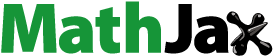
Abstract
When installed in deep tunnel systems, the cover of the vortex dropshaft may be partially or fully sealed. With air exchange restricted through the cover, the air supply to the dropshaft through the inflow channel might not be adequate. Hence, further investigation is required to examine the effect of the restricted air passage through the cover on the flow regimes and hydraulic performance of the scroll vortex dropshaft. This study aims to quantify the head-discharge relationship, air-water distribution, and minimum air core size with different cover opening conditions. The 3D model of a fully open scroll vortex dropshaft is constructed, and numerical simulations are carried out and validated using experiment data. The results show that the effect is generally small when the ratio of the opening and dropshaft area is larger than 1%. When the cover is fully sealed, the minimum air core size reduces by ~5% of the dropshaft area which can have a serious implication to the design of the scroll vortex dropshaft. The present study provides a practical evaluation of the effect of the opening condition in the cover of the vortex chamber on the performance of scroll vortex dropshafts for underground water drainage systems..
Notation
Aac | = | air core cross-sectional area |
B | = | width of the inflow channel |
D | = | shaft diameter |
Da | = | diameter of the vent hole |
e | = | shaft eccentricity |
ea | = | the relative error between two sets of simulation parameters |
E | = | total energy head throughout the flow field of the scroll vortex dropshaft |
Fr | = | Froude number |
Fs | = | the safety factor of the grid convergence index |
g | = | gravitational acceleration |
ha | = | the water level height of approach flow (at the monitoring surface S1) |
H | = | height of the inflow channel |
HL | = | the height of vertical dropshaft above water |
Ht | = | the height of vertical dropshaft |
Hs | = | the height of vertical dropshaft under water |
H(φ) | = | the Heaviside function |
k | = | turbulent kinetic energy |
ki | = | interface curvature |
kα, nα | = | coefficients associated with α |
L | = | length of the inflow channel |
N1, N2, N3 … | = | grid numbers of different schemes |
P | = | pressure |
Qa | = | air discharge through the vertical dropshaft |
Qah | = | air discharge through the vent hole |
Q | = | inflow discharge of the scroll vortex dropshaft |
Qd | = | design inflow discharge of the scroll vortex dropshaft |
Q* | = | dimensionless inflow discharge of the scroll vortex dropshaft |
r | = | radial distance |
rin | = | the distance from the inlet shaft centre |
rp | = | the grid refinement factor |
R1 | = | vortex chamber first arc radius |
R2 | = | vortex chamber second arc radius |
R3 | = | vortex chamber third arc radius |
R4 | = | vortex chamber fourth arc radius |
s | = | vortex chamber tongue length |
t | = | time |
u | = | the velocity vector field |
v(r) | = | the tangential velocity at the bell mouth area |
x | = | coordinates of flow field |
X | = | coordinate parallel to the approach channel |
Y | = | coordinate perpendicular to the approach channel |
Z | = | vertical coordinate |
α | = | the ratio of rin and B |
δ | = | shaft transition fillet |
δ(φ) | = | the Dirac Delta smoothing function |
ε | = | dissipation rate of turbulent kinetic energy |
λa | = | air core area ratio |
λm | = | the minimum air core area ratio |
μ | = | dynamic viscosity of fluids |
μ1 | = | dynamic viscosity of phase 1 |
μ2 | = | dynamic viscosity of phase 2 |
ξ | = | smoothing width on one side of the two-phase interface |
ρ | = | density of fluids |
ρ1 | = | density of phase 1 |
ρ2 | = | density of phase 2 |
σ | = | surface tension coefficient |
φ | = | a distance function to the two-phase interface |
Γ∞ | = | the tangential velocity circulation at the bell mouth area |
1 Introduction
The utilisation of deep tunnel stormwater systems is becoming more popular for integrated urban water management to modulate the peak discharge of urban runoff when urban extreme rainfall happens. Designing these underground systems requires the considerations of stormwater regulations as well as overflow control and pollution management (Wang et al., Citation2018). A crucial component is the dropshaft which conveys the flow from higher to lower elevations while dissipating the flow energy. For vortex dropshaft, the flow is guided along a helicoidal path down the vertical dropshaft, creating friction with the shaft wall with energy dissipation (Crispino et al., Citation2021). This swirling flow is produced by vortex chambers of different geometries, with different head-discharge relationships of approach flow (Mulligan, Plant, et al., Citation2019). With the approach flow Froude number Fr < 1, for subcritical flow, circular and scroll intakes are generally used. Among them, the scroll vortex intake has the main advantage of being stable hydraulically which was utilised as an energy dissipation device in hydropower projects (Drioli, Citation1947) and now widely adopted in urban drainage systems (Motzet & Valentin, Citation2002). It can also be designed for supercritical approach flow by creating a hydraulic jump with a negative step (Del Giudice et al., Citation2010), acting like spiral and tangential inlet structures (Hajiahmadi et al., Citation2022; Wei & Chang, Citation2023).
Studies on vortex dropshafts so far utilised model experiments extensively to establish the hydraulic characteristics. Examples in recent years include the study by Del Giudice and Gisonni (Citation2011) which addressed the issue of supercritical flow towards the dropshafts of the sewer system at Naples, Italy. Guo (Citation2012) conducted detailed flow measurements in the vortex chamber using Laser Doppler Anemometry. His results revealed that the distribution of tangential velocity inside the chamber follows a free vortex pattern, but the circulation at the bell mouth beneath is notably smaller. Mulligan et al. (Citation2016) compared the circulation and flow rate of strong free-surface vortices in various experimental models. Their results highlighted the significance of hydraulic control section as well as transition section in affecting the vortex flow regime. Mulligan, Creedon, et al. (Citation2019) compared the tangential velocity distributions of strong free-surface vortices inside the vortex chamber under different discharge conditions using Particle Tracking Velocimetry and Particle Image Velocimetry. Utilising the experimental results, they proposed a tangential velocity model based on the number of reverse cycles with empirical coefficients.
With the advancement in computing power, Computational Fluid Dynamics (CFD) methods are also gaining popularity in recent years as an effective tool to study the performance of vortex dropshafts. Plant and Crawford (Citation2016) and Carty et al. (Citation2019) demonstrated that the Volume of Fluid (VOF) and Mixture multiphase flow models, coupled with the SST turbulence model, yielded accurate predictions of the free-surface (with errors below 5%) in the vortex chamber. They suggested that the grid size and the turbulence model are most crucial influencing the simulation accuracy. Chang and Wei (Citation2022a, Citation2022b) employed 3D numerical simulations to investigate the rapid transition between the swirling flow in the vortex chamber and the spiral flow down the dropshaft. By comparing the pressure distribution, they determined that the minimum to maximum wall pressure ratios should exceed 0.1 at the intake cross-section and 0.2 during the falling process, respectively. Under high discharge conditions, the impact of entrance constriction on flow rotation performance becomes more pronounced. Chan et al. (Citation2022), Chan (Citation2022) investigated the air core size, tangential velocity distribution, and pressure gradient at the entrance bell mouth by numerical simulations. Their findings confirmed that the swirling vortex at the entrance to the dropshaft resembled a free vortex with an equivalent circulation. Notably, the use of CFD models has gained the high level of confidence to the extent that it is increasingly prevalent in the design stage.
Most research focused on the flow regime and velocity distribution of vortex chamber with fully opened cover (Del Giudice and Gisonni (Citation2011); Mulligan et al., Citation2016; Mulligan, Creedon, et al., Citation2019; Chan et al., Citation2022; Chan, Citation2022), but there has been no discussion on the other inlet structure with restricted air passage. In underground systems, for reducing cost, vent holes with limited diameter are usually used for air passage through the dropshaft and atmosphere. For studies of vertical dropshafts, the flow regime and air entrainment were found to be affected by limited air supply (Ding et al., Citation2018; Ding & Zhu, Citation2018). But for vortex dropshafts, this limits the supply of outside air for the entrainment need and affects the air core size λa inside the dropshaft (Mulligan et al., Citation2016). The current standard design practice for scroll vortex dropshafts is to maintain a minimum air core size λm of 25% of the cross-sectional area of the dropshaft (Jain & Ettema, Citation1987), but the minimum value with limited air supply is unknown. Consequently, in order to ensure stability in the hydraulic performance and avoid choking inside the dropshaft, an assessment of the cover vent condition is needed to quantify the effects on the hydraulic performance of the scroll vortex intake under different cover opening conditions.
In order to examine the effect due to the opening condition in the top cover of the vortex chamber on the minimum air core size of scroll vortex intake, it is necessary to first validate with fully open conditions. 3D modelling and numerical simulations based on a two-phase CFD model are first carried out for this objective. The use of two-phase CFD models together with the coupling of the Level-Set and Volume of Fluid (VOF) methods is known for its ability to accurately simulate the position of the air–water interface. After validation, the CFD model is then adopted to evaluate and assess the relative flow discharge and interface shape of water and air phases in partially or fully sealed cover conditions. In the following sections, the CFD model and its validation are first presented in Section 2. Section 3 discusses the results obtained. The conclusion of the present study is then drawn in Section 4.
2 Methodology
2.1 Governing equations
A two-phase CFD model based on a fixed Euler grid is used in this study. The model enhances the simulation of the interface curvature and surface tension by incorporating the Level-Set method while maintaining a constant volume fraction with the VOF method (Sussman & Puckett, Citation2000) which together can quantify the air–water interface more accurately. The Level-set method uses the zero isosurface function, , to define the two-phase interface (Sussman et al., Citation1998), where φ is defined as a distance function to the interface and x and t are coordinates and time, respectively. The convective transport equation for the interface can then be expressed accordingly as follows:
(1)
(1) where u represents the velocity vector field. In the momentum equation, the model incorporates the surface tension coefficient σ into the expression through the body force term:
(2)
(2) where ρ is the density, P is the pressure, g is the gravitational acceleration, μ is the dynamic viscosity, k is the interface curvature, δ(φ) is the Dirac Delta smoothing function defined as:
(3)
(3) where ξ represents the smoothing width on one side of the air–water interface which is typically taken as 1.5 times the grid size near the interface.
To ensure smoothness and avoid excessive variations of density, viscosity, and other parameters near the interface, their property is interpolated between that of the primary and secondary phase fluids indicated using subscripts 1 and 2, respectively. For example, the density and viscosity are defined with the Heaviside function H(φ) as follows:
(4)
(4)
(5)
(5)
The CFD method adopts the RNG k-ε turbulence closure developed by Yakhot et al. (Citation1992). The closure exhibits good performance in capturing strong streamline bending that occurs in swirling and vortex flows, and is thus suitable for the simulation of the flow field of scroll vortex dropshafts (Chan, Citation2022; Chan et al., Citation2022). The turbulent kinetic energy k equation and turbulent dissipation rate ε equation are as follows:
(6)
(6)
(7)
(7) where
;
;
; ui is velocity; xi and xj are coordinates; Gk is the generation term of the average velocity gradient turbulent kinetic energy; Sij is the tensor of deformation rate, η0, β, C1ε, C2ε, Cμ, σk and σε are constants.
Equations (1) to (7) together are solved numerically using the commercial CFD software ANSYS FLUENT version 19.2 (ANSYS Inc, Citation2018).
2.2 Design geometry and theories
The geometry of the scroll vortex intake utilised for assessment in this study is based on the physical model of Guo (Citation2012) with extensive velocity measurements. The geometry of the vortex chamber, inflow channel, and vertical dropshaft is depicted in Figure (a). The dimensions of each component adhere to the standard design proposed by Jain and Ettema (Citation1987), and the specific parameters and their values are shown in Table . In addition to the scroll vortex intake geometry, the inflow condition plays a crucial role in the formation of the free-surface vortices. Thus, the classification of vortex dropshafts is typically based on the inflow Froude number distinguishing between subcritical flows (Fr < 1) and supercritical flows (Fr ≥ 1), with subcritical flows being the most common in practical applications of scroll vortex dropshafts.
Figure 1. (a) Geometry definition and (b) 3D computational mesh of scroll vortex dropshaft with a vent hole.

Table 1. Dimensions of scroll vortex dropshaft model in Guo (Citation2012).
Binnie and Hookings (Citation1948) made several assumptions as follows to derive the design inflow discharge Qd of the scroll vortex dropshaft:
The total energy head E remains constant throughout the flow field, i.e. assuming no energy loss within the dropshaft.
The flow is symmetric and non-rotating around the shaft axis. The tangential velocity circulation Γ∞ at the bell mouth area can then be determined as
where v(r) is the tangential velocity and r is radial distance.
The pressure in the air core (with the air core area ratio λa at the horizontal section) remains atmospheric.
Based on the above assumptions with the three unknown quantities (total energy head E, tangential velocity circulation Γ∞ and air core area ratio λ), Ackers and Crump (Citation1960) derived an expression for Qd as:
(8)
(8) Mulligan et al. (Citation2016) simplified the equations and introduced a semi-empirical model as follows:
(9)
(9) where the dimensionless parameter α represents the ratio of the distance rin from the inlet shaft centre and the width B of the inflow channel, expressed as:
(10)
(10) Substituting the geometric parameters from Table into Equation (10), α is calculated as 1.494 in this structure. Based on experimental results of Mulligan et al. (Citation2016), the constant kα and exponent nα are 2.85 and 0.985, respectively. Substituting the values of α, kα, nα, and geometric parameters into Equation (9), Qd for this scroll vortex intake geometry model can be calculated to be approximately ∼10 L/s.
2.3 Model grid, boundary and initial conditions
The ANSYS ICEM (ANSYS Inc, Citation2018) software is utilised to generate a hexahedral structured grid for the numerical domain of the scroll vortex dropshaft as illustrated in Figure (b). The mass flow inlet is employed as the inflow boundary condition, and the water level of the open channel flow at the inlet is specified for the corresponding inflow discharge. The bottom of the vertical dropshaft and the top cover of the vortex chamber and inflow channel are set as pressure outlets, while the wall surfaces are treated as non-slipping boundaries. We note that the fully open condition of the cover will be varied later in Section 3 to assess the effect of partially or sealed conditions. The gas phase (air) model is defined as an ideal gas, and the liquid phase (water) model is defined as an incompressible liquid without considering heat exchange. The influence of gravity as a driving force is taken into account with an acceleration of 9.81 m2/s in the negative Z-axis direction.
The pressure and velocity fields are simulated using the PISO algorithm with a convergence criterion of 10−3. The timestep is set as 0.01s, and the simulation continues until the water level height ha at the monitoring surface S1 (located 70mm in front of the entrance of vortex chamber as shown in Figure (a)) stabilises. Typically, the stabilisation occurs within the simulation time of 100s under different inflow conditions, with a computation time of approximately 10h using a Dell workstation with an Intel Xeon Gold 6258R 2.7 GHz central processing unit (CPU) having 20 cores for parallel computation.
In order to verify the grid convergence, the simulation results with three schemes having different grid numbers, namely N1 = 95,000, N2 = 274,000, and N3 = 799,000, are compared. The convergence is evaluated based on the grid convergence index (GCI) recommended by American Society of Mechanical Engineers (ASME), and the formula is expressed as (Celik et al., Citation2008):
(11)
(11) where Fs is the safety factor which is typically set to 1.25, ea represents the relative error between two sets of simulation parameters (specifically the water level height ha at the monitoring surface S1 in this study), and the grid refinement factor rp indicates the mesh size ratio which should be consistent between each two adjacent grid schemes as follows:
(12)
(12)
(13)
(13) which i, j = 1, 2 or 3. The results on numerical convergence are presented in Table . Scheme 3 satisfies the grid accuracy requirements (GCI = 0.6%) and is therefore adopted in the present study. The maximum cell aspect ratio of it is 7.937, and the inflation layer with a first layer thickness is 0.67mm. In all cases, the Y + value is from 50 to 215 which is suitable for RNG k-ε turbulence model.
Table 2. Grid convergence.
2.4 Experimental data for model validation
The CFD simulation results are compared with the experimental data from Guo (Citation2012) for validation under different inflow discharge conditions of Q/ Qd = 0.1∼1.0. Here, the dimensionless flow discharge Q* is introduced and defined as as well as the dimensionless water level ha/D. The characteristic curves obtained from the simulation results compared to the experimental measurements are shown in Figure (a). It can be seen that the simulation results follow the trend of measured head-discharge relationship closely. Based on the deviation percentage between the measurements and simulations for the dimensionless water level ha/D under the same inflow discharges, the CFD predictions could be deemed to have a maximum relative error of 3%.
In this paper, the air core area ratio is defined as where Aac is the air core cross-sectional area at the horizontal cross-sections of S2-S22 as shown in Figure (a). The vertical variation of air core size from the present numerical results is plotted in Figure (b) together with the measurements from Guo (Citation2012) and CFD simulation results from Chan (Citation2022). It is evident that the minimum air core sizes predicted by the two CFD simulations are noticeably smaller than the experimental results by Guo, and even fall below the design value of 0.25. One reason for this disparity is the possible deviation due to the use of a 50% air volume fraction as the air–water interface in the simulations which might differ from the actual position of the interface observed visually in the experiments. Despite this difference, the simulation results in the present study with the fully open condition can still provide the quantitative insights and serve as a reference to assess the effects due to different opening conditions in the cover of the vortex chamber in the following sections.
3 Results and discussion
3.1 Hydraulic characteristics
In this section, the cover of the vortex chamber and top of the inflow channel are now closed for air passage, except for a single opening through the cover with a diameter Da along the axis of the vertical shaft as shown in Figure (a). The cover opening condition is varied within the range of dimensionless hole diameter Da/D = 0∼2, where Da/D = 0 is the completely sealed condition. The flow regime of inflow channel, with the Froude number as a reference, is a key factor influencing the state of free-surface vortices in the vortex chamber. The comparison of Froude numbers under different cover opening and inflow conditions are presented in Figure , where the dotted lines indicate the Froude numbers of the original fully open dropshaft and scattered points represent the simulation results with restricted air passage. It can be seen that under high inflow discharge conditions which the Froude number is relatively small, the effect on Fr due to the cover opening condition is also small. Notably, the maximum deviation of the Froude number is 1.8% under the inflow condition of 1.0Qd. However, when the discharge reduces, the deviation increases reaching 13.7% at 0.2Qd. Comparing different hole diameters, the effect is more significant when Da/D ≤ 0.5, and becomes clearly noticeable when Da/D ≤ 0.1.
3.2 Air–water distribution and air core size comparison
The air–water distribution under different cover opening conditions are compared in Figure at the planes of Y = 0 and Z = 0 (Y = 0 is the plane passing through the axis of vertical shaft parallel to the channel inlet; Z = 0 represents the bottom of the vortex chamber), where the coordinates of X, Y and Z are shown in Figure (b). From Figure (a), a distinct air core can be observed extending from the top of the free-surface vortex to the spiral flow in the dropshaft. The air core exhibits similar shapes under different cover opening conditions that resemble an asymmetrical trumpet. At the bottom plane of vortex chamber (as shown in Figure (b)), the shape of air core becomes more circular and its geometric centre is more inclined to the R3 area of the vortex chamber. The air core size reaches its minimum value shortly after the entrance and then slow increases as the flow progresses down the vertical dropshaft. It can be observed that the water level in most areas of the vortex chamber is slightly lower under the fully open condition compared to partially or fully sealed conditions. In the bell mouth region, the width of air core is notably larger when Da/D = 0.1.
Figure 4. Air core shape for design flow discharge under different cover opening conditions at specific sections of (a) Y = 0; (b) Z = 0.

The changes in air core area ratio are plotted in Figure (a) under different cover opening conditions with the design discharge of 1.0Qd. As the vertical elevation decreases, the air core size decreases reaching a minimum below the intake floor and then increases. Comparing the different cover opening conditions, it is observed that when the top of vortex chamber is completely sealed, the minimum air core area ratio reduces to only 19.2% with the design inflow discharge. The comparison of the minimum air core size λm and inflow discharge condition is further assessed in Figure (b). From the figure, it can be observed again that the minimum air core size ratio with restricted cover condition is generally lower than the original fully open condition. With the smallest hole size (Da/D = 0.1), λm is 4.6%, 13.1%, and 12.9% less than that of the open dropshaft with the inflow discharge Q/Qd of 1.0, 0.8, and 0.6, respectively. We note that although the net reduction of 4.6% in the area ratio compared to the fully open condition is small under the design flow, it might have serious implications since the scroll vortex dropshaft might no longer satisfy the minimum requirement.
3.3 Air entrainment
The air entrainment has always been a matter of important concern for scroll vortex dropshafts. To characterise the air entrainment, the relative entrained air discharge is defined as Qa/Q where Qa represents the air discharge at the bottom of dropshaft and Q represents the water inflow discharge. Viparelli (Citation1961) concluded that the air entrainment effect is related to the height of vertical dropshaft Ht. Based on the submerged depth downstream, Ht is divided into the height above water HL and under water Hs. Qa/Q can then be expressed as:
(14)
(14) Vischer and Hager (Citation1995) further suggested that the air entrainment with the low inflow discharge condition is correlated with the velocity of water in the open channel flow. They introduced the dimensionless flow discharge Q* and expressed the relative entrained air discharge as:
(15)
(15) Mahmoudi-Rad and Najafzadeh (Citation2021) included the impact of air mixing at the bottom of the dropshaft into account. They performed a linear regression analysis using HL, Hs, D and Fr, and the best-fit relationship is:
(16)
(16) In this study, the numerical domain of scroll vortex dropshaft adopts an open bottom configuration with constant Ht. As a result, the cover opening condition and Fr become the key variables that influence the air entrainment.
The variation in cover opening conditions and Froude numbers is represented by different hole diameters (Da/D = 0∼2) and various inflow rates (Q/Qd = 0∼1). The change in the relative entrained air discharge is illustrated in Figure (a), where dotted lines show simulation results of the original dropshaft, and scattered points represent the entrained air discharge corresponding to different hole diameters. The results indicate that the relative entrained air discharge is not affected by the opening area when the diameter range is sufficiently large (Da/D>∼0.2). However, when the opening further decreases, air inflow becomes increasingly restricted resulting in a decrease in the air entrainment discharge. When compared to the fully open dropshaft, the relative entrained air discharge decreases by more than 85% with a small opening area (Da/D = 0.1). Using the least square method and building a piecewise polynomial with Da/D = 1 as the reference, in conjunction with the simulation results of Fr shown in Figure , the relative entrained air discharge Qa/Q related to Fr and Da/D can be expressed as:
(17)
(17) Figure (b) plots the opening air inflow ratio Qah/Qa where Qah is the air inflow discharge through the cover opening hole by combining with Fr in Figure . It can be observed that the opening air inflow ratio increases with both the Froude number and opening area, and is consistently maintained above 70%. Therefore, it can be concluded that the air inflow through the cover of the vortex chamber serves as the primary source of air entrainment for the dropshaft, while the air flow carried by the open channel water inflow has limited influence. However, when the opening area reduces to Da/D = 0.1, the air inflow ratio becomes smaller and the air entrainment of the dropshaft is significantly reduced. This finding can be insightful towards the design of exhaust control in deep tunnel systems together with the reduction in the minimum air core size.
4 Conclusions
Scroll vortex dropshafts are commonly adopted in urban drainage systems nowadays, however the cover opening condition of the vortex chamber can affect their performance in underground systems. In this study, numerical simulations are conducted to quantify the effects based on the experimental setup of Guo (Citation2012). The following conclusions are summarised: The opening area of cover affects the water level height ha at the entrance of the vortex chamber, particularly in low inflow discharge conditions. The maximum deviation in Froude number compared to the fully open condition occurs under fully sealed condition, and is 1.8% with Qd, and 13.7% with 0.2Qd. Significant effect on the minimum air core area ratio only occurs when the cover is almost fully sealed, i.e. when the cover opening area is less than 1% of the dropshaft area or Da/D < 0.1. In this situation, λm is 4.6%, 13.1% and 12.9% less than that of the fully open dropshaft with Q/Qd of 1.0, 0.8, and 0.6, respectively. The proportion of air inflow through the cover remains relatively high despite a very small opening area, typically above 70%.
Disclosure statement
No potential conflict of interest was reported by the author(s).
Data availability statement
All relevant data are included in the paper or its Supplementary Information.
Additional information
Funding
References
- Ackers, P., & Crump, E. S. (1960). The vortex drop. Proceedings of the Institution of Civil Engineers, 16(4), 433–442. https://doi.org/10.1680/iicep.1960.11720
- ANSYS Inc. (2018). ANSYS Fluent 19.2 theory guide.
- Binnie, A. M., & Hookings, G. A. (1948). Laboratory experiments on whirlpools. Proceedings of the Royal Society of London. Series A. Mathematical and Physical Sciences, 194(1038), 398–415.
- Carty, A., O’Neill, C., Nash, S., Clifford, E., & Mulligan, S. (2019). Hydrodynamic modelling approaches to assess mechanisms affecting the structural performance and maintenance of vortex drops shaft structures. Journal of Structural Integrity and Maintenance, 4(3), 162–178. https://doi.org/10.1080/24705314.2019.1622188
- Celik, I. B., Ghia, U., Roache, P. J., & Freitas, C. J. (2008). Procedure for estimation and reporting of uncertainty due to discretization in CFD applications. Journal of Fluids Engineering-Transactions of the ASME, 130(7), 1–4.
- Chan, S. N. (2022). Insights from three-dimensional computational fluid dynamics modelling of a scroll vortex intake. Journal of Hydraulic Research, 60(3), 408–422. https://doi.org/10.1080/00221686.2021.2001591
- Chan, S. N., Guo, J., & Lee, J. H. (2022). Physical and numerical modeling of swirling flow in a scroll vortex intake. Journal of Hydro-Environment Research, 40, 64–76. https://doi.org/10.1016/j.jher.2021.11.004
- Chang, L., & Wei, W. (2022a). Numerical investigation of the plunging and vortex-flow regimes occurring in drop shafts with a tangential intake. Journal of Irrigation and Drainage Engineering, 148(8), 04022026. https://doi.org/10.1061/(ASCE)IR.1943-4774.0001692
- Chang, L., & Wei, W. (2022b). Numerical study on the effect of tangential intake on vortex dropshaft assessment using pressure distributions. Engineering Applications of Computational Fluid Mechanics, 16(1), 1100–1110. https://doi.org/10.1080/19942060.2022.2072954
- Crispino, G., Contestabile, P., Vicinanza, D., & Gisonni, C. (2021). Energy head dissipation and flow pressures in vortex drop shafts. Water, 13(2), 165. https://doi.org/10.3390/w13020165
- Del Giudice, G., & Gisonni, C. (2011). Vortex dropshaft retrofitting: case of Naples city (Italy). Journal of Hydraulic Research, 49(6), 804–808. https://doi.org/10.1080/00221686.2011.622148
- Del Giudice, G., Gisonni, C., & Rasulo, G. (2010). Design of a scroll vortex inlet for supercritical approach flow. Journal of Hydraulic Engineering, 136(10), 837–841. https://doi.org/10.1061/(ASCE)HY.1943-7900.0000249
- Ding, Q., Ma, Y., & Zhu, D. Z. (2018). Air entrainment in a vertical dropshaft with limited air supply. Theoretical and Applied Mechanics Letters, 8(5), 315–319. https://doi.org/10.1016/j.taml.2018.05.002
- Ding, Q., & Zhu, D. Z. (2018). Flow regimes in a dropshaft with limited air supply. Journal of Hydraulic Engineering, 144(5), 06018006. https://doi.org/10.1061/(ASCE)HY.1943-7900.0001455
- Drioli, C. (1947). Jet Su un particolare tipo di imbocco per pozzi di scarico. L’Energia Elettrica, 24(10), 447–452.
- Guo, J. (2012). Velocity field measurement of a scroll vortex intake flow. Master of Philosophy thesis [Unpublished doctoral dissertation]. University of Hong Kong, Hong Kong. https://hub.hku.hk/handle/10722/161583
- Hajiahmadi, A., Crispino, G., Ghaeini-Hessaroeyeh, M., & Gisonni, C. (2022). Effect of the flow regime on the hydraulic features governing the operation of vortex drop shafts with spiral inlets. Water Science & Technology, 86(5), 1095–1107.
- Jain, S. C., & Ettema, R. (1987). Swirling flow problems at intakes. In J. Knauss (Ed.), Vortex intakes, IAHR hydraulic structures design manual 1 (pp. 125–137). Balkema.
- Mahmoudi-Rad, M., & Najafzadeh, M. (2021). Air entrainment mechanism in the vortex structure: Experimental study. Journal of Irrigation and Drainage Engineering, 147(5), 04021007. https://doi.org/10.1061/(ASCE)IR.1943-4774.0001545
- Motzet, K. M., & Valentin, F. (2002). Efficiency of a vortex chamber with horizontal bottom under supercritical flow. In Proc 9th international conference on urban drainage, Portland, 1–11.
- Mulligan, S., Casserly, J., & Sherlock, R. (2016). Effects of geometry on strong free-surface vortices in subcritical approach flows. Journal of Hydraulic Engineering, 142(11), 04016051. https://doi.org/10.1061/(ASCE)HY.1943-7900.0001194
- Mulligan, S., Creedon, L., Casserly, J., & Sherlock, R. (2019). An improved model for the tangential velocity distribution in strong free-surface vortices: An experimental and theoretical study. Journal of Hydraulic Research, 57(4), 547–560. https://doi.org/10.1080/00221686.2018.1499050
- Mulligan, S., Plant, J., Nash, S., & Clifford, E. (2019). Vortex drop shaft structures: state-of-the-art and future trends. In Proc 38th IAHR world congress-water connecting the world, Panama, 3860–3869 September.
- Plant, J., & Crawford, D. (2016). Pushing the limits of tangential vortex intakes: is higher capacity and flow measurement possible in a smaller footprint? In Proc WEFTEC 2016, water environment federation, New Orleans.
- Sussman, M., Fatemi, E., Smereka, P., & Osher, S. (1998). An improved level set method for incompressible two-phase flows. Computers & Fluids, 27(5-6), 663–680. https://doi.org/10.1016/S0045-7930(97)00053-4
- Sussman, M., & Puckett, E. G. (2000). A coupled level set and volume-of-fluid method for computing 3D and axisymmetric incompressible two-phase flows. Journal of Computational Physics, 162(2), 301–337. https://doi.org/10.1006/jcph.2000.6537
- Viparelli, M. (1961). Les courants d'air et d'eau dans les puits verticaux. La Houille Blanche, 47(6), 857–869. https://doi.org/10.1051/lhb/1961053
- Vischer, D. L., & Hager, W. H. (1995). Vortex drops. In Energy dissipators: Hydraulic structures design manual, Rotterdam, The Netherlands (pp. 167–181). Rotterdam, The Netherlands: Balkema.
- Wang, H., Mei, C., Liu, J., & Shao, W. (2018). A new strategy for integrated urban water management in China: Sponge city. Science China Technological Sciences, 61(3), 317–329. https://doi.org/10.1007/s11431-017-9170-5
- Wei, W., & Chang, L. (2023). Analytical solutions for vortex flow at the tangential inlet of a vertical dropshaft. Physics of Fluids, 35, 015160. doi:10.1063/5.0135575
- Yakhot, V., Orszag, S. A., Thangam, S., Gatski, T. B., & Speziale, C. G. (1992). Development of turbulence models for shear flows by a double expansion technique. Physics of Fluids A: Fluid Dynamics, 4(7), 1510–1520. doi:10.1063/1.858424