.
Conversely, the mean wind profile undergoes significant modifications under the influence of TWFs. An inflexion point emerges around z/Zr = 1.0, above which the most significant deviations from the Type-B profile manifest at point E for TWF15, and at point B and E for TWF30. These deviations also account for an overarchingly accelerating effect on the mean wind profile. Below the inflexion point, the deviational patterns of TWF15 and TWF30 differ substantially. In the case of TWF15, wind decelerates at point C, accelerates at points D and E, and exhibits a secondary inflection at points B and A, where wind accelerates above z/Zr = 0.3 and decelerates below. This observation contrast sharply with the wind profile of TWF30, which is mainly decelerative below the primary inflection at z/Zr = 1.0, perhaps with the only exception near the floor. This may be caused by the size limitation of the wind tunnel and the large angle of twist, which makes the twisted wind collide with the side wall of the wind tunnel.
The twisted effect of TWF relative to CWF is evident. To minimize the impact caused by the wind tunnel’s size constrains and replicate the actual twisted wind profile as accurately as possible, the data at the center of the turntable (point C) were chosen for further analysis.
Figure provides a comparison of the normalized results of the CWF with the corresponding outcomes of the Type-B landform in the GB50009-2012 specification. It is deduced that the trends of variation, specifically the exponential profiles of streamwise velocity and turbulence intensity, are qualitatively consistent with the theoretical specifications. In terms of quantity, the mean disparities between the empirical and theoretical curves amount to 3.91% and 14.55% for velocity and turbulence intensity, respectively, falling comfortably within the acceptable deviation range. It is worth noting that the three points in close proximity to the ground (with heights of 15, 30 mm, and 60 mm) of the velocity exhibit disparities from the empirical values, possibly attributable to the influence of coarse constituents. The wind tunnel laboratory, being confined by experimental constrains, features rough elements of no less than a cube with a side length of 50 mm. Considering the limitations of the experimental conditions, the wind profile depicted in the illustration represents the most optimal outcome following numerous adjustments. With the exception of a discrepancy of approximately 10% at the lowermost three points, the remaining deviations remain below 5%. Consequently, the replicated CWF is deemed viable to be comply with the specification requirements and can be employed as the benchmark for subsequent wind field analysis.
Figure 11. Comparison of the streamwise velocity and turbulence intensity profiles between the CWF and theoretical curve of GB50009-2012.

The normalized profiles of TWF and CWF, as obtained from wind tunnel tests, are compared in Figure . It is evident that while the wind velocity profiles of TWF15 and TWF30 generally follow the trend of CWF, significant deviations can be observed near the ground and above z* = z/600 = 1.0 for TWF15, and between z* = 0.2-1.0 for TWF30, indicating a clear twisted effect. In the case of TWF15, the mean deviation from CWF is 6.71%, occurring near the ground at z* = 0.15, with the deviation above z* = 1.0 also being non-negligible at 3.39%. Thus, z* = 1.0 acts as an inflection point of a convex curve. In contrast, for TWF30, the mean deviation from CWF is 5.57%, occurring mid-height at z* = 0.6. There are two inflection points at z* = 0.2 and 1.0, where the overall convex curve is divided by a concave segment in between. It is evident that the angle of twist has a significant impact on the wind profile, as expected.
Figure 12. Comparison of the streamwise velocity and turbulence intensity profiles between (a) TWF15 and (b) TWF30 and the corresponding CWF.

Interestingly, despite the significant impact of twisting on the velocity profile, its influence on turbulence intensity is found to be negligible. The turbulence profiles of both TWF cases coincide with that of CWF, with mean deviations of 7.90% and 4.11%, indicating near-perfect matching. The wind tunnel results demonstrate that while twisting changes the direction of wind flow and varies the streamwise speed, it has little effect on promoting turbulence content. This highlights the difference between twists of fluid elements and eddies of turbulence. Further discussions on the twisted wind field will be conducted based on full-field CFD measurements.
3.3. CFD-simulated wind fields
The flow field's comprehensive information, which is a crucial supplement to subsequent analysis, is provided by CFD simulations in addition to wind tunnel measurements. In this section, the validation of CFD-simulated twisted winds against the wind tunnel benchmark is presented, and comparisons based on TWF15 are chosen as the representative case for concision. First, Figure illustrates the normalized profiles’ comparison between the wind tunnel test and CFD simulations. As anticipated, the turbulence profile consistently follows the trend, exhibiting minimal differences between wind tunnel test and CFD (called WT-CFD). The mean deviation is limited to 9.90%. The velocity profiles perfected overlap between z* = 0.6-1.5. while some variations are observed below z* = 0.6, even though the single-inflection, concave curvature is well maintained. The mean deviation is 5.97%.
Figure 13. Comparison of the streamwise velocity and turbulence intensity profiles between wind tunnel test and CFD simulation for TWF15.

This phenomenon may arise from the reflections resulting from the unavoidable interaction between the incoming flow and the wind tunnel wall during testing, despite efforts made to mitigate this issue. Conversely, such a predicament does not manifest itself in the CFD simulation of the TWF. Fortunately, the maximum disparity between the test results and CFD findings remains below 12%, falling within the realm of acceptability. Henceforth, it is deemed appropriate to employ the CFD simulation results for subsequent analysis.
The LES-NWR was successful in simulating the twisted effect with high accuracy. Figure presents a comparison of the yaw angle, or the angle of twist, between the wind tunnel test and CFD simulations. It is evident that the agreement is excellent below z* = 1.0, where the two curves exhibit a remarkable overlap. Quantitatively, the mean deviation is limited to a mere 7.43%. The only notable difference between WT-CFD is observed above z* = 1.0, and it is due to the upright shielding board installed above the guiding vanes. The CFD case was unable to model the sudden change of slope. Nevertheless, this difference will not affect the analysis of building aerodynamics because the portion is well above the building height. The comparison of lateral velocity v further supports the agreement between WT-CFD (see Figure ). The qualitative agreement is self-evident below z* = 1.0, where the mean quantitative deviation is only 5.41%. Overall, the CFD simulation accurately describes the twisted wind fields generated in the wind tunnel.
The argument is further reinforced by Figure , which demonstrates consistent patterns in the pressure coefficient induced by the twist-wind on the structural model. Although the CFD case slightly overestimates the pressure on all four walls, the pressure patterns clearly depict the forced, sharp-edge fluid separation on the windward wall, the formation of turbulence-transition-bypassed separation zones on the crosswind walls, and the pressure restoration on the leeward wall. Most notably, Figure emphasizes the asymmetric pressures of the crosswind walls, with one exhibiting a significant decrease compared to the other. This observation will be further explored in Section 4. In general, the resemblance between the simulated and the experimental wind fields establishes a study foundation for the subsequent analysis of building aerodynamics.
4. The twisted wind field and building aerodynamics
The experimental and numerical results are analyzed in this section, with detailed discussions presented on the flow field characteristics of twisted wind. This is followed by a discussion of the rigid manometric test and CFD simulations of a high-rise building with a square section and an aspect ratio of 6.25:1, with a focus on the influence of twisted winds on a structure’s mean surface pressure, force spectrum, and lift/drag coefficients.
4.1. Characteristics of TWF without building
This section primarily elucidates the wind field attributes pertaining to TWF, encompassing turbulence intensity, crosswind velocity, twisted angle profile, and fluctuating spectrum. This serves to foster a profound comprehension of TWF.
Figure presents a comparative analysis between the turbulence observed during testing and the theoretical turbulence at point C, across three distinct wind fields. As depicted in the illustration, the turbulence for both CWF and TWF30 aligns harmoniously with the theoretical turbulence. However, it is noteworthy that a slight disparity exists within the lower portion of the TWF15 turbulence, in relation to the theoretical turbulence. Nonetheless, the maximum deviation remains under 5%, thereby falling within the permissible margin of error.
Given the negligible magnitude of the crosswind component in the CWF, it can be disregarded. Additionally, the CWF does not account for the variation of the twisted angle with height. Therefore, Figure and Figure solely depict the comparative findings pertaining to the crosswind speed and the variation of the twisted angle profile along the vertical axis within the TWFs. The figure reveals that within the TWF scenario, both the crosswind wind speed and twisted angle exhibit a consistent pattern in relation to the variation in height. Specifically, they gradually diminish as the height increases. The highest values for the crosswind speed and twisted angle are observed in proximity to the ground. Moreover, as the twisted angle within the TWF increases from 15° to 30°, the alterations in both the crosswind speed and twisted angle profile become more pronounced with respect to changes in height. Additionally, Figure also presents a comparison between the outcomes of the twisted angle profile and the Ekman spiral. It is discernible from the illustration that the fluctuation pattern of the twisted angle profile harmonizes with that of the Ekman spiral. With the exception of a specific divergence in the outcomes near the base of the edifice, the remaining portions situated beneath the edifice's altitude evince substantial consonance. This occurrence may stem from the interplay between the inflow and the wind tunnel wall, which exerts a discernible influence on the results. Regarding the deviation above the height of the building, it is attributable to the erect shielding panel affixed atop the guide vanes. Nevertheless, the incongruity in this segment, surpassing the edifice's stature manifold, does not cast a shadow upon the aerodynamic scrutiny of the structure.
The maximum twisted angles recorded during the test for TWF15 and TWF30 are 13° and 21°, respectively. These values exhibit a slight deviation from the corresponding theoretical values. This variance can potentially be attributed to the considerable distance between the incoming wind and the measurement point, resulting in some loss as the wind traverse passive simulation devices such as wedges and rough elements before reaching the measurement point. Furthermore, it is worth noting that as the twisted angle increases, the level of loss also experiences an escalation.
Figure exhibits the comparative outcomes between the fluctuating wind speed spectrum and the Von Karman spectrum at various wind fields, specifically at the height of Zr. The illustration reveals a substantial resemblance between the fluctuating spectra generated during the test for both CWF and TWF, and the esteemed Von Karman spectrum. Furthermore, it is worth noting that the twisted effect induced by the wind direction twist does not exert a notable impact on the downwind fluctuating spectrum. In contrast to the varying spectrum observed in the downwind direction, the crest of the crosswind fluctuation spectrum experiences a subtle displacement towards higher frequencies due to the influence of twisted effects, particularly evident in the case of TWF30. The migration of the zenith of the crosswind fluctuation spectrum can be attributed to the alteration of the flow field induced by the anterior positioning of vane systems preceding the turntable installation.
4.2. Building aerodynamics
4.2.1. Time-average pressure distribution
To analyze the data effectively, the dimensionless pressure coefficient is used to quantify the building’s surface pressure. The pressure coefficient at time t at the measuring point i on the model surface is derived by the formula (3):
(3)
(3) where
is the pressure coefficient at measurement node i at time instant t;
is the wind pressure at measurement point i at time t;
and
are the total pressure and static pressure at the reference height, respectively, and the reference height in this paper is taken as the height of the structure (H).
In Figure , a comparison is made between the time-averaged pressure coefficients of tall buildings that are isolated, under conditions of CWF and TWF. It can be observed from the figure that under CWF, the windward side of the structure experiences positive pressure. The pressure exhibits a symmetric distribution that is bounded by the center line of the structure, and gradually increases with the height of structure. The maximum pressure is attained at approximately 0.8H, which indicates the stagnation location. Subsequently, the pressure decreases further up the structure due to end effects, such as free-surface separation. In contrast to the windward wall, the pressure on the leeward and crosswind walls is negative, indicating the expected suction. The magnitude of suction also increases with height, with an overall symmetry. The maximum suction is observed near the free-end due to the draw-in from recirculation. Lastly, the symmetry of the crosswind walls serves as evidence for the accuracy of the wind tunnel experiment. The suction observed therein is stronger than that of the leeward wall, reaching the maximum at approximately 0.9H. The appearance of sharp pressure gradients, commonly referred to as pressure bands, highlights the symmetric occurrence of reattachment (impingement of leading vortex) that is frequently reported by studies on vertical prisms (Chen et al., Citation2020, Citation2021, Citation2023).
Figure 21. Comparison of average wind pressure coefficient distribution of isolated tall buildings under CWF and TWF.

Significant differences in pressure are observed under the TWFs as compared to the CWF benchmark, with the most remarkable being the loss of symmetry. Looking at TWF15 first (refer to Figure b), pressure on the crosswind walls highlights the effects of twisting: the left wall no longer sees the pressure band, signaling the absence of reattachment; the right wall shows an intensified pressure band that encroaches further up towards the leading-edge for early reattachment. The maximum suction remains unchanged on the left wall, but on the right wall, it slightly increased from −1.15 to −1.20. The leeward wall displays a twisted pressure pattern and witnesses the most significant increase in suction among all walls near the free-end, rising from −0.8 to −0.9. Moreover, the mid-span region characterized by low-suction (−0.65) in the CWF benchmark reduces in size and increases to −0.70. Finally, the windward wall also exhibits a twisted pressure distribution, resulting in a reduction in the size of the stagnation region.
As the angle of twist increased to 30°, the changes described above become more pronounced (refer to Figure c). The stagnation region on the windward wall collapses down to essentially a single point, and the stagnation pressure weakens to 0.70. The leeward wall experiences a total loss of symmetry and significant pressure restorations, with the maximum suction decreasing from 0.90 in TWF15 to 0.65 in TWF30. Furthermore, suction on the left wall also decreases from −1.10 to −0.95, and no sign of reattachment could be observed. In contrast, the right wall experiences increasingly early reattachment, in which the pressure band runs across the entire span of the model, and the suction region (i.e. separation bubble) becoming more constrained towards the leading-edge. The observed pressure distribution patterns corroborate previous findings by Tse et al.(Tse et al., Citation2016), thus reinforcing the accuracy of our current results.
4.2.2. Force spectrum and force coefficient
This section selects Level 1, 4, and 7 (0.09H, 0.51H, and 0.93H) of the building, characterizing the fix-end, mid-span, and free-end, respectively, to analyze the Root-Mean-Square (RMS) lift coefficient and its spectral density. The RMS lift coefficient is calculated according to:
(4)
(4) where
is the RMS lift/drag;
is the density of air;
is the velocity at the top of building; A(z) is the projected area of the windward surface; z is the height.
Figure and Figure compares the lift and drag spectra by Levels (Figure a-c, Figure a-c) and wind field conditions (Figure d-f, Figure d-f). The abscissa is , and the ordinate is
. S denotes the fluctuating spectrum, f is the frequency (Hz),
is the variance of fluctuating wind velocity.
Figure 22. Comparisons of lift spectra of (a) CWF; (b) TWF15; (c) TWF30 at Levels 1, 4, and 7; comparisons of lift spectra of (d) Level 1; (e) Level4; (f) Level 7 under CWF, TWF15, and TWF30.

Figure 23. Comparisons of drag spectra of (a) CWF; (b) TWF15; (c) TWF30 at Levels 1, 4, and 7; comparisons of drag spectra of (d) Level 1; (e) Level4; (f) Level 7 under CWF, TWF15, and TWF30.

The sharp spectral peak observed in Figure a depicts the concentrated and intense occurrence of the Bénard-von Kármán instability and the subsequent vortex shedding, as induced by the CWF. The sharpest peak is noted at Levels 1, followed by Level 4 and 7. With an increase in the angle of twist (0°→15°→30°), the peak of the power spectrum gradually diminishes and the bandwidth gradually shifts from ‘sharp’ to ‘flat’, indicating a stable reattachment of the crosswind flow. Furthermore, it can be observed from the figure that an increase in the angle of twist results in an apparent upward trend in the power spectrum curve of the first layer, while the trend of the power spectrum curve of the fourth and seventh layers remains unchanged. This trend can be attributed to the fact that the effect of twisted wind is more significant at a lower height, with the twisting of wind direction gradually decreasing and eventually flattening as height increases.
Figure d-f portrays the crosswind wind power spectrum curves of a single high-rise building at the same height, under varying wind field conditions, with the aim of studying its crosswind wind characteristics under TWF conditions.
As depicted in Figure d, an increasing of the wind direction angle of twist results in a decrease in power spectrum energy at the height of the first layer, with a significant decrease in peak value, and a rapid shift of the bandwidth from a ‘sharp’ state to a ‘flat’ state. Moreover, an increase in the wind direction angle of twist leads to an apparent asymmetry on the side wind surface of the building, with a weakened energy concentration phenomenon. However, as the building height increases, the wind direction angle of twist continuously decreases along the height. Specifically, on the fourth floor of the building, the phenomenon observed on the first floor gradually disappears. When the height reaches the seventh floor, the transverse wind direction power spectrum curves under different wind field conditions remain unchanged, as illustrated in Figure e and f.
The influence of wind direction torsion on the drag spectrum in the low-frequency range is evident, as depicted in Figure . Figure a-c demonstrates that the variation in height has minimal impact on the drag spectrum under the CWF condition. Nevertheless, an increase in the twisted angle results in a noticeable occurrence where the drag spectrum decreases with height in the low-frequency range. This phenomenon is particularly pronounced, especially in the case of TWF30.
In a similar vein, for a more comprehensive analysis of downwind wind characteristics in a single high-rise building under TWF conditions, Figure d-e illustrates the downwind wind power spectrum curves of buildings at the same height but experiencing different wind field conditions. The figure reveals that, at the ground level of the building, the drag spectrum exhibits a significant increase in the low-frequency range with an augmentation of the twisted angle. However, as the height ascends, the disparity in the drag spectrum at the base of the building starts to diminish, and by the time the structure reaches the seventh floor, the distinction among the drag spectra under the three wind field conditions virtually disappears.
Figure displays the curve of the RMS lift coefficient and drag coefficient along the height under different wind field conditions. It can be observed that the sensitivity of the RMS lift coefficient to the building height and the wind direction angle of twist is significantly greater than that of the RMS drag coefficient. This phenomenon can be attributed to the fact that wind direction torsion has a more substantial impact on the average wind pressure coefficient of the crosswind surface of the structure, with the lift coefficient primarily being affected by the wind pressure coefficient of the left and right sides.
Figure 24. High-rise building sectional RMS force coefficient: (a) RMS lift coefficient; (b) RMS drag coefficient.

Figure a illustrates that the RMS lift coefficient initially increases with the the building height, reaching its the maximum value between 0.6H and 0.7H. However, as the height continues to increase, the RMS lift coefficient gradually decreases. Additionally, Figure a also indicates that an increase in the wind angle of twist results in a decrease in the RMS lift coefficient, with a greater decrease closer to the bottom of the building. In contrast to the RMS lift coefficient, the RMS drag coefficient is less affected by the twisted wind and remains stable in the height range, as presented in Figure b.
4.3. Flow phenomenology
The present section aims to delve deeper into the action mechanism of wind load on a solitary high-rise building under TWF. This is achieved by scrutinizing the flow field of the mean velocity around the edifices based on the results of CFD simulation. Additionally, the Q criterion is employed to scrutinize the vortex structure on the surface of the buildings. Formula (5) delineates the computation of the Q criterion.
(5)
(5) Among them, the matrices A and B represent symmetric and antisymmetric tensors respectively, which can be calculated according to formula (6).
(6)
(6) Simplified to the three-dimensional Cartesian coordinate system, the Q value can finally be expressed as:
(7)
(7) Where, u, v, and w are the velocities in the three directions of x, y, and z.
Figure and Figure illustrate the streamlines of the average velocity downstream and z-direction vorticity diagrams of high-rise buildings at various elevations in the vertical direction under the influence of CWF, TWF15, and TWF30.
Figure 25. Streamline at different heights of building in CWF, TWF15, and TWF30: (a) Z = 0.1 m; (b) Z = 0.3 m; (c) Z = 0.5 m.

Figure 26. Vorticity graph at different heights of building in CWF, TWF15, and TWF30: (a) Z = 0.1 m; (b) Z = 0.3 m; (c) Z = 0.5 m.

As depicted in Figure , it is evident that flow separation transpires at the leading edge of the isolated structure’s two sides due to shear force under CWF conditions. This leads to the formation of a columnar separation vortex. Additionally, large separation vortices arise in the structure’s wake due to the influence of the inverse pressure gradient. This outcome concurs with the findings illustrated in Figure a, wherein the separation zones of the left and right sides and the leeward side establish a considerable negative pressure zone.
Under the TWF condition, the incoming flow’s direction alters gradually at different heights of the building, and the flow separation of the incoming flow varies significantly at various elevations. At the building’s base, the incoming flow exhibits the largest angle of twist, causing the separation of the shear layers and resulting in asymmetry on the structure’s surface. This asymmetric shear layer separation inclines towards the direction of wind torsion and progressively extends downstream, leading to a reduction in the absolute value of negative pressure on the leeward side of the building. Furthermore, these occurrences become more distinct with the increase in the angle of twist.
As depicted in Figure , the influence of CWF results in the symmetric distribution of separated vortices along the height of the building, thereby leading to symmetrical shedding of vortices in the wake region. This phenomenon is in agreement with the outcome of the symmetrical distribution of the average wind pressure coefficients on the left and right sides of the building under the CWF condition. In contrast, under the TWF condition, it was observed that the twisted wind led to the development of a sizable separation vortex on the left side of the building, whereas the separation vortex on the right side was small and proximate to the building surface. Furthermore, fluid reattachment occurred at the rear edge of the right side, which corroborated the analysis findings in Figure . As the angle of twist of the incoming flow increased, the difference in vortex distribution on the side of the building become more pronounced under the TWF30 condition.
In order to conduct a more detailed analysis of the flow field characteristics of individual high-rise buildings under varying wind conditions, Figure presents the surface configuration of the building as well as the morphology of the wake vortex street from different perspectives under the influence of CWF, TWF15, and TWF30.
Figure 27. Q criterion level diagram of the high-rise building (Q = 5e5): (a) CWF; (b) TWF15; (c) TWF30.

Based on Figure , it can be observed that under CWF conditions, the shear layer experiences flow separation at the top of the structure as well as the edges on both sides, leading to the formation of a relatively complete spanwise vortex and axial vortex, as illustrated in Figure a. These vortices then separate and shed on either side of the structure. Conversely, under the TWF condition, the shear layer also separates on both sides in a similar manner, but as the incoming wind deflects from bottom to top, the vortices gradually break up into small vortex streets in the wake region. This results in a similar vorticity plane outcome, whereby the eddy tends to deflect in the direction of the current flow within the building height. This trend becomes increasingly prominent with the rise in the angle of twist, as shown in Figure b and c.
5. Conclusion
The present study entails the simulation of conventional wind field (CWF), 15° twisted wind field (TWF15), and 30° twisted wind field (TWF30) under standard landform conditions. This was achieved through the use of wind tunnel test and CFD numerical analysis. A comparison of the results from both methods was subsequently conducted. The disparities in wind profile, turbulence, and wind pressure coefficient between TWF and CWF were examined. Lastly, the aerodynamic characteristics of single high-rise buildings were explored based on the simulated TWF. Additionally, the flow field distribution around single high-rise buildings was analyzed utilizing the results obtained from CFD simulated.
The phenomenon of twisted winds exerts a significant influence on the distribution of wind pressure across the surfaces of buildings. When subjected to twisted effects, the asymmetry in the distribution of the average wind pressure coefficient between the windward and leeward sides becomes notably pronounced. The magnitude of the negative value of the average wind pressure coefficient on both the side and leeward aspects diminishes. With an escalation in the degree of twist, the lateral facet may simultaneously encounter diverse segments of positive and negative average wind pressure. Furthermore, a reattachment of the shear layer was observed on the lower right segment of the isolated high-rise building, engendering a substantial recovery in the average pressure coefficient – resulting in a diminished negative value – thus attenuating the wind's suction effect on the lateral face of the building.
The twisted wind has a substantial impact on the cross-wind fluctuating lift spectrum and RMS lift coefficient of single high-rise buildings. Due to the distortion induced in the flow, the occurrence of the vortex shedding phenomenon is mitigated. Consequently, there is a gradual reduction in both the peak magnitude and the energy content of the power spectrum, correlating with the augmentation of the twisted angle. It is important to note, however, that this influence wanes in significance as the height increases. In addition, the RMS lift coefficient of the isolated high-rise building decreases significantly under TWF conditions with an increase in the angle of twist. This disparity is most obvious at the bottom of the structure. In contrast, the RMS drag coefficient is hardly influenced by the twisted wind and remains stable within the height range.
The twisted wind has a significant impact on the flow field and vortex structure of single high-rise buildings. As the angle of twist increases, the wake region extends downstream and trends towards the wind-twisting direction. The twisted wind causes asymmetrical separation bubbles to form on the left and right sides of the building. With an increase in the angle of twist, the flow attachment on the right side moves backward, while the flow attachment on the left side gradually diminishes. Moreover, because of the evident divergence in twisted angles across various elevations, the extensive vortex formation encircling the uppermost section of the building is adeptly fragmented into diminutive vortices. This phenomenon progresses in an inclined and contorted manner.
Acknowledgments
The authors acknowledge the teachers and students of the Wind tunnel laboratory for their guidance and help in the experiments of this paper, and especially thank Ms. Fu Xianzhi for her contribution to the design and implementation of the wind tunnel test scheme.
Disclosure statement
No potential conflict of interest was reported by the author(s).
Additional information
Funding
References
- Celik, I. B., Cehreli, Z. N., & Yavuz, I. (2005). Index of resolution quality for large eddy simulations. Journal of Fluids Engineering, 127(5), 949–958. https://doi.org/10.1115/1.1990201
- Chen, Z., Fu, X., Xu, Y., Li, C. Y., Kim, B., & Tse, K. T. (2021). A perspective on the aerodynamics and aeroelasticity of tapering: Partial reattachment. Journal of Wind Engineering and Industrial Aerodynamics, 212, 104590. https://doi.org/10.1016/j.jweia.2021.104590
- Chen, Z., Huang, H., Tse, K. T., Xu, Y., & Li, C. Y. (2020). Characteristics of unsteady aerodynamic forces on an aeroelastic prism: A comparative study. Journal of Wind Engineering and Industrial Aerodynamics, 205, 104325. https://doi.org/10.1016/j.jweia.2020.104325
- Chen, Z., Zhang, L., Li, K., Xue, X., Zhang, X., Kim, B., & Li, C. Y. (2023). Machine-learning prediction of aerodynamic damping for buildings and structures undergoing flow-induced vibrations. Journal of Building Engineering, 63, 105374. https://doi.org/10.1016/j.jobe.2022.105374
- Ekman, V. W. (1905). On the influence of the earth’s rotation on ocean-currents. Ark.mat.Astron.fys. https://www.researchgate.net/publication/46766779.
- Flay, R. G. (1996). A twisted flow wind tunnel for testing yacht sails. Journal of Wind Engineering and Industrial Aerodynamics, 63(1-3), 171–182. https://doi.org/10.1016/S0167-6105(96)00080-3
- GB50009-2012. (2012). Load code for the design of building structures. China Architecture & Building press. https://www.mayiwenku.com/p-25113114.html.
- Hedges, K. L., Richards, P. J., & Mallinson, G. D. (1996). Computer modelling of downwind sails. Journal of Wind Engineering and Industrial Aerodynamics, 63(1-3), 95–110. https://doi.org/10.1016/S0167-6105(96)00071-2
- Kareem, A. (1992). Dynamic response of high-rise buildings to stochastic wind loads. Journal of Wind Engineering and Industrial Aerodynamics, 42(1-3), 1101–1112. https://doi.org/10.1016/0167-6105(92)90117-S
- Liu, Z., Zheng, C., Wu, Y., Flay, R. G. J., & Zhang, K. (2019). Investigation on the effects of twisted wind flow on the wind loads on a square section megatall building. Journal of Wind Engineering and Industrial Aerodynamics, 191, 127–142. https://doi.org/10.1016/j.jweia.2019.06.003
- Marukawa, H., Ohkuma, T., & Momomura, Y. (1992). Across-wind and torsional acceleration of prismatic high rise buildings. Journal of Wind Engineering and Industrial Aerodynamics, 42(1-3), 1139–1150. https://doi.org/10.1016/0167-6105(92)90121-P
- Mendenhall, B. R. (1967). Statistical study of frictional wind veering in the planetary boundary layer. Colorado State University, Libraries. https://hdl.handle.net/10217/234956.
- Ming, G. (2009). Study on wind loads and responses of tall buildings and structures. Proceedings of the seventh Asia pacific conference on wind engineering, Taipei, Taiwan. https://iawe.org/Proceedings/7APCWE/IS3.pdf.
- Ming, G., Yi, T., & Yong, Q. (2009). Basic characteristics of torsional fluctuating wind force on rectangular super-tall buildings. Journal of Building Structures, 30(5), 191–197. https://doi.org/10.14006/j.jzjgxb.2009.05.019
- Ng, C. F. (2017). Living and working in tall buildings: Satisfaction and perceived benefits and concerns of occupants. Frontiers in Built Environment, 3, 70. https://doi.org/10.3389/fbuil.2017.00070
- Peña, A., Gryning, S.-E., & Floors, R. (2014). The turning of the wind in the atmospheric boundary layer. Journal of Physics: Conference Series, 524, 0012118. https://doi.org/10.1088/1742-6596/524/1/012118
- Rossby, C.-G., & Montgomery, R. B. (1935). The layer of frictional influence in wind and ocean currents. Massachusetts Institute of Technology and Woods Hole Oceanographic Institution, 3, https://doi.org/10.1575/1912/1157
- Tamura, T., Nozawa, K., & Kondo, K. (2008). AIJ guide for numerical prediction of wind loads on buildings. Journal of Wind Engineering and Industrial Aerodynamics, 96(10-11), 1974–1984. https://doi.org/10.1016/j.jweia.2008.02.020
- Tanaka, H. (2012). Experimental investigation of aerodynamic forces and wind pressures acting on tall buildings with various unconventional configurations. Journal of Wind Engineering and Industrial Aerodynamics, 107-108, 179–191. https://doi.org/10.1016/j.jweia.2012.04.014
- Taylor, G. I. (1916). Skin friction of the wind on the earth’s surface. Proceedings of the Royal Society of London. Series A, Containing Papers of a Mathematical and Physical Character, 92(637), 196–199. https://doi.org/10.1098/rspa.1916.0005
- Tse, K. T., Weerasuriya, A. U., & Kwok, K. C. S. (2016). Simulation of twisted wind flows in a boundary layer wind tunnel for pedestrian-level wind tunnel tests. Journal of Wind Engineering and Industrial Aerodynamics, 159, 99–109. https://doi.org/10.1016/j.jweia.2016.10.010
- Tse, K. T., Weerasuriya, A. U., Zhang, X., Li, S., & Kwok, K. C. S. (2017a). Pedestrian-level wind environment around isolated buildings under the influence of twisted wind flows. Journal of Wind Engineering and Industrial Aerodynamics, 162, 12–23. https://doi.org/10.1016/j.jweia.2017.01.002
- Tse, K. T., Weerasuriya, A. U., Zhang, X., Li, S. W., & Kwok, K. C. S. (2017b). Effects of twisted wind flows on wind conditions in passages between buildings. Journal of Wind Engineering and Industrial Aerodynamics, 167, 87–100. https://doi.org/10.1016/j.jweia.2017.04.011
- Weerasuriya, A. U., Hu, Z. Z., Zhang, X. L., Tse, K. T., Li, S., & Chan, P. W. (2018a). New inflow boundary conditions for modeling twisted wind profiles in CFD simulation for evaluating the pedestrian-level wind field near an isolated building. Building and Environment, 132, 303–318. https://doi.org/10.1016/j.buildenv.2018.01.047
- Weerasuriya, A. U., Tse, K. T., Zhang, X., & Li, S. W. (2018b). A wind tunnel study of effects of twisted wind flows on the pedestrian-level wind field in an urban environment. Building and Environment, 128, 225–235. https://doi.org/10.1016/j.buildenv.2017.11.041
- Yan, B., Li, Y., Li, X., Zhou, X., Wei, M., Yang, Q., & Zhou, X. (2022). Wind tunnel investigation of twisted wind effect on a typical super-tall building. Buildings, 12(12), 2260. https://doi.org/10.3390/buildings12122260
- Yeo, D. (2012). Practical estimation of veering effects on high-rise structures: A database-assisted design approach. Wind and Structures An International Journal, 15(5), 355–367. https://doi.org/10.12989/was.2012.15.5.355
- Yuan, Y., Yan, B., Zhou, X., Yang, Q., Huang, G., He, Y., & Yan, J. (2022). RANS simulations of aerodynamic forces on a tall building under twisted winds considering horizontal homogeneity. Journal of Building Engineering, 54, 104628. https://doi.org/10.1016/j.jobe.2022.104628
- Zhang, X., Weerasuriya, A. U., Tse, K. T., & Kwok, K. C. S. (n.d.). Characteristics of pedestrian level wind environment in twisted wind flow around isolated buildings. ANBRE 15 & ASEM15. http://www.iasem.org/publication_conf/asem15/1.ISEM15/2t/T3B.4.SM120_1946F1.pdf.
- Zhang, Y. (2013). Study on wind veering effects on super-tall buildings. Harbin Institute of Technology. https://doi.org/10.7666/d.D420495.
- Zheng, C., Zhang, K., Liu, Z., Wu, Y., & Chen, W. (2019). Wind tunnel simulation of a thousand-meter high twisted wind flow. Harbin Gongye Daxue Xuebao/Journal of Harbin Institute of Technology, 51(12), 71–78. https://doi.org/10.11918/j.issn.0367-6234.201902072