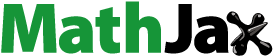
Abstract
The dynamic characteristics of the pumped storage unit (pump-turbine runner) make it highly susceptible to vibrations. Previous studies seldom addressed the clearance variation due to runner vibration, largely because of two challenges: the integration of the moving grid with the clearance and governing equations, and the intricacy of factoring in the entire shaft system's influence on vibration. By employing user-defined functions (UDF), kinematic equations for the pump-turbine runner's rotation and translation were formulated and integrated with computational fluid dynamics results. This paper introduces a computational method to simulate clearance-induced vibration displacement. The study examines the impact of clearance vibration displacement on the pump-turbine's transient flow field and runner vibrations under various operating conditions. Notably, deviations from the rated load condition led to an expansion-contraction trajectory of the runner axis, culminating in chaotic behaviour in the "S" characteristic zone. The correlation between runner vibrations and pressure fluctuations strengthened with the clearance displacement model, especially in narrow labyrinth seal clearances. Simulations also provided a refined estimation of the secondary rotational speed increase during load rejection. The method's reliability is confirmed through field test data comparison, offering a fresh perspective to identify the vibration characteristics of the pump-turbine runner.
1. Introduction
The development of hydropower with strong power regulation performance has caught the attention of the energy sector due to the rapid increase in global electricity demand and the ongoing transition to a clean energy structure. The difficulty of regulating the electricity system has grown due to developing several new clean energy sources (Zhang et al., Citation2023), such as offshore wind and photovoltaic power generation. And installing appropriate supporting pumped storage units can significantly improve the stability of the power network. Pumped storage can optimize the system's utilization of energy resources, enhance the worth of other energy sources, and realizes the spatial and temporal transfer of electricity in various values and qualities. Pumped storage power plants have the potential to regulate the power system, which increases the flexibility of the power system and contributes significantly to the stable functioning of the power network. These properties include peak control, frequency regulation, energy storage, etc. The pump-turbine runner is essential to preserving stability during operation as one of the most vital hydro-mechanical parts of pumped storage power plants. (Li et al., Citation2015; Liang et al., Citation2022) Due to the complicated flow characteristics of the ‘Anti-S zone' (Suh et al., Citation2021)and ‘Hump zone' (Gong et al., Citation2022; Zhao et al., Citation2022) and the frequent switching of its operating circumstances, the pump-turbine exhibits frequent hydraulic instability issues due to the features of both the hydraulic turbine and pump. As a result, ensuring the pump-turbine's operating stability is a prerequisite for it to perform an effective function.
It has been discovered that the pressure and distribution of fluid in the runner clearance significantly affect the force acting on the runner body (Liu & Tan, Citation2018; Nan et al., Citation2022; Hou et al., Citation2021) Many researchers are now exploring the numerical model of the turbine by including the clearance flow model in addition to simply the simple runner body as they continue to demonstrate the non-negligible influence of the gap on the runner. (Trivedi & Dahlhaug, Citation2018; Li et al., Citation2021) However, because of the outdated research techniques, it is challenging to precisely analyze the clearance flow in rotating units. The clearance flow path should be fully taken into account when examining the stability of pumped storage units. The runner vibration's radial displacement will alter the circumferential clearance's pressure distribution and size. Whereas the axial displacement will primarily alter the pressure inside the cavities of the crown and band, which in turn alters the magnitude of the axis displacement. This makes it necessary to develop runner dynamics equations (Li et al., Citation2018) to describe the coupling phenomenon and to improve the accuracy of the flow field study of pumped storage units. The investigation of the dynamics of multiscale fluid-runner interactions during transient process (Chen et al., Citation2021; Fu et al., Citation2022; Xu et al., Citation2022) in pumped storage units could enhance the stability of safe operation of pumped storage plants. The kinetic equations, however, are either exclusively applied to local components in previous research or are primarily employed for fluids. There is still room for improvement in the application of kinetic equations in this field because few researchers have applied them to the entire rotating equipment.
Numerical simulations are challenging to comprehend since pumped storage power facilities include sophisticated multiscale overflow systems (Zhou, Chen, et al., Citation2019) (scale gaps from the 100-meter level to the millimeter level). The effect of various cavity gaps on the cavity pressure of Francis Turbine was revealed by Zhou and Chen (Citation2015) through the simulation of multiscale flow in Francis turbine, and the accuracy of the calculation was confirmed. In recent years, researchers have been studying multiscale flow in hydraulic machinery. It has also been demonstrated that the clearance flow causes changes in the radial and axial forces acting on the runner (Zhou, Wu, & Su, Citation2019). In addition, the 3D numerical simulation method taking into account the labyrinth gap flow could more accurately predict the rotational speed and force changes of the runner during the transition of the hydraulic unit (Fu et al., Citation2018; Trivedi & Cervantes, Citation2017). The significance of the gap flow has also been emphasized in numerous studies on the flow response of the blade tip clearance (Kim et al., Citation2019; Kan et al., Citation2022; Liu et al., Citation2021) and the guide vane clearance.(Chitrakar et al., Citation2018; Sentyabov et al., Citation2019) The above research progress demonstrates that the clearance structure associated with the main flow channel and its flow characteristics have a notable significance on the stability of the unit. However, the research on the evolution of the transient process of the sealed clearance flow field is not sufficiently in depth, and the shift in the clearance flow path brought on by the change in runner position is not taken into account. Additionally, the interaction mechanism between various flow scales is rarely considered.
The majority of recent research has considered the deformation factors of solid components, (Waqas & Ahmad, Citation2020) and fluid-solid coupling techniques (Elhami et al., Citation2021; Li et al., Citation2022; Nakhchi & Rahmati, Citation2021) are frequently employed to examine unit vibrations. A hydraulic machine's nonconstant flow could cause variations in the forces acting on the corresponding solid walls, resulting in deformation or position changes in the solid components. These changes in the solid components then have an impact on the flow structure and cause unit vibrations. Using the unidirectional fluid-solid coupling technique, Liang et al. (Liang et al., Citation2020) investigated the stress–strain characteristics of a runner body with a cavity structure in addition to the internal flow field of the runner cavity structure. To study the stress distribution of the runner and the characteristics of the law of fluid internal flow, Liu et al. (Citation2021) investigated crack generation in a Francis turbine runner after modification; Qu et al. (Citation2019) used bidirectional fluid-structure coupling to calculate a turbine for a cooling tower and concluded that the primary influencing factor of blade vibration was engendered by the dynamic and static interference of the structural elements. To investigate hydraulic excitation and the law of structural vibration during unit operation, Zhang et al. (Citation2020) developed a three-dimensional water-machine-concrete structural coupling model for hydropower plants. The aforementioned study only accounted for the deformation effect of solid components; the change in solid component locations was not considered due to restrictions in the structural dynamics analysis.
In the existing research, it is evident that the study of runner clearance has garnered increasing attention from scholars. However, it is also acknowledged that researching this aspect presents significant difficulties and challenges. The primary challenges revolve around the implementation of small-scale dynamic grid techniques and the consideration of the dynamic response of the entire shaft system. In this study, the runner's clearance flow and the change in clearance shape are both taken into account. The three-dimensional simulation method with a user defined function (UDF) and dynamic grid technology are combined to resolve the issues of cross-interface data transfer and clearance grid reconstruction. Simulating the process of the runner clearance changing with the runner vibration and the algorithm's dependability is confirmed by comparing the field test data. The method offers a fresh perspective on how to study the characteristics of the runner dynamics and the operational stability of the pump-turbine, and it can also be utilized for determining the stability of the runner under various operating conditions. The remainder of this study is structured as follows. Section 2 presents the utilized geometric model and numerical methods, including the creation of dynamical equations and the application of dynamic meshes. The study results are compared and validated with field tests, and the vibration characteristics of the pump-turbine under various operating situations, as well as the pressure pulsation characteristics of the runner clearance are explored in Section 3. Finally, the study's primary concluding remarks are included in Section 4.
2. Numerical methodology
2.1. Computational model and mesh description
A pumped storage power plant in China is studied in this paper, and its key features are listed in Table .
Table 1. Basic parameters of the runner.
The geometry built by using Creo software (Figure ) includes water diversion channel, a spiral casing(SC), stay vanes(SV), guide vanes(GV), a runner, a draft tube(DT), clearances, balance pipes, and decompression tubes. Four balance pipes with a 50 mm diameter are installed between the crown clearance and band clearance, and four decompression tubes with a 219 mm diameter are set at the crown clearance of the unit section.
A trigonal mesh with good adaptability to mesh reconstruction is used for meshing the movable guide vanes, and the spiral casing is separated into unstructured mesh, while the rest of the computational domains are divided into hexahedral structured grids. Figure depicts a portion of the meshing of the computational domain. To save computational resources and reduce the influence of the mesh on the calculation results, five meshing options are adopted for the model in this study, and grid-independent verification is based on the axial force, flow rate and efficiency. Option 4 was chosen for further calculations after critical evaluation, and the outcomes are displayed in Tables and .
Figure 2. Distributions mesh node (a) Grid coupling of different scales (b) Gids on unit (c) Gids on the crown and band clearance.

Table 2. Five meshing schemes.
Table 3. Number of grid cells in scheme four.
2.2. Governing equation
The fluid in the multiscale flow channel of the pump-turbine is an incompressible viscous turbulent flow, and the effect of temperature on the fluid is ignored in this study, so the energy equation is not considered, and several common fluid equations such as the N-S equation, the continuity equation, and the momentum equation are employed as the basic control equations.
The motion of the runner clearance is realized by the runner dynamics equation. Due to the complexity of the shafting system of the pump-turbine set, which consists of the turbine main shaft, generator rotor, turbine runner, guide bearing (upper guide bearing, lower guide bearing, and water guide bearing), and thrust bearing, a simplified shaft system model is used in this study to simplify the computation. This model ignores the length and rigidity of the main shaft and concentrates the mass of all spinning components into a rigid mass system. The thrust bearing is simplified to an axial constraint while the guide bearings are simplified to a radial constraint. The simplified model is shown in Figure .
Set (x, y, z) as the form center coordinates of the rotating sections in the simplified schematic above, and based on Newton's second law, the system's kinematic equation is:
(1)
(1)
(2)
(2)
(3)
(3) where m is the mass of the rotating parts, including the mass of the turbine runner, generator rotor and turbine main shaft, k1 and k2 are the radial and axial stiffnesses taken as 600 and 8000 MPa respectively; and c1 and c2 are the radial and axial damping coefficients taken as 108 and 5 × 107 respectively. Fx, Fy, Fz are the fluid excitation forces on the runner in the x, y and z directions, respectively, and g is the acceleration of gravity.
2.3. Boundary conditions, turbulence model and discrete methods
Gravity's effect on the flow field was considered in the computation. User-defined functions (UDFs) were used to set the inundation pressure distribution at the inlet and outlet boundaries. The outlet pressure of the draft tube at each time step is used as the pressure value of the pressure decompression tube outlet and will be determined by a user-defined function (UDF) as well.
As a ‘scale-adaptive' model, the SAS-SST model was selected as the turbulence model (Hou et al., Citation2021), it effectively captures the turbulent behavior of local flow characteristics, making it particularly well-suited for flows spanning multiple scales, from the entire flow system to clearance flows, which combines the best features of multiple models and uses the wall function method to provide stable and accurate predictions in the near-wall region. Engineering practice permits the average value of y + in the near-wall region of the main components to be less than 15, even if it does not match the high accuracy criteria of the turbulence model. (Deng et al., Citation2022; Liu et al., Citation2020) The no-slip boundary condition is imposed on the solid walls. The pressure and velocity fields are solved using the SIMPLEC method, which typically excels in handling flow simulations with a wide range of scale variations. And a more reliable second-order windward format with second-order accuracy is adopted. The central difference format is employed as the discretization method for the diffusion term, and the time domain discretization format follows an implicit integration strategy.
2.4. Dynamic mesh technique
To realize the position change of the clearance, UDF programming is utilized to assign the motion velocity of the runner body in three dimensions. The layered method dynamic mesh technique is used to simulate the clearance variability during the runner vibration since the runner clearance model uses structured meshing to divide. The crown clearance motion domain is schematically represented in the top half of Figure . The schematic diagram of the grid change of the clearance meridian profile boundary is shown in the bottom half of Figure , where figure(a) the lower boundary moves upward in the Figure (corresponding to the runner's upward vibration) and figure(b) the right boundary of the clearance moves to the left in the Figure (corresponding to the left vibration of the runner).
3. Results and discussion
3.1. Flow-induced vibration characteristics analysis in the pump-turbine runner under multiple load conditions
Four distinct load conditions were simulated and compared with the test data. The test consists of four groups of data in the load dumping test, including 100%, 75%, 50%, and 25% of the rated load. The efficiency of the unit is highest in working condition 1, which is also close to the rated condition. The opening of the guide vane gradually decreases from working conditions 2–4. The for levels of guide vane opening were 26.6°, 18.1°, 12.4° and 9.6°. As indicated in Table , the computed results are contrasted with the field test vibration data.
Table 4. Vibration data comparison under various working conditions.
The vertical displacement of the generator under-rack is the axial displacement of the field test, and the calculation result is closer to the field test result. The water guide bearing displacement measured in the test as radial displacement data, the calculated value and the test value are of the same magnitude, with only a few deviations, primarily because only the hydraulic excitation force is considered in the calculation, without taking into account the role of factors such as nonlinear electromagnetic pull and eccentric force generated by runner condition change, while the effects of shaft length and torque on the calculation are also not taken into consideration.
The scatter diagram of radial force is displayed in Figure , and the scatter points indicate the distribution of two perpendicular radial forces exerted on the runner at a certain moment in 10 spinning cycles. The axial force scatter distribution of cases 1 and 3 is an annulus, with a higher scatter distribution density in the circle of Case 1. There is an eccentric state biased toward the + x and -y directions in Case 3, with a lower scatter distribution density; Case 2 is nearly elliptical in shape; Case 4 has a circular radial force distribution, and the distribution density declines from the center to the surroundings.
Due to the characteristic frequencies of the runner vibration in the x-direction and y-direction in each case being the same, there are only slight differences in the values. Figure displays only the vibration spectrum in one direction (x-direction) and the axial trajectory curve of the runner dynamics response of the pump-turbine under four steady-state working conditions. Since case 1 is close to the rated working condition, the flow trajectory inside the runner is smooth with no additional disturbance frequencies, and thus, the primary vibration frequencies are related to the rotational frequency fn. Cases 2 and 3 should be emphasized as they exhibit the largest vibration ranges. In these cases, the low-frequency tailwater vortices (0.16fn and 0.25fn) become the dominant factors affecting the runner's vibration. The presence of the bend in the draft tube results in an asymmetrical elliptical shape of the runner's vibration trajectory. Case 4, which operates with a smaller guide vane opening, runs in the unstable ‘S’ zone within the characteristic curve, resulting in unstable internal flow and relatively chaotic fluid excitation force, so the shape of the axial trajectory is irregular. However, due to the reduced flow rate in this case, the amplitude of runner vibration decreases. Moreover, the runner's vibration frequency component is more complex in this case showing that there are more low-frequency components. The primary frequency is low (0.6fn), but there are also high-frequency components present at this time, including rotational frequency and runner blade passing frequency.
Figure 6. Vibration frequency spectrum (x-direction) and axial trajectory of the runner in four cases.

Figure displays the distribution of draft tube vortex bands (at the same time) when the vortex identification method is the Q-criterion and the value is 0.008. These distributions clearly illustrate that the occurrence of tailwater vortices in cases 2 and 3 closely matches the range of radial forces applied to the runner and the extent of vibration displacement. The water flow at the hydraulic turbine outlet can be simply accepted as the combination of top-down vertical motion generated by gravity and rotational motion caused by the rotation of the runner. A schematic diagram of the circular section of the turbine outlet is illustrated in the middle of Figure . In case 1, the maximum guide vane opening corresponds to the maximum flow, and the high-velocity area dominated by gravity is concentrated in the A1 region, which can be interpreted as gravity suppressing the tendency of the rotating flow to develop radially, and the vortex band has a cylindrical shape with a relatively stable state. The minimum guide vane opening corresponds to the minimum flow in case 4. At this time, the rotating flow completely dominates, and gravity cannot suppress the rotating flow, so it is fully developed to the wall in the radial direction. The high flow velocity area is concentrated in the A3 region, and there are a few sporadic tiny vortex bands close to the draft tube wall. There is no definite law, and the hydraulic disturbance of the runner is not significant. When gravity and rotational flow of the water do not have a clear dominant effect, the two flow states ‘confront,' which intensifies the flow instability of the draft tube and accounts for the considerable amplitude of vibration in these two cases. The vortex band is currently in an unstable spiral configuration, and it is the primary source of the runner's low-frequency vibration. Disrupting the opposition of two fluid motions may be an approach to suppressing vortex band formation.
The differential pressure value p* and the dimensionless number Cp are introduced to characterize the transient pressure pulsation at each monitoring point to simplify the analysis:
(4)
(4)
(5)
(5) where pi is the measured pressure value of each time step, pave is the average of all pressure values, ρ is the density of water, g is the acceleration of gravity, and H is the water head of 120.65 m.
Fast Fourier variation is used to create the frequency domain signal for the pressure pulsation, and the horizontal coordinate is displayed in the spectrogram as a multiple of the runner's rotational frequency, while the vertical coordinate table is still represented by the dimensionless number Cp.
In order to obtain the pressure fluctuation characteristics of the fluid in the changing clearance channels, the spectral features of various clearance monitoring points were compared under four different operating conditions. According to Figure . In the crown clearance, in addition to the frequency components associated with the rotational speed transmitted from the main flow passage, particularly prominent low-frequency components were observed at monitoring points S4-S6. Through investigation, it was found that the vibration amplitude of the turbine is positively correlated with it. This correlation also exists in the lower labyrinth clearance, especially at the narrow labyrinth monitoring points. More low-frequency components appear at the lower labyrinth monitoring points due to the combined effects of the runner zone and the draft tube, leading to more complex pulsation components in this region.
3.2. Flow-induced vibration characteristics analysis in the pump-turbine runner under full load rejection condition
The simulation used the ‘Speed Changes during 100% Load Rejection Test' curve from the pumped storage unit's load rejection test report and the regulating guide vane closing law was listed in Table . Numerical simulations with time steps of 0.001 and 0.005 s were conducted to determine an appropriate time step in Figure (a). In order to adequately capture the flow characteristics for rotating machinery, that each time step the runner should be rotated at an Angle of less than 3 degrees. But after adding the clearance flow, the step size accuracy requirements are increased. Finally based on the simulations, a time step of 0.001 s was selected, which represents a runner rotation of 1.2 degrees, as it also resulted in the closest match to the experimental data and showed a secondary speed increase (At T1, the rotational speed of the unit reaches its maximum value. At T2, the rotational speed increases again, and at T3, it decreases once more). Using the chosen time step as the basis, the calculation was performed by incorporating the clearance displacement model. The variation in rotational speed was also compared, as shown in Figure (b). It was observed that the calculated results closely resembled those without the vibration model until the second increase in rotational speed. However, by introducing the vibration model, the hydraulic excitation force necessary for the second increase in rotational speed was adequately accounted for, resulting in a maximum speed that more closely matched the field test data, as indicated by the orange dashed line in the figure.
Figure 9. Comparison of rotational speed with field test data (a) Different time steps (b) With or without vibration displacement model.

Table 5. Closing rule of guide vanes.
Figure illustrates the schematic diagram of runner axis trajectory during load rejection transition. At the initial stage of the transition process. Under the action of hydraulic torque, the rotational speed of the turbine increases rapidly. During this process, the change in the inflow rate to the turbine causes unbalanced blade forces, which increases the turbine vibration. At T1, the rotational speed of the unit reaches the runaway speed, and the unit enters the braking state. The continuous closing of the guide vanes causes the flow angle at the inlet to change rapidly, resulting in a large amount of vortex flow inside the unit. Simultaneously, the vibration-induced water hammer and the squeezing of the fluid by the clearance induce hydraulic excitation, which results in an increase in the turbine vibration range. At T2, the unit returns to the operating state, and the internal flow gradually stabilizes, leading to a slight decrease in the vibration range, which tends to be stable. At T3, the flow rate has reached a low level, and the pump-turbine operates far from the rated condition. At this time, low-frequency vortexes in the draft tube cause an increase in the turbine vibration range. And we need to pay attention to the reasons and time points of the increased amplitude of runner vibration in the transition process, so as to provide reference ideas for the power station to optimize the guide vane closure strategy.
During the load rejection process, vibrations in the turbine unit are inevitable, but not all pressure fluctuations contribute to them. To investigate which pressure fluctuations have a significant impact on the vibrations, a correlation analysis was conducted between the pressure fluctuations and the hydraulic excitation forces that affect the turbine vibrations during the load rejection process. As illustrated in Figure . The data includes pressure pulsations in various components of the unit as well as the axial and radial forces influencing the turbine vibration. The absolute value of the correlation coefficient indicates the strength of the relationship between the two sets of data, with values closer to 1 indicating a stronger correlation. When studying turbine vibration, our primary concern is the factors influencing the radial force it experiences. It can be observed that the pressure pulsations in the labyrinth clearance between the crown, indicated by the red box, show the strongest correlation with the axial force among all the monitoring points. This highlights the significance of clearance vibration. Similarly, the draft tube pressure pulsation, being the data with the strongest correlation to the axial force, aligns with practical observations. Since the pressure pulsation at monitoring point S5 shows the strongest correlation with the radial force, the influence of the upper crown displacement on the correlation was further compared throughout the entire load rejection process. The results clearly indicate a significant increase in correlation when the crown exhibits displacement, thus emphasizing the reliability and necessity of considering the clearance displacement.
Figure 11. Correlation analysis plot of pressure fluctuations at monitoring points during load rejection process.

Due to the high correlation between clearance pulsations and turbine vibration, their characteristics were further investigated. By examining the three-dimensional time–frequency plots, it was observed that the inclusion of the vibration model led to the emergence of more low-frequency components in the pulsations at the clearance monitoring point, as indicated by the red loop in Figure (b). Comparing it with the draft tube monitoring point in Figure (c), it can be noted that there is a significant similarity in the low-frequency components between Figure (b) and Figure (c). This suggests that the pulsations in the draft tube primarily affect the axial force of the turbine, thus governing the vertical displacement of the wheel. Subsequently, the draft tube transmits this low-frequency pulsation to the clearance through the compression caused by the vertical displacement of the wheel. Therefore, the incorporation of the vibration model provides a more realistic representation of the interrelationship between clearance pressure pulsations and the main flow field of the turbine unit, resulting in more precise and reliable calculations.
4. Conclusions
The transient process of the pump-turbine is numerically simulated, the shift of runner clearance with runner vibration is realized using dynamic mesh and UDF techniques, and the reliability of the calculation method proposed in this paper is verified by comparison with field test results. The dynamic reaction of the water pump-turbine's runner and the features of the clearance flow field are investigated under various operating situations. The following are the key conclusions:
The radial force scatter distribution of the pump-turbine essentially exhibits circular or annulus behavior under various operating conditions for a while after stabilization. The more symmetrically distributed the radial force is along the central axes of X = 0 and Y = 0, the higher the distribution density, the smaller the vibration amplitude. The range of the shaft trajectory is initially large and subsequently small when the operating condition of the unit deviates greatly from the rated condition, and the vibration gradually veers toward disorder since the unit operating in the anti ‘S' characteristic region.
Three distinct high-speed swirling flow zones will manifest at the runner outlet under the assumption of constant speed when the opening of the guide vane is reduced. High-velocity cyclonic flow is usually found in the central circular area for high vibration conditions, and the spiral vortex band of the draft tube is responsible for the low-frequency vibration of the runner body. The runner force fluctuations become more unstable as a result of the low-frequency and high-amplitude vibration characteristics being transmitted into the clearance and then increased by vibration extrusion. Low-frequency pressure fluctuations in the upper cavity seal clearance are positively correlated with turbine vibration amplitude. In the band clearance, due to the hydraulic disturbances from both the non-blade region and the tailwater pipe region, the fluctuation components are more complex, leading to increased instability.
During the load rejection transition process, the pressure fluctuations with the strongest correlation to the unit vibration were observed at the crown clearance monitoring point. Furthermore, when the vibration displacement model was included in the simulation, it was found that the computed results of the secondary increase in rotational speed during load rejection were more accurate, and the correlation of clearance pressure fluctuations was enhanced. Additionally, the low-frequency fluctuations induced by the draft tube vortices caused the vertical vibration of the runner, resulting in the compression of the labyrinth band flow passage and the transmission of these low-frequency fluctuations to the clearance. This is a result that cannot be obtained without the vibration displacement model, thereby improving the computational accuracy of complex clearance flow analysis.
This study emphasizes the correlated effects of runner clearance vibration. However, there are still some limitations that need to be addressed: 1) The simplified dynamic equations consider the overall shaft system's impact on runner vibration; 2) The grid refinement in the clearance area needs improvement. In addition. The characteristics of the internal flow field after clearance vibration are not extensively analyzed in this paper, which is one of the directions for future research. Additionally, a more accurate dynamic model of the runner considering the shaft system remains a focus for future efforts.
Acknowledgments
The support of Hohai University and Wuhan University, China is also gratefully acknowledged.
Disclosure statement
No potential conflict of interest was reported by the author(s).
Additional information
Funding
References
- Chen, H. X., Zhou, D. Q., Kan, K., Guo, J. X., Zheng, Y., Binama, M., Xu, Z., & Feng, J. G. (2021). Transient characteristics during the co-closing guide vanes and runner blades of a bulb turbine in load rejection process. Renewable Energy, 165, 28–41. https://doi.org/10.1016/j.renene.2020.11.064
- Chitrakar, S., Dahlhaug, O. G., & Neopane, H. P. (2018). Numerical investigation of the effect of leakage flow through erosion-induced clearance gaps of guide vanes on the performance of Francis turbines [Article]. Engineering Applications of Computational Fluid Mechanics, 12(1), 662–678. https://doi.org/10.1080/19942060.2018.1509806
- Deng, W. Q., Xu, L. C., Li, Z., Tang, W., Wang, X. L., Shang, L. M., Liu, D. M., & Liu, X. B. (2022). Stability analysis of vaneless space in high-head pump-turbine under turbine mode: Computational fluid dynamics simulation and particle imaging velocimetry measurement [Article]. Machines, 10, 20, Article 143. https://doi.org/10.3390/machines10020143
- Elhami, M. R., Najafi, M. R., & Bafghi, M. T. (2021). Vibration analysis and numerical simulation of fluid-structure interaction phenomenon on a turbine blade [Article]. Journal of the Brazilian Society of Mechanical Sciences and Engineering, 43(5), 24, Article 245. https://doi.org/10.1007/s40430-021-02933-6
- Fu, X. L., Li, D. Y., Wang, H. J., Yang, J. L., & Wei, X. Z. (2022). Multi-objective optimization of guide vane closure scheme in clean pumped-storage power plant with emphasis on pressure fluctuations [Article]. Journal of Energy Storage, 55, 14, Article 105493. https://doi.org/10.1016/j.est.2022.105493
- Fu, X. L., Li, D. Y., Wang, H. J., Zhang, G. H., Li, Z. G., & Wei, X. Z. (2018). Influence of the clearance flow on the load rejection process in a pump-turbine. Renewable Energy, 127, 310–321. https://doi.org/10.1016/j.renene.2018.04.054
- Gong, J., Luo, W. Z., Wu, T. C., & Zhang, Z. Y. (2022). Numerical analysis of vortex and cavitation dynamics of an axial-flow pump [Article]. Engineering Applications of Computational Fluid Mechanics, 16(1), 1921–1938. https://doi.org/10.1080/19942060.2022.2122570
- Hou, X. X., Cheng, Y. G., Yang, Z. Y., Liu, K., Zhang, X. X., & Liu, D. M. (2021). Influence of clearance flow on dynamic hydraulic forces of pump-turbine during runaway transient process [Article]. Energies, 14, 20, Article 2830. https://doi.org/10.3390/en14102830
- Kan, K., Zhang, Q. Y., Xu, Z., Zheng, Y., Gao, Q., & Shen, L. (2022). Energy loss mechanism due to tip leakage flow of axial flow pump as turbine under various operating conditions [Article]. Energy, 255, 14, Article 124532. https://doi.org/10.1016/j.energy.2022.124532
- Kim, H. H., Rakibuzzaman, M., Kim, K., & Suh, S. H. (2019). Flow and fast Fourier transform analyses for Tip clearance effect in an operating Kaplan turbine. Energies, 12, 15. https://doi.org/10.3390/en12020264
- Li, D. Y., Duan, X. Q., Yue, N., Gong, R. Z., Wang, H. J., Qin, D. Q., & Wei, X. Z. (2022). Investigation on runner resonance and fatigue life of a high-head pump-turbine [Article]. Proceedings of the Institution of Mechanical Engineers Part C-Journal of Mechanical Engineering Science, 236(11), 5978–5993, Article 09544062211065371. https://doi.org/10.1177/09544062211065371
- Li, D. Y., Fu, X. L., Wang, H. J., Zhao, R. D., & Wei, X. Z. (2021). Evolution mechanism of a prototype pump turbine after pump power-off [Article]. Physics of Fluids, 33, 19, Article 106109. https://doi.org/10.1063/5.0065660
- Li, D. Y., Gong, R. Z., Wang, H. J., Xiang, G. M., Wei, X. Z., & Liu, Z. S. (2015). Dynamic analysis on pressure fluctuation in vaneless region of a pump turbine. Science China-Technological Sciences, 58(5), 813–824. https://doi.org/10.1007/s11431-014-5761-4
- Li, Z. J., Heng, X., Li, T. H., Wang, Y. J., & Wang, H. Y. (2018). Dynamic equation and response of turbine runner blade under flow excitation [Article]. Advances in Mechanical Engineering, 10, 9, Article 1687814018770509. https://doi.org/10.1177/1687814018770509
- Liang, Q. W., Kang, W. Z., Zhou, L. J., & Wang, Z. W. (2022). Numerical investigation of the flow regime in the vanes and the torsional self-excited vibration of guide vane in the pump mode of a reversible pump-turbine. Processes, 10, 1–13. https://doi.org/10.3390/pr10112314
- Liang, W. K., Huang, H. W., Wu, Z. J., Dong, W., Yan, X., & Liu, Y. Q. (2020). Numerical simulation of fluid-solid coupling of a Francis turbine with an upper crown cavity. Journal of Hydraulic Engineering, 51, 1383–1392+1400. https://doi.org/10.13243/j.cnki.slxb.20200211
- Liu, Y., Pan, L. T., Meng, P., & Wei, Z. P. (2021). Analysis of runner crack retrofit of Francis turbine based on fluid-solid coupling. Water Resources and Power, 39, 151–154+163.
- Liu, Y., Wang, D. Z., & Ran, H. J. (2021). Computational research on the formation mechanism of double humps in pump-turbines [Article]. Engineering Applications of Computational Fluid Mechanics, 15(1), 1542–1562. https://doi.org/10.1080/19942060.2021.1977711
- Liu, Y. B., Han, Y. D., Tan, L., & Wang, Y. M. (2020). Blade rotation angle on energy performance and tip leakage vortex in a mixed flow pump as turbine at pump mode [Article]. Energy, 206, 13, Article 118084. https://doi.org/10.1016/j.energy.2020.118084
- Liu, Y. B., & Tan, L. (2018). Tip clearance on pressure fluctuation intensity and vortex characteristic of a mixed flow pump as turbine at pump mode. Renewable Energy, 129, 606–615. https://doi.org/10.1016/j.renene.2018.06.032
- Nakhchi, M. E., & Rahmati, M. (2021). Direct numerical simulations of flutter instabilities over a vibrating turbine blade cascade. Journal of Fluids and Structures, 104, 18. https://doi.org/10.1016/j.jfluidstructs.2021.103324
- Nan, D., Shigemitsu, T., Ikebuchi, T., Ishiguro, T., & Hosotani, T. (2022). Pressure fluctuation on casing wall and investigation to tip leakage flow of contra-rotating small hydro-turbine [Article]. Proceedings of the Institution of Mechanical Engineers Part a-Journal of Power and Energy, 236(3), 506–518. Article 09576509211052734. https://doi.org/10.1177/09576509211052734
- Qu, B., Shen, Y. C., Lu, Y., Zhang, X., Shui, X. F., Zhu, M., & Wang, S. (2019). Fluid-Solid coupling calculation and analysis of new hydraulic turbine for cooling towers. Water Resources and Power, 37, 163–166.
- Sentyabov, A. V., Timoshevskiy, M. V., & Pervunin, K. S. (2019). Gap cavitation in the End clearance of a guide vane of a hydroturbine: Numerical and experimental investigation. Journal of Engineering Thermophysics, 28(1), 67–83. https://doi.org/10.1134/S1810232819010065
- Suh, J. W., Kim, S. J., Yang, H. M., Kim, M. S., Joo, W. G., Park, J., Kim, J. H., & Choi, Y. S. (2021). A comparative study of the scale effect on the S-shaped characteristics of a pump-turbine unit [Article]. Energies, 14, 29, Article 525. https://doi.org/10.3390/en14030525
- Trivedi, C., & Cervantes, M. J. (2017). Fluid-structure interactions in Francis turbines: A perspective review. Renewable & Sustainable Energy Reviews, 68, 87–101. https://doi.org/10.1016/j.rser.2016.09.121
- Trivedi, C., & Dahlhaug, O. G. (2018). Interaction between trailing edge wake and vortex rings in a Francis turbine at runaway condition: Compressible large eddy simulation [Article]. Physics of Fluids, 30(7), 15, Article 075101. https://doi.org/10.1063/1.5030867
- Waqas, M., & Ahmad, N. (2020). Computation of stress distribution in hydraulic horizontal propeller turbine runner based on fluid–structure interaction analysis. Arabian Journal for Science and Engineering (2011), 45(11), 9325–9337. https://doi.org/10.1007/s13369-020-04727-9
- Xu, L. C., Liu, D. M., Li, Z., Zhao, X. Y., & Liu, X. B. (2022). Experimental and numerical simulation research on flow characteristics of model pump-turbine in four-quadrant operating quadrants [Article]. Journal of Energy Storage, 54, 18, Article 105083. https://doi.org/10.1016/j.est.2022.105083
- Zhang, L. J., Yin, G. J., Wang, S., & Guan, C. N. (2020). Study on FSI analysis method of a large hydropower house and Its vortex-induced vibration regularities [Article]. Advances in Civil Engineering, 2020, 13, Article 7596080. https://doi.org/10.1155/2020/7596080
- Zhang, Z., Zhang, Y. Q., Zheng, Y., Zhang, J. S., Fernandez-Rodriguez, E., Zang, W., & Ji, R. W. (2023). Power fluctuation and wake characteristics of tidal stream turbine subjected to wave and current interaction [Article]. Energy, 264, 16, Article 126185. https://doi.org/10.1016/j.energy.2022.126185
- Zhao, H. R., Wang, F. J., Wang, C. Y., & Wang, B. H. (2022). Investigation on the hump region generation mechanism of pump mode in low-head pumped hydro-storage unit [Article]. Physics of Fluids, 34, 22, Article 115148. https://doi.org/10.1063/5.0130836
- Zhou, D. Q., Chen, H. X., Zhang, J., Jiang, S. W., Gui, J., Yang, C. X., & Yu, A. (2019). Numerical study on flow characteristics in a Francis turbine during load rejection [Article]. Energies, 12, 15, Article 716. https://doi.org/10.3390/en12040716
- Zhou, D. Q., & Chen, Y. (2015). Numerical simulation of clearance flow in Francis turbine with weep holes. Transactions of the Chinese Society for Agricultural Machinery, 46(04), 53–58. https://kns.cnki.net/kcms/detail/11.1964.s.20141209.0917.002.html.
- Zhou, X., Wu, H. G., & Su, K. (2019). Overview and discussion on hydraulic axial thrust in Francis turbine research. Journal of Hydraulic Engineering, 50, 1242–1252. https://doi.org/10.13243/j.cnki.slxb.20190325