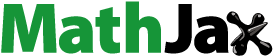
Abstract
In this paper, a double-focused conical inlet axisymmetric structure with double acceleration is proposed and the evolution of composite core-shell droplets is calculated using the volume of fluid (VOF) method, focusing on the size and droplet formation frequency of composite core-shell droplets as well as the quality control method. The results show that dripping and jetting regimes occur in the channel, and the generation period and flow distribution under the two mechanisms are investigated accordingly. The effect of local geometry on the evolution of composite droplets in the core-shell is investigated and analyzed using the composite droplet size, frequency, maximum extended length, and core droplet relative offset rate at the initial stage. It is found that changing the entrance angle can achieve the regulation of droplet size to a certain extent. With the increase of dimensionless width of the collection channel, the core-shell composite droplets size shows an overall increasing trend and is inversely proportional to the outer phase capillary number. Finally, a quality control method for composite droplet formation based on the maximum relative offset rate of core droplets is proposed for the purpose of controlling the integrity of composite droplets.
1. Introduction
Microfluidics (Oomen et al., Citation2016) is widely used in liquid-liquid extraction and droplet generation, etc. The application of microchannels to liquid-liquid extraction (Amini et al., Citation2023c) can improve the efficiency of mass transfer (Jafari et al., Citation2015) and reduce energy consumption on the one hand, and on the other hand extraction efficiency can be improved (Amini et al., Citation2023b). In terms of droplet generation, the technology can realize the high-throughput generation of single droplets (Jafari & Shamloo, Citation2023) and composite droplets. The generation of single droplets is relatively simple, but the shortcoming is that the risk of oxidative contamination of the substances inside the droplets can not be avoided, while the composite droplets can be a good solution to the problem due to their special structure. The shell fluid of composite droplets acts as a barrier separating the core fluid from the external matrix fluid, which can ensure the stability of the nuclear material during the delivery process, due to their structural excellence, composite droplets have a wide range of applications in related fields, which commonly include food science (Aditya et al., Citation2015), cosmetics (Yoshida et al., Citation1999), pharmaceuticals (Agarwal et al., Citation2013; Shao et al., Citation2021), encapsulation and additive manufacturing (Visser et al., Citation2018), among others. Composite microdroplets usually consist of a core (polymer) matrix and a (polymer) shell (Utada et al., Citation2005) with complex structures and small sizes (micrometer scale), and their compositions are roughly classified as O-W-O or W-O-W, including bilayered shell-core structured microdroplets (Nabavi et al., Citation2015; Nabavi et al., Citation2017b), multilayer composite-structured microdroplets (Abate & Weitz, Citation2009; Yu et al., Citation2021), Janus microdroplets (Yang et al., Citation2012), and multinuclear-structured microdroplets (Leister et al., Citation2022; Okushima et al., Citation2004), among others. In relevant applications, monodispersity and droplet size are the corresponding evaluation metrics, and excellent generation processes have high monodispersity and controllable droplet size. Therefore, it is crucial to investigate the composite droplet generation process.
Composite droplets can be generated in both active and passive ways (Zhu & Wang, Citation2016), and the traditional methods for composite droplet preparation are collision method (Kim & Wu, Citation2010), interfacial polymerization (Alexandridou et al., Citation2001), and spray drying (Guadarrama-Lezama et al., Citation2012), which have methods are inefficiently generated and less controllable. With the advancement of related scholars’ research, there are three common composite droplet generation devices, including the T-junctions structure (Wang et al., Citation2020), the flow-focusing structure (Chen et al., Citation2015), and the co-flowing structure (Zhang et al., Citation2021). In the T- junctions channel, three mutually immiscible liquids enter through three microchannels, and under the combined effect of surface tension and shear, the inner phase first breaks, then the intermediate phase wraps inward, and finally, under the force exerted by the outer phase, breakage occurs and a composite droplet is formed. Pan et al. (Citation2017) investigated the formation mechanism of S/W/O composite droplets in horizontal T-junctions devices, and additionally classified the flow mechanisms based on the continuous-phase capillary number and flow rate ratios, and explored each of the mechanisms in detail to clearly elucidate the composite droplet formation mechanism in the T-channel. Abate et al. (Citation2011) performed composite droplet production by means of a double-cross microfluidic structure, where highly controllable preparation was achieved by controlling the flow rate of each stream and thus the shell thickness of the composite droplets. Due to the decrease in the width of the flow-focusing aperture channel, the pressure in the part of the flow-focusing structure increases significantly, and the increase in squeezing pressure makes the composite fluid break rapidly, generating composite droplets. Compared to these two, the co-flowing structure is promising for the application of co-flowing devices in composite droplet generation due to its uniform external relative dispersed phase force as well as stability. Some scholars have carried out experimental studies on co-flowing structures. Pan et al. (Citation2020) experimentally realized the generation of W1/O/W2 compound droplets by using a co-flowing microfluidic device, and found the main influencing factors of the outer diameter of the composite droplets, and predicted the outer diameter of the droplets accordingly, and the results of the study provide experimental bases for the preparation of composite droplets. Leister et al. (Citation2022) applied a two-step jetting decomposition method in a co-flowing structure, which can realize the generation of multinuclear composite droplets, and the sizes of the inner and outer droplets can be independently controlled to meet diversified practical needs. Sattari et al. (Citation2021a) introduced an innovative microfluidic device for the one-step production of double emulsions, combining single-step and two-step methods, by which highly dispersible composite droplets can be prepared with high generation efficiency. Bandulasena et al. (Citation2019) designed a highly effective, reconfigurable, glass capillary microfluidic device that generates single and composite droplets, which produces micro/nanomaterials with controllable size and morphology.
In addition to experimental work, numerical simulation studies have been carried out by some scholars to reveal the fundamentals of the hydrodynamic behaviour in microfluidics. The current simulation methods mainly include the volume of fluid (VOF) method (Amini et al., Citation2016; Amini et al., Citation2023a), the lattice Boltzmann method (LBM) (Chen et al., Citation2022), the Phase-field method, etc., and the volume of fluid (VOF) method is the one that has been used most frequently in the simulation of the composite droplet generation process, and related scholars have used this method to conduct a series of research.
For example, Nabavi et al. (Citation2015) constructed a three-phase axisymmetric numerical model based on the volume of fluid-continuum surface force (VOF-CSF) model to investigate the flow state in a coaxial countercurrent focusing structure, predicting droplet and jetting distributions, and synthesizing and discussing the influencing factors of droplet size. Tan et al. (Citation2020) performed numerical simulations of composite droplet generation using a six-pass microfluidic device based on the volume of fluid (VOF) method and discussed the effects of the relevant parameters on the shell thickness, which was found to be strongly influenced by the intermediate fluid. Zhou et al. (Citation2022) investigated the generation of composite droplets in the designed channel by using the volume of fluid (VOF) method to analyze the variation of the flow distribution and the pressure field and investigate the deformation index of the droplets, which can help to understand the basic physical principles and the conditions for the generation of composite droplets. Sattari et al. (Citation2021b) used an axisymmetric approach to numerically simulate the generation of composite droplets and investigated the effects of flow rate, viscosity, and interfacial tension on the size of composite droplets using the response surface method. Vu et al. (Citation2013) investigated the rupture mode of immiscible fluids and studied the non-constant evolution and rupture of composite jets by a front-tracking/finite-difference approach, which helps to understand the rupture process. In contrast, the volume of fluid (VOF) method is more stable in the droplet generation process, and the corresponding numerical simulations were carried out by the droplet collisions and experiments done by Peng et al. (Citation2022a), and the results demonstrated a better agreement, which further verified the accurate reliability of the volume of fluid (VOF) method. Therefore, in this paper, the volume of fluid (VOF) method is used to numerically simulate the evolution of composite droplet generation and the corresponding fluid dynamics study.
Currently, studies on composite droplet generation mainly focus on the effects of factors such as fluid properties (Zhang et al., Citation2021) and fluid flow rate (Shao et al., Citation2013). The studied metrics mainly include the composite droplet diameter (Ong et al., Citation2007; Peng et al., Citation2022b), shell thickness (Tan et al., Citation2020), and flow distribution (Ge et al., Citation2021). It is found that there is a relative shift of the nuclear droplet relative to the shell droplet during the generation of composite droplets, and although a great deal of work has been carried out to study the evolutionary generation of composite droplets, there has been almost no study on the maximum relative shift rate of the core droplet and the shell droplet during the initial stage of composite droplet generation. The maximum relative offset rate between the core droplet and the shell droplet plays an important role in maintaining the integrity of the composite droplet after composite droplet generation, in addition to the imperfect influence on the mechanism of the flow distribution and the width of the collection channel during composite droplet generation.
In order to better guide the preparation of composite droplets, this paper investigates the controlled generation of composite droplets based on the volume of fluid (VOF) method. It is found that the generation process of composite droplets mainly consists of dripping and jetting, and is subdivided into six types according to the multiphase flow break length and flow state. Different geometrical (collection channel width W, inlet angle θ, flow-focusing orifice radius Rk, and length Ll) and physical parameters enable different degrees of modulation of the composite droplet size, generation frequency, break length, and relative offset rate. Finally, for the offset phenomenon of core droplets relative to shell droplets, a composite droplet quality control method is proposed, which realizes the control of the quality of composite droplets by adjusting the parameters and predicting the optimal collection pipeline length, thus realizing the controllable and high-quality preparation of composite droplets.
2. Model geometry and boundary conditions
2.1. Composite droplet preparation device model
Schematic diagram of the composite droplet generating micrometer piping device, as in Figure (a), which contains a total of three velocity inlets, of which the inner inlet and the middle inlet are conical constriction inlets. The internal inlet is used to flow the internal phase fluid (density ρ1, viscosity μ1), the intermediate inlet is used to flow the intermediate phase fluid (density ρ2, viscosity μ2), and the external inlet is used to flow the external phase fluid (density ρ3, viscosity μ3). Figure (a-a) shows a detailed view of the flow-focusing structure along with a schematic diagram showing the structure of a composite droplet, including both core and shell droplets. The geometrically calculated domain display for each part is shown in the geometry of the model in Figure (b), including the collection channel Lw with a length of 1650 µm, w is the width (diameter) of the collection channel, the width of the outer-phase inlet Lwo = 50 µm, the radius size of the flow-focusing channel Rk = 40µm, the length of the flow-focusing structure Ll = 50 µm, the height of the outer-phase inlet h = 230 µm, Ro is the radius of the shell droplets, Ri is the radius of the core droplet, and δ is the size of the shell thickness of the generated droplet, where U1, U2, and U3 are the magnitude of the velocities of the internal-phase fluid, intermediate-phase fluid, and outer-phase fluid at the inlet respectively, and they are constant velocities. Figure (c) shows the 3D structure of the generating device, and Figure shows the enlarged view at the inlet, with the inlet angles varying in the ranges of θf and θs, the inlet dimensions of ir1 = 15 µm and ir2 = 45-(ir1- ih1/tanθf), and the lengths of the inner-phase inlet and the intermediate-phase inlet in x-axis direction of ih1 = 5 µm and ih2 = 20 µm, respectively. Since the geometry of the device is symmetric about the x-axis, the upper half Figure (b-b) domain is chosen as the computational domain in order to reduce the overall computational effort. The channel width is characterized by the dimensionless width W* = W/ir1, the flow focusing structure is characterized by the dimensionless radius = Rk/2ir1 and the dimensionless length Ll* = Ll/ir1, and the length of the fluid neck is dimensionless as
= Lm/ir1. The ranges of variation of the local dimensions are displayed in Table , and the physical parameters for the individual parts are shown in Table .
Figure 1. (a) The main structure of the composite droplet generation device, including the flow focusing structure, and the structure of the composite droplets (shell droplets and core droplets); (b) The computational domain; (c) Schematic of the three-dimensional structure of the composite droplet generation device.

Table 1. Range of local dimensional changes.
Table 2. Physical properties and parameters of each part of the fluid.
In order to get more fluid downstream in a shorter time and to accelerate the molding of composite droplets, a conical inlet, and an inclined extrusion are used, where the inner and intermediate phases can obtain higher velocities on entry. In this process, the inclined wall acts as a flow aggregation guide, as shown in Figure . As the internal phase fluid enters the inlet region of the intermediate phase, the wall guiding action creates a downstream moving pressure on the intermediate phase, further increasing the velocity of the internal phase fluid. Meanwhile, when the inner phase enters at the same time as the middle, the multiphase fluid undergoes an acceleration, also known as the first acceleration, due to the squeezing effect of the inlet. The internal fluid phase and the intermediate fluid phase continue to move downstream under the action of the outer fluid phase. When entering the region of the flow-focusing structure, the squeezing action of the flow-focusing structure increases the pressure and increases the flow velocity of the fluid phase, which is referred to as the secondary acceleration in the flow process, which can improve the efficiency of the fluid to enter the downstream and shorten the time of droplet break generation.
2.2. Boundary conditions
The three-phase inlet with constant velocity inlet, no-slip condition at the near-wall, and axisymmetric boundary condition at the shaft can reduce the amount of simulation computation and improve computational efficiency. As shown in Figure (b), the outlet of this composite droplet generation structure is defined as the pressure outlet.
Capillary number equations: , and
, where μ, U, and σ are the viscosity, average velocity, and interfacial tension coefficient, respectively.
2.3. Case validation
In order to verify the accuracy of the computational methods used in this paper, numerical simulations were carried out using the experimental setup described in reference (Zeng et al., Citation2015), as shown in Figure . The analytical validation was carried out mainly at two flow rate ratios of 0.5 and 2.0. From the simulation results, it can be observed that the results of droplet generation are consistent with the experimental results and the generation is good. It can also be seen from Figure (c) that in both cases, the droplet sizes are similar in size and the errors are within acceptable limits. This further validates the accuracy of the method used in this paper and shows that it is capable of making reasonable predictions of droplet generation.
Figure 3. (a) Experimental images of T-channel droplet formation (Zeng et al., Citation2015); (b) corresponding numerical simulation images; (c) droplet lengths generated by experiment and simulation.

As shown in Figure , in order to further verify the accuracy of the numerical simulation method, the authors perform simulations of the droplet impact oscillation process and compare them with the experimental results of Qian and Law (Citation1997). It can be seen that the simulation results of the shape of the interface are in good agreement with the experimental results, which verifies the reliability of the model for predicting the evolutionary generation behaviour of composite droplets.
Figure 4. Current simulation results are validated against the experimental results of Qian and Law (Citation1997).

2.4. Grid independence verification
Four different grid sizes (Case A, Case B, Case C, and Case D) were tested based on quadrilateral mesh partitioning as shown in Figure . The grid size of Case A-D was increased from 1 × 10−3 mm to 4 × 10−3 mm in equal proportions, and the number of grids was reduced from 257,027 to 16,330. The data are counted for the length of the shell fluid under different time points, and it can be seen from Figure (a) that these curves almost overlap at the initial stage of generation with a high degree of consistency. With the gradual increase of the outer-phase extension length, the maximum break length is 1140.85 µm at the Case A grid number and the length of the break location is larger; at the Case B grid number, the maximum break length slightly decreases and is delayed. Under Case C and Case D, the maximum extension lengths were 963.864 and 981.424 µm, respectively, and the maximum break locations were almost the same, which had a small effect on the results by the number of grids in these two cases, whereas the difference in the maximum break lengths between Case C and Case A was 12%, with a relatively large error. In addition, as can be seen in Figure (b), there is a 2.6% difference in core droplet size and a 2.1% difference in shell droplet size between Cases C and D. The effect of the number of grids on the droplet size is small. The mesh size of Case C, i.e. the number of meshes is 28,571, was chosen on the basis of ensuring the appropriate maximum break length and the size of the composite droplets, taking into account the accuracy and economic efficiency of the calculations. Figure shows the grid division of Case C and the contour image during composite droplet generation, which shows the state of composite droplet generation more clearly.
2.5. Control equations
A separation algorithm based on unsteady flow pressure established based on the volume of fluid-continuum surface force (VOF-CSF) method (Hirt & Nichols, Citation1981) is used to solve the governing equations, the SIMPLE algorithm scheme is used to couple pressure and velocity, and the pressure equations are discretized using the PRESTO! algorithm. A second-order windward scheme is used to approximate the discretized momentum equations, and the phase interface is tracked by the Geo-Reconstruct method. Since the Reynolds number in this study is lower than 5, the fluid flow is laminar (Chen et al., Citation2015). After setting the parameters of each part of the three fluids in the simulation, the inlet region is initialized to reduce the computation time, and the generation of composite droplets is simulated at a suitable step size.
The governing equations for the three-phase flow throughout the computational domain are the continuity (Liu et al., Citation2018) and momentum (Amini et al., Citation2016; Liu et al., Citation2018) equations:
(1)
(1)
(2)
(2) where ρ,
, P, t and μ are density (kg/m3), velocity (m/s), pressure (Pa), time (s) and viscosity (
), respectively. Fg represents the force of gravity, which is negligible due to the micrometer scale of the channel (
).
is the surface tension (N/m3). The nature of each computational grid cell denotes pure or mixed phase, respectively, and here the three liquid phases are denoted by subscripts 1, 2, and 3, respectively, and the density ρ and viscosity μ of each cell are calculated as follows (Chen et al., Citation2015; Jafari et al., Citation2015):
(3)
(3)
(4)
(4) In the volume of fluid (VOF) model, there are three possible conditions for the volume fraction αi of each constituent phase in the cell:
αi = 0, no liquid in the cell;
0 < αi < 1, the cell contains an interface involving fluid i;
αi = 1, the cell is filled with liquid i, and the liquid–liquid interface is tracked by solving a continuous medium equation for αi.
The volume fractions of the inner, intermediate, and outer fluids throughout the computational region satisfy the following transport equations:
(5)
(5) where t is the time and
is the magnitude of the fluid velocity.
The principles followed for the volume fractions of the three components within each unit are as follows:
(6)
(6) The interfacial tension is calculated by the continuous surface tension (CSF) model (Brackbill et al., Citation1992):
(7)
(7) where the unit vector normal to the interface is the gradient of the volume fraction of the phase at the interface:
(8)
(8) where κ is the local interface curvature, which can be obtained by finding the dispersion of the unit normal to the phase interface:
(9)
(9) where
is:
(10)
(10)
3. Results and discussion
3.1. Flow regime
3.1.1. Dripping regime
As shown in Figure , the evolution of composite droplet generation at low external phase capillary number is demonstrated, with velocity streamlines on the left and pressure clouds on the right. At low outer fluid phase capillary counts, the multiphase fluid is slower as it extends downstream, and the common dripping state emerges (Zhang et al., Citation2021). As shown in Figure (a-1), the fluid velocity is higher at the inlet and is referred to as the first acceleration. After 0.5 ms, the multiphase fluid continues to grow downstream, while at 36 ms, the internal fluid phase is squeezed due to the shear resistance exerted by the intermediate fluid phase, leading to the fracture and generation of the core droplets as shown in Figure (a-2). The dominant effect of interfacial tension causes the velocity vectors to be in opposite directions, creating two velocity vortices. As shown in Figure (a-3), the core droplet moves further downstream by the intermediate fluid as time passes up to 44.5 ms. The composite droplet generation is further accelerated by an increase in the intermediate fluid velocity due to the squeezing effect of the fluid phase. At 45 ms, the intermediate fluid breaks, and a composite droplet is formed. Immediately after the break, the upstream intermediate fluid shrinks due to interfacial tension, creating a countercurrent at the front of the droplet, which results in the formation of the third vortex in the generation process (a-4), with smaller satellite droplets due to structural dominance.
Figure 7. Evolutionary formation process of droplet-like composite droplets, including an enlarged view of the local position of the evolutionary generation process (Cai = 0.027, Cam = 0.0078, Cao = 0.0027; U1:U2:U3 = 10:10:1; μ1:μ2:μ3 = 3.4:1:3.4; W* = 20; = 1.33;
= 3.33; θf/θs = 1).

According to Figure , it is possible to observe the generation cycle of the droplet generation mechanism core droplets and shell droplets. Figure (a) shows the variation of the generation cycle of the core droplets, the first cycle has a longer duration of 44 ms, while the second cycle has a duration of about 36 ms. Due to the continuous extension of the intermediate-phase and internal-phase fluids, the shearing effect of the intermediate fluid on the internal fluid is strengthened, thus accelerating the generation of core droplets. The generation time of the second cycle is reduced relative to the first cycle. In addition, the first-cycle droplets experience asynchronous breakage with respect to the intermediate fluid, in which the internal fluid exhibits a growth-breakage-growth cycle, while the broken droplets combine to form larger droplets downstream of the intermediate fluid. Figure (b) highlights two distinct fracture processes in the shell droplet. The maximum elongation of the intermediate fluid reaches 11.15 µm in the first stage, while the maximum elongation in the second cycle is about 9.83 µm, and the corresponding time plots clearly show the changes in the morphology of the composite droplet generation at each moment.
Figure 8. (a) Periodogram of droplet core droplet generation (b) Periodogram of droplet shell droplet generation (Cai = 0.027, Cam = 0.0078, Cao = 0.0027; U1:U2:U3 = 10:10:1; μ1:μ2:μ3 = 3.4:1:3.4; W* = 20; = 1.33;
= 3.33; θf/θs = 1).

As shown in Figure , the pressure variations on the axis at four typical moments during droplet composite droplet generation were mainly monitored. The x-axis is expressed as the ratio (dimensionless) of the distance travelled by the composite droplet along the axis of symmetry to the radius of the aperture of the flow-focusing structure (Rk = 20 µm), while the y-axis demonstrates the variation of the pressure value at the corresponding position. a, b, and c represent the variation of pressure in the x-axis for the core droplet, shell droplet, and outer fluid phase, respectively. The locally enlarged image shows that at 46 ms the corresponding initial pressure reaches a maximum value, which gradually decreases from 2000Pa to about 250 Pa as the multiphase fluid flows downstream. It is worth noting that at t = 184.5 ms, the composite droplets show a uniform arrangement with periodic variations, and the pressure values show a decrease as the composite droplets continue to flow downstream. The maximum pressure is concentrated in the centre of the composite droplet at about 300 Pa, with different pressure distributions between the core droplet, the shell droplet, and the external fluid, and this difference is caused by the additional pressure generated by the liquid-liquid interfacial tension, specifically, the core pressure is greater than the shell pressure, which in turn is greater than the outer fluid phase pressure.
3.1.2. Jetting regime
Figure demonstrates the composite droplet jetting generation mechanism for a flow rate ratio of U1: U2: U3 = 4:5:1 (Zhang et al., Citation2021). Droplet generation in the jetting state consists of growth, necking, and pinch-off phases (Yu et al., Citation2021). The upstream fluid starts to grow at t = 5 ms, which leads to the continuous extension of the composite jetting downstream with the continuous entry of the fluid and enters the squeezing necking stage at t = 9.5 ms, which shortens the processing time by 19.5 ms compared with Figure and completes the pinch-off process at the tail of the composite jetting at t = 10 ms due to the instability of the capillaries to generate the composite droplets. At the same time, the front part of the composite jetting shrinks due to interfacial tension, leading to the generation of backflow and the formation of a localized vortex. Figure (a-i) shows the generation process of the five droplets, and the average generation time is 4.7 ms, which has a high generation efficiency. In addition, from Figure (f) and (e), it can be seen that the internal-phase fluid and the intermediate-phase fluid show asynchronous extension, and the internal-phase fluid, in the presence of the intermediate-phase fluid, leads to the generation of core droplets earlier than the shell droplet generation, resulting in a faster composite droplet generation rate. The dynamic focusing effect of the conical inlet and the flow-focusing structure leads to relatively high pressure of the composite jetting inside the orifice, which accelerates the composite jetting, thus shortening the generation time of the composite droplet. As the outer fluid phase flows out of the flow-focusing structure the pressure difference causes the outer fluid phase to expand and vortex at the corners of the collection channel, and the vortex surface area as well as the intensity remains constant for the same number of outer phase capillaries.
Figure 10. Velocity streamlines and phase diagrams for the evolution and formation of composite droplets in the jetting state (Cai = 0.11, Cam = 0.039, Cao = 0.027; U1:U2:U3 = 4:5:1; μ1:μ2:μ3 = 3.4:1:3.4; W* = 20; = 1.33;
= 3.33; θf/θs = 1).

Figure 11. (a) Periodogram of core droplet generation in jetting state; (b) Periodogram of shell droplet generation in jetting state (Cai = 0.11, Cam = 0.039, Cao = 0.027; U1:U2:U3 = 4:5:1; μ1:μ2:μ3 = 3.4:1:3.4; W* = 20; = 1.33;
= 3.33; θf/θs = 1).

Figure (a) illustrates the variation of the generation cycles of the core droplets under the jetting generation mechanism. The duration of the first cycle is about 8 ms, which is characterized by two break-up processes, i.e. asynchronous extension relative to the intermediate-phase fluid, and the second and third cycles are about 5 and 4 ms, respectively. It can be observed that the maximum break length increases with the generation cycle, the maximum dimensionless break length (Bl* = Bl/ir1) reaches 18.13 in the third cycle, and the lengths of the phase fluids are almost the same after breaking in the second and third cycles. The increase in viscous forces of the outer-phase fluid at high flow velocities leads to a spontaneous transition from dripping to jetting, in which the dominant force changes from interfacial tension to viscous forces, generating composite droplets at the end of the stretched coaxial jetting (Yu et al., Citation2018). Since the viscous force is proportional to 1/Rk2, jetting is more likely to occur in flow-focused structures. Figure (b), on the other hand, demonstrates the two breakup processes of the shell droplet under the jetting generation mechanism, with the first cycle evolution time extended compared to the core droplet, which in combination with Figure (a) further corroborates the asynchronous extension of the composite jetting in the first cycle. The maximum dimensionless break length of the intermediate fluid in the first cycle reaches 24.96, which is improved by 55.33% compared to the dripping case, and the evolution time is improved by about 30 ms compared to the dripping case, while the maximum dimensionless break length in the second cycle reaches 25.6, which is improved by 61.6% compared to the dripping case, and the evolution time is improved by about 64 ms compared to the dripping case. Overall, the faster rate of composite droplet generation compared to the dropping case is clearly demonstrated in the contour illustrations, revealing the corresponding droplet generation patterns at each moment in time.
3.1.3. Division of flow regimes
Zhou et al. (Citation2022) mainly classified the generation of composite droplets into four flow regimes, including regular dripping, irregular dripping, synchronized jetting, and asynchronous jetting. Wang et al. (Citation2020) classified the composite droplet evolutionary generation into 11 different flow regimes, which has a more detailed classification compared to Zhou et al. (Citation2022). Unsynchronized short jetting, and synchronized long jetting are represented in the experiments done by Nabavi et al (Citation2017a, Figure 5), Shang et al. (Citation2014, Figure ), and Abate et al. (Citation2011), respectively, but the common problem is that the jetting mechanism has not been quantified. As shown in Figure , we classify the jetting into four jetting states: Synchronous or Asynchronous short jetting (17 < ≤ 29), Synchronous or Asynchronous long jetting (
> 29), based on the dimensionless maximum break neck length and combining the break synchronization states of the internal fluid phase and the intermediate fluid phase. The droplet state is reflected in experiments such as (Pan et al., Citation2020), (Chen et al., Citation2015), in this device the droplet has a certain neck length before breaking (Liu et al., Citation2017), which is different from the droplet state appearing in the experiments, in order to be more clear about the process of the generation of the droplet state under the device, based on the quantization of the dimensionless length, the droplet state is classified into short-necked drop states (0 <
≤ 11) and long-necked drop states (11 <
≤ 17). Compared with the research results of previous scholars, the flow mechanism in this paper is categorized more finely, which makes the generation process of composite droplets clearer. On this basis, the effect of capillary number on the flow mechanism is further investigated to explore a deeper phenomenon.
Figure 12. Three-dimensional modelling of dripping, short jetting and long jetting based on dimensionless extension lengths.

Figure shows the distribution of flow regimes for outer phase capillary numbers of 0.002,684 and 0.02684. Based on the dimensionless value of the final break length of the neck, the generation states are mainly classified into six modes, including short-necked dripping (Snd), long-necked dripping (Lnd) in the drop state, and asynchronous short jetting (Asj), asynchronous long jetting (Alj), synchronous short jetting (Ssj), and synchronous long jetting (Slj) in the jetting state. With a capillary number of 0.02684 for the outer fluid phase, the outer fluid provides a large inertial force, and the inner and intermediate fluid phases are rapidly separated from the inlet and extend downstream, mainly in the form of jetting. However, in the case of an external phase capillary number of 0.002684, the internal and intermediate fluids could not be pushed away from the union due to the low inertial force and mainly showed a dripping state. The dripping state is mainly distributed in the region where the capillary number of the intermediate phase is 0.06 or less, whereas the synchronized jetting mainly appears in the region where the capillary number of the inner and intermediate phases is larger.
3.2. Effects of local geometry
3.2.1. Dimensionless width of collection channel W*
According to Liu et al. (Citation2017), the effect of different structural parameters (flow-focused structural aperture, etc.) on the composite droplet generation process has been explored. However, the effect of the collection channel width w has been rarely addressed, and based on this, we have studied this element accordingly. Figure illustrates the composite droplet generation process at six dimensionless collection channel widths. The images at each channel size include the maximum elongation length lmax of the first droplet before breakage, the just-breakage process, the image of the composite droplet within 5 ms after breakage, and the image of the second composite droplet after breakage. The dimensionless diameter of the generated composite droplet core droplet is = (a + b)/2ir1 and the dimensionless diameter of the shell droplet is
= (c + d)/2ir1. The size of the composite droplets gradually increases as the dimensionless channel width increases. In the case of W* = 40 channels, the composite droplet generation frequency is 21.28 Hz, which is slower and has a larger droplet size. In contrast, the composite droplets are generated more efficiently in a narrow channel with W* = 20, with a generation frequency of 250 Hz. 11 ms for the first droplet generation and 15 ms for the second composite droplet generation, which is accelerated by the reduction of the dimensionless channel width, which strengthens the shear force in the channel and accelerates the fracture generation of droplets. In the case of W* = 34 and W* = 37.4, the first droplet is poorly formed and the roundness of the outer droplet is not as good as that under several other channels. Macroscopically, it can be seen that the droplet generation process is good and the generation morphology is better when the dimensionless channel width is between 20 and 30.67.
Figure 14. Composite droplet generation process for dimensionless collection channel widths W* of 20; 24; 30.67; 34; 37.4 and 40 (Cai = 0.11, Cam = 0.039, Cao = 0.027; U1:U2:U3 = 4:5:1; μ1:μ2:μ3 = 3.4:1:3.4; = 1.33;
= 3.33; θf/θs = 1).

The low relative offset rate is crucial for the stability of the composite droplets during the collection process. As shown in Figure , to ensure the integrity of the generated composite droplets, the offset rate of the core droplets relative to the shell droplets at the initial stage was investigated in conjunction with the dimensionless collection channel width W* as well as the flow rate ratio. The two-dimensional schematic of the composite droplets in which l is the core-shell boundary distance and the core-shell relative offset rate is the ratio of the relative displacement to time. when U2/U3 = 2.0, the high outer-phase flow rate leads to the rapid generation of the composite droplets, and the core droplets have a smaller velocity relative to the shell droplets, which presents a low relative offset rate at different collection channel widths W*. when W* = 24, the effect of the flow rate ratio on the relative offset rate decreases accordingly. The maximum relative offset rate is 30.88 mm/s for W* = 30.67 and U2/U3 = 3.3. The minimum relative offset rate is 4.37 mm/s for W* = 34 and U2/U3 = 2.0. At W* = 37.4, the offset rate of the small flow rate ratio and large flow rate ratio pulls a certain gap, and the large flow rate ratio is not favourable for the stabilization of composite droplets at this channel width. At U2/U3 = 5.0, large relative offset rates were observed at different collection channel widths W*, which were not favourable for the stability of composite droplets. By studying the offset rates of composite droplets at different channel widths and different rates, a more optimal criterion for the width of the collection channel can be determined and a suitable flow rate ratio can be selected under this criterion, thus optimizing the preparation process of composite droplets.
Figure 15. Variation of the relative offset rates of core and shell droplets at the initial stage of composite droplet fracture generation under different dimensionless collection channel widths W* and flow rates (Cai = 0.11, Cam = 0.039, Cao = 0.027; μ1:μ2:μ3 = 3.4:1:3.4; = 1.33;
= 3.33; θf/θs = 1).

Figure (a-f) corresponds to the generation of composite droplets for dimensionless channel widths W* of 20; 24; 30.67; 34; 37.4 and 40, respectively, and the generated sizes of the core droplets and shell droplets increase with the increase of W*. From Figure (a-f), it can be roughly obtained that the composite droplet size is inversely proportional to Cao, which leads to a consequent decrease in the size of the composite droplet due to the viscous force exerted by the outer-phase fluid that increases with the increase of Cao (Zhang et al., Citation2021). Specifically, the core droplet and shell droplet size decrease rates are 71% and 72%, respectively, for W* = 40, while the core droplet and shell droplet size decrease rates are 42% and 43%, respectively, for W* = 24, which suggests that the composite droplet sizes are more affected by Cao when W* is larger. The shell thickness δ is inversely proportional to Cao, the generation frequency f increases with increasing Cao, and the composite droplet size is inversely proportional to the generation frequency f. The composite droplet generation frequency is maximum at 2.0 kHz for W* = 20 and Cao = 0.0671. It is found from the analysis that the composite droplet generation frequency is highest when the dimensionless channel width is W* = 20 and the generation is stable and excellent. The size-frequency relationship plot can show the effect of channel width on droplet generation more clearly for composite droplet generation of different sizes.
Figure 16. (a-f) Core-shell droplet diameter, shell thickness, and generation frequency as a function of the number of outer-phase capillaries for dimensionless collection channel widths W* of 20; 24; 30.67; 34; 37.4; and 40, respectively (Cai = 0.11, Cam = 0.039, Cao = 0.027; U1:U2:U3 = 4:5:1; μ1:μ2:μ3 = 3.4:1:3.4; = 1.33;
= 3.33; θf/θs = 1).

3.2.2. Entrance angles
Figure illustrates the effect of different combinations of internal and intermediate fluid phase inlet angles θf and θs (see Figure ) on composite droplet generation. Figure (a) shows a numerical simulation of composite droplet generation, where the angle of the inlet is zoomed in to compare with the later data plots. Figure (b) shows the variation of dimensionless neck fracture length with the variation of intermediate phase inlet angle θs for inner phase inlet angles θf of 30°, 60°, 80°, 90° and 120°. At θf = 120° and θs = 60° in Figure (c), the core droplet diameter reaches a maximum due to more internal phase fluid entering the channel at the same time. At θs ≤ 60°, the range of variation of core droplet size is large, and as θs increases the range of variation of droplet size decreases, eventually showing an almost constant trend. The variation of shell droplet diameters in Figure (d) shows a similar pattern, with a smaller range of shell droplet size variations when θs is small. The inlet combination of θf = 120° and θs = 60° produces a larger shell droplet diameter with a of 14.55. On the contrary, the inlet angle combination of θf = 80° and θs = 30° produces a smaller shell droplet diameter with a
of 13.11. This indicates that the preparation of composite droplets of different sizes can be accommodated by different combinations of device inlet angles.
3.2.3. Flow-focusing structure
Figure demonstrates the effect of the length of the dimensionless flow-focusing structure on the composite droplet formation process for U1:U2:U3 = 4:5:1. From Figure (a), it can be seen that both the velocity and pressure are larger at the flow-focusing structure, and the neck break length
of the multiphase flow increases gradually with the increase of
, and
reaches a maximum value of 71.184 at
= 9.3. Figure (c) is a zoomed-in view of the velocity cloud at the flow-focusing structure, where it can be seen that the high flow velocity region extends further with the length of the flow-focusing structure, leading to an increase in the acceleration region. Vortex generation can be observed at the rectangular corners and at the droplet break location, similar to the vortices generated in the previous flow analysis. Combined with the composite droplet generation frequency plot in Figure (b), it can be found that the generation frequency of composite droplets gradually increases in the length range of the flow-focusing structure between
= 3.33–6.67 and the frequency reaches a maximum value of 0.222 at
= 6.67. which indicates that a small elongation of the extension length can accelerate the generation of composite droplets. However, exceeding a certain extension length leads to a decrease in the generation frequency. Therefore, controlling a certain range of flow-focusing structure lengths at a certain fluid phase rate is important for the efficiency of composite droplet generation. Figure (a) The small range of variation in the dimensionless diameter of the core-shell droplets suggests that the length of the flow-focusing structure has a small effect on the size of the composite droplets (Liu et al., Citation2017). The elongation length of the jetting is shown in Figure to increase with the length of the flow-focusing structure, and there is a positive relationship between longer flow-focusing structures corresponding to longer neck break lengths Bl* at the same moment. This demonstrates more fully the effect of variations in the length of flow-focused structures on the generation of composite droplets and complements the work of previous scholars.
Figure 18. Velocity field and pressure field changes after changing the length of the flow-focusing structure (Cai = 0.11, Cam = 0.039, Cao = 0.027; U1:U2:U3 = 4:5:1; μ1:μ2:μ3 = 3.4:1:3.4; W* = 20; = 1.33; θf/θs = 1).

Figure 19. (a) Variation of composite droplet size as well as shell thickness for different flow-focusing structure lengths; (b) Length and frequency of neck breakage during composite droplet generation for different flow-focusing structure lengths (Cai = 0.11, Cam = 0.039, Cao = 0.027; U1:U2:U3 = 4:5:1; μ1:μ2:μ3 = 3.4:1:3.4; W* = 20; = 1.33; θf/θs = 1).

Figure demonstrates the effect of the dimensionless orifice radius of the flow-focusing structure on the composite droplet generation process. As can be observed from Figure (b), the evolutionary generation of core-shell composite droplets is influenced by the radius of the dimensionless flow-focusing orifice
. When
is small, the focusing effect is enhanced, the local flow resistance increases, the extension length of the multiphase flow in the collection pipe increases, the neck break location is farther away from the orifice, and, at the same time, a larger pressure drop is generated in the flow focusing structure. In addition, two symmetric vortices are visible on both sides of the exit of the flow-focusing orifice, and the vortex region decreases with increasing
, and the vortex disappears when
= 3.33 (Wu et al., Citation2017). As shown in Figure (a), the variation of the neck break position affects the size of the core-shell composite droplets, and the size of the core droplets has the same pattern as that of the shell droplets, i.e. it increases and then decreases, and then remains steady. Figure (b) shows that the production frequency of core-shell composite droplets does not rise or fall monotonically. The generation frequency of composite droplets reaches 0.25 kHz at
= 2 when the size of composite droplets is more stable. The generation frequency is minimized at
= 2.67 when the droplet size is the largest, and the composite droplet generation frequency varies less as
continues to increase at
≥ 3.33. Therefore, the appropriate size of the flow-focusing orifice plays an important role in the efficient and controllable generation of composite droplets.
Figure 20. Effect of orifice size of flow focusing structure on velocity and pressure fields (Cai = 0.11, Cam = 0.039, Cao = 0.027; U1:U2:U3 = 4:5:1; μ1:μ2:μ3 = 3.4:1:3.4; W* = 20; = 3.33; θf/θs = 1).

Figure 21. (a) Variation of composite droplet size as well as shell thickness for different flow-focusing structure apertures; (b) Variation of maximum neck break length as well as generating frequency relationship for different flow-focusing structure orifice (Cai = 0.11, Cam = 0.039, Cao = 0.027; U1:U2:U3 = 4:5:1; μ1:μ2:μ3 = 3.4:1:3.4; W* = 20; = 3.33; θf/θs = 1).

3.3. Influence of physical property parameters
3.3.1. Diameter and shell xthickness
Figure (a) demonstrates the effect of the flow rate ratio (U1 = 0.03 m/s) on the size of the composite droplet generation and the shell thickness δ. At U1/U2 = 0.3, has a maximum value of 11.74, with the increase of U1/U2, the flow rate of the intermediate phase into the flow-focusing structure decreases and
gradually decreases, and
reaches a minimum value of 8.14 at U1/U2 = 1.0. The shell thickness δ reaches its maximum value at U1/U2 = 0.3, and the relative flow rate of the fluid in the intermediate phase decreases, leading to a gradual decrease in
, δ, and
showing a similar decreasing trend. The change of flow rate ratio is accompanied by the change of flow pattern, and when U1/U2 < 0.43, as the break length of the composite droplet, keeps lengthening with the increase of U2, it leads to the core droplet leaving the pipe outlet earlier than the shell droplet, and the asynchronous long jetting occurs. The intermediate-phase inertial force decreases gradually with the increase of U1/U2, and the break length undergoes a certain degree of shortening, and there is a transition from a long jetting to a short jetting. Figure (b) demonstrates the effect of interfacial tension ratio (σ23 = 0.005 N/m for σ12/σ23 ≤ 0.8 and σ12 = 0.005 N/m for σ12/σ23 > 0.8) on the composite droplet generation. Unlike the flow rate ratio case, the core droplet
and shell droplet
show similar patterns of change, and the core droplet
and shell droplet
increase with the increase of σ12 for σ12/σ23 ≤ 0.6. Figure (c) demonstrates the effect of density ratio (ρ1 = 1000 kg/m3) on the composite droplet generation size and shell thickness δ. Similar to the effect of interfacial tension ratio (σ12/σ23),
and
increase synchronously for ρ1/ρ2 ≤ 0.83, and δ tends to increase with the density ratio (ρ1/ρ2), and on the whole, the density of the intermediate phase has a small effect on the composite droplet generation. Figure (d) demonstrates the effect of viscosity ratio. At different viscosity ratios (μ1 = 0.00671 Pa·s), the effect of μ2 on droplet size as well as shell thickness is less, the generated droplet size is more homogeneous, and the quality of composite droplet generation is higher. In addition, it was found that the flow rate ratio had the greatest modulation on the composite droplet size, with a drop rate of 30.6% for
and 27.2% for
, and the viscosity ratio had the least modulation on the composite droplet size.
Figure 22. (a)–(d) shows the variation of the flow rate ratio of the inner phase to the intermediate phase, the interfacial tension, the density ratio, and the viscosity ratio as a function of the composite droplet size and the shell thickness respectively. (W* = 20; = 1.33;
= 3.33; U1:U2:U3 = 4:5:1; θf/θs = 1).

3.3.2. Composite generation frequency and initial offset rate
Figure shows the effect of physical property parameters on the composite droplet generation frequency f as well as the relative offset rate. From Figure (a)–(d), it can be seen that the larger relative offset rates are mainly concentrated in the first half, while the larger generation frequency f is mainly concentrated in the second half. When U1/U2 ≤ 0.43, due to the high inertia when the intermediate phase breaks, the relative offset rate is increasing, and as the flow rate ratio (U1 = 0.03 m/s) continues to increase, the inertia force when the intermediate phase breaks gradually decreases, resulting in a corresponding decrease in the relative offset rate, and reaches a minimum of 12.44 mm/s. The composite droplet generation frequency increases slowly with the increase of U1/U2 in the initial stage, and as U1/U2 continues to increase, the extension length of the composite droplet generation decreases due to the decrease of the inflow of the intermediate-phase fluid to the extent that the generation frequency increases rapidly. The maximum relative offset rate between core and shell droplets is 22.196 mm/s for different interfacial tension ratios (σ23 = 0.005 N/m for σ12/σ23 ≤ 0.8; σ12 = 0.005 for σ12/σ23>0.8), which gradually decreases with the increase of σ12/σ23. Smaller relative offset rates play an important role in maintaining the integrity of the composite droplet and avoiding premature breakup of the composite droplet. The minimum relative excursion rates achieved for different viscosity ratios (μ1 = 0.00671 Pa·s) indicate that a suitable choice of viscosity between the internal and outer fluid phases has a positive effect on controlling the mass of the composite droplets. From Figure OLE_LINKFIG23(a), (b), and (d), it can be observed that higher composite droplet generation frequency corresponds to a lower relative offset rate of core-shell droplets, showing a certain regularity. However, the case in Figure (c) is different in that the maximum generation frequency does not correspond to the minimum offset rate. The interfacial tension ratio (σ12/σ23) has the greatest effect on the relative offset rate with a difference of 17.5545 between the maximum and minimum values, and the flow rate ratio (U1/U2) has the least effect on the relative offset rate with a difference of 8.8505 between the maximum and minimum values. In the composite droplet preparation process, a high-frequency low offset rate is the preferred case. Appropriate selection of physical property parameters can control the homogeneity of the composite droplet size on the one hand to realize the controllable preparation; on the other hand, it also has a certain influence on the offset rate to ensure that the prepared composite droplets have complete quality.
Figure 23. (a)–(d) shows the variation of internal and intermediate phase flow rate ratios, interfacial tension ratio, density, and viscosity ratio as a function of the composite droplet evolution generation frequency and the core droplet offset rate at the initial stage of generation, respectively (W* = 20; = 1.33;
= 3.33; U1:U2:U3 = 4:5:1; θf/θs = 1).

3.4. Composite droplet quality control
As shown in Figure , a schematic diagram of the profile generating the composite droplet offset rupture process is demonstrated. Focusing on ensuring the integrity of the collected composite droplets, based on the previous simulation results and the research of related scholars (Vu et al., Citation2013; Zhou et al., Citation2022) , we found that the core droplets are offset relative to the shell droplets, and the relative velocities of the core droplets and the shell droplets reach the maximum when the composite droplets are just fracture-generated (the velocity of the core droplets is larger). Therefore, the offset distance of the core droplet is larger in the initial fracture stage, and as the composite droplet continues to move downstream, the relative velocity between the core droplet and the shell droplet gradually decreases, and it is a variable speed motion.
Figure 24. Schematic diagram of the profile of the offset rupture process for generating composite droplets.

In this process, two scenarios can occur: one is that the core droplet reaches the same velocity as the shell droplet before the shell droplet breaks up and continues to move downstream at that velocity, thus maintaining the integrity of the composite droplet; the other scenario is that the composite droplet breaks up before the core droplet reaches the same velocity as the shell droplet, resulting in a reduction in its quality. In order to determine the optimal collection channel length (both economically as well as to control the high quality of collected composite droplets), we assumed that the relative velocity of the composite droplet core droplet to the shell droplet is constant at the high speed just at the time of rupture (i.e. without taking into account the decrease of the relative velocity behind it), and determined the predicted critical time for the rupture of the shell droplet at this maximum velocity (which was predicted earlier than the actual time of rupture, which can guarantee the quality of the composite droplet in the actual case), so that we could calculate the predicted critical distance for the movement of composite droplets (which is less than the actual critical distance, which can ensure the quality of the composite droplet in the actual case). The collection channel length calculated in this case is the optimal collection channel length, which on the one hand can control the quality of the composite droplets and can ensure that the composite droplets can be collected before the composite droplets break up, and on the other hand can reduce the length of the collection channel within a suitable range, which is economical.
Prediction of the optimal collection channel length, Lw, based on the maximum relative offset rate between core and shell droplets. The distance moved by the core droplet in t (s) in Figure is LN, which gives the velocity of the core droplet as VN = LN/t. Similarly, the velocity of the shell droplet in t (s) is VS = LS/t. This gives the relative rate of movement of the core droplet and the shell droplet in just breaking t (s) as V = VN-VS. If the distance from the right boundary of the core droplet to the right boundary of the shell droplet at the initial moment is L0, the predicted critical time for the rupture of the shell droplet as the composite droplet continues to move downstream is t* = L0/V (where the relative velocity is kept constant at the high speed of the initial stage). Combining the shell droplet velocity and the predicted critical breakup time yields the maximum distance along the collection channel travelled by the composite droplet breakup front (predicted critical distance) L* = t* × VS.
If the composite droplet generation mechanism is dripping, the predicted critical channel length is Lw ≈ L* before composite droplet rupture; If the composite droplet generation mechanism is jetting, the critical pipe length before the composite droplet rupture is the sum of the maximum distance the composite droplet moves along the collection channel before the rupture (predicted critical distance) and the length of the jetting in the collection channel, i.e. Lw ≈ L* + Ljet. The integrity of the composite droplets is well ensured at this predicted collection channel length, ensuring the generation quality of the collected composite droplets.
Based on the principles mentioned above we investigate the critical collection pipe length Lw in order to ensure the quality of the resulting composite droplets and predict it according to different conditions.
3.4.1. Local geometry
It has been previously known that the predicted collection channel length is related to the maximum relative excursion rate of the core-shell droplets in the initial phase and the maximum elongation of the jetting in the collection channel. Figure (a) shows the dimensionless collection channel width, W*, as a function of the predicted collection channel length, which satisfies the equation Lw = 1287–0.144W*, and the average error between the resulting predicted value and the true measured value is within 5%, which is within acceptable accuracy. Figure (b) shows the inlet angle ratio as a function of the predicted collection channel length. As the angle ratio increases, the predicted collection channel length decreases, and after fitting, it can be obtained to satisfy Lw = 1158.4 × (θf/θs)−0.05 with a small average error. Figure shows that as the length of the flow focusing structure is extended, the extension length of the multiphase flow in the collection channel increases accordingly, but the increase is small. As a result, a similar pattern of proportional increase to the flow focusing length is not presented in Figure (c), which predicts a small upward and downward fluctuation between the collection channel lengths, satisfying the equation Lw = 1226–1.8. In Figure (d), the effect of the dimensionless aperture of the convective focusing structure on the predicted collection channel length is investigated. The predicted collection channel length has a similar trend to the jetting length (Figure ), i.e. the collection channel length decreases as the dimensionless radius increases. In this case, the effect of the maximum relative offset rate suffered is less than that of the jetting length. The predicted collection channel length satisfies the function Lw = 1402(
)−2.06 with an average error of about 1%, which is accurate. Predicting the collection channel length by changing the local geometry can provide an optimal reference value for the collection channel length during device design. A collection channel length near the predicted value can reduce the collection channel length while maintaining the quality of the core-shell composite droplets, ensuring economic efficiency. In addition, this can also reduce losses by avoiding breakage of composite droplets due to relative offset.
3.4.2. Physical parameters
Figure illustrates the physical property parameters as a function of the predicted collection channel length. Figure (a) shows the effect of the ratio of the velocities of the internal fluid phase to the intermediate fluid phase on the optimally predicted collection channel length. The collection channel length is predicted to be larger when the flow rate ratio is smaller, which means that the volume of intermediate-phase fluid entering the collection channel in the same amount of time increases, which in turn extends the length of the jetting in the collection channel. At this time, the length of the jetting in the collection channel has a dominant position, while the relative offset rate of the core droplets and shell droplets has less influence, with the increase of the flow rate ratio of the length of the collection channel has a tendency to decrease and to meet the prediction formula Lw = 982.77 × (U1/U2)−0.017, with an average error of about 4.3%, and the accuracy is within the acceptable range. Figure (b–d) shows the interfacial tension ratio, density ratio of the inner and intermediate phases, and viscosity ratio as a function of the predicted collection channel length, respectively. As the interfacial tension coefficient ratio and density ratio increase, the collection channel length gradually increases, in the predicted collection channel length reaches a maximum value of 1329.83 µm at a density ratio of 1.0. Figure (b) and (c) satisfy the prediction equations Lw = 1486.95 × (σ12/σ23)0.093 and Lw = 1214.02 × (ρ1/ρ2)0.032, respectively, with an average error of 5%. Figure (d) is predicted more accurately under the trigonometric function with an average error of 1%, which is highly accurate, and its predicted value can be used as a reference value for the design of the collection channel. By discussing the relationship between the physical property parameters and the predicted optimal collection channel Lw length, the method of quality control of composite droplets is improved, which plays an important role in selecting the appropriate physical property parameters and designing the appropriate length of the collection channel when carrying out the preparation of composite droplets.
4. Conclusion
In this paper, the numerical simulation of the composite droplet generation process in the controlled generation device of core-shell composite droplets in an axisymmetric conical inlet double-focusing microchannel is carried out based on the VOF method, and the evolution process of the composite droplets and the influence of the relevant parameters as well as the quality control method of the composite droplets are comprehensively discussed. The results show that:
Refinement of previous studies (Zhang et al., Citation2021) based on the functional relationship of Ca. The two mechanisms, dripping and jetting, are refined into six generative states, with asynchronous short jetting mainly occurring when Cao = 0.002684 and Cam ≥ 0.06, and the rest mainly occurring in the form of dripping. Asynchronous long jetting and synchronous long jetting mainly occur when Cao = 0.02684 and Cam ≥ 0.05, and short jetting mainly occurs when Cam < 0.05.
Reducing the collection channel diameter W can improve the composite droplet generation efficiency, while the collection channel diameter W and the flow rate ratio affect the relative offset rate of the core droplets. The inlet angle θ as well as Cao can realize the regulation of composite droplet size. The length of the flow-focusing structure Ll and the orifice diameter Rk mainly affect the generation efficiency of the composite droplets and have less influence on the composite droplet size.
The effects of physical property parameters on the composite droplet diameter D and shell thickness δ are summarized. Meanwhile, focusing on the relationship between the physical property parameters and the offset rate, the high-frequency low offset state, which plays an important role in the composite droplet preparation, this part can provide a guiding significance for the determination of the physical property parameters.
A quality control method for composite droplet generation based on core-shell composite droplets with a maximum relative offset rate between core and shell droplets is proposed to control the integrity of the generated composite droplets and to predict the optimal collection channel length in conjunction with the cost-effectiveness.
In summary, this study of composite droplet preparation and generation based on double focusing accelerated axisymmetric conical inlet channel provides important theoretical guidance and an experimental basis for the controllable and high-quality generation of composite droplets.
Disclosure statement
No potential conflict of interest was reported by the author(s).
Additional information
Funding
References
- Abate, A. R., Thiele, J., & Weitz, D. A. (2011). One-step formation of multiple emulsions in microfluidics. Lab on a Chip, 11(2), 253–258. https://doi.org/10.1039/c0lc00236d
- Abate, A. R., & Weitz, D. A. (2009). High-order multiple emulsions formed in poly(dimethylsiloxane) microfluidics. Small, 5(18), 2030–2032. https://doi.org/10.1002/smll.200900569
- Aditya, N. P., Aditya, S., Yang, H., Kim, H. W., Park, S. O., & Ko, S. (2015). Co-delivery of hydrophobic curcumin and hydrophilic catechin by a water-in-oil-in-water double emulsion. Food Chemistry, 173, 7–13. https://doi.org/10.1016/j.foodchem.2014.09.131
- Agarwal, P., Zhao, S., Bielecki, P., Rao, W., Choi, J. K., Zhao, Y., Yu, J., Zhang, W., & He, X. (2013). One-step microfluidic generation of pre-hatching embryo-like core-shell microcapsules for miniaturized 3D culture of pluripotent stem cells. Lab on A Chip, 13(23), 4525–4533. https://doi.org/10.1039/c3lc50678a
- Alexandridou, S., Kiparissides, C., Mange, F., & Foissy, A. (2001). Surface characterization of oil-containing polyterephthalamide microcapsules prepared by interfacial polymerization. Journal of microencapsulation, 18(6), 767–781. https://doi.org/10.1080/02652040110055243
- Amini, Y., Ghazanfari, V., Heydari, M., Shadman, M. M., Khamseh, A. G., Khani, M. H., & Hassanvand, A. (2023a). Computational fluid dynamics simulation of two-phase flow patterns in a serpentine microfluidic device. Scientific Reports, 13(1), 9483. http://doi.org/10.1038/s41598-023-36672-6
- Amini, Y., Hassanvand, A., Ghazanfari, V., Shadman, M. M., Heydari, M., & Alborzi, Z. S. (2023b). Optimization of liquid-liquid extraction of calcium with a serpentine microfluidic device. International Communications in Heat and Mass Transfer, 140, 106551. http://doi.org/10.1016/j.icheatmasstransfer.2022.106551
- Amini, Y., Karimi-Sabet, J., & Nasr Esfahany, M. (2016). Experimental and numerical study of multiphase flow in new wire gauze with high capacity structured packing. Chemical Engineering and Processing: Process Intensification, 108, 35–43. https://doi.org/10.1016/j.cep.2016.07.003
- Amini, Y., Shadman, M. M., Ghazanfari, V., & Hassanvand, A. (2023c). Enhancement of immiscible fluid mixing using passive micromixers to increase the performance of liquid-liquid extraction. International Journal of Modern Physics C. https://doi.org/10.21203/rs.3.rs-2307741/v1
- Bandulasena, M. V., Vladisavljević, G. T., & Benyahia, B. (2019). Versatile reconfigurable glass capillary microfluidic devices with Lego® inspired blocks for drop generation and micromixing. Journal of Colloid and Interface Science, 542, 23–32. https://doi.org/10.1016/j.jcis.2019.01.119
- Brackbill, J. U., Kothe, D. B., & Zemach, C. (1992). A continuum method for modeling surface tension. Journal of Computational Physics, 100(2), 335–354. https://doi.org/10.1016/0021-9991(92)90240-Y
- Chen, L., Gao, M., Liang, J., Wang, D., Hao, L., & Zhang, L. (2022). Lattice Boltzmann simulation of wetting gradient accelerating droplets merging and shedding on a circumferential surface. Engineering Applications of Computational Fluid Mechanics, 16(1), 1796–1812. https://doi.org/10.1080/19942060.2022.2116488
- Chen, Y., Wu, L., & Zhang, L. (2015). Dynamic behaviors of double emulsion formation in a flow-focusing device. International Journal of Heat and Mass Transfer, 82, 42–50. https://doi.org/10.1016/j.ijheatmasstransfer.2014.11.027
- Ge, X., Rubinstein, B. Y., He, Y., Bruce, F. O., Li, L., Leshansky, A. M., & Li, Z. (2021). Double emulsions with ultrathin shell by microfluidic step-emulsification. Lab on a Chip, 21(8), 1613–1622. https://doi.org/10.1039/D0LC01044H
- Guadarrama-Lezama, A. Y., Dorantes-Alvarez, L., Jaramillo-Flores, M. E., Pérez-Alonso, C., Niranjan, K., Gutiérrez-López, G. F., & Alamilla-Beltrán, L. (2012). Preparation and characterization of non-aqueous extracts from chilli (Capsicum annuum L.) and their microencapsulates obtained by spray-drying. Journal of Food Engineering, 112(1-2), 29–37. https://doi.org/10.1016/j.jfoodeng.2012.03.032
- Hirt, C. W., & Nichols, B. D. (1981). Volume of fluid (VOF) method for the dynamics of free boundaries. Journal of Computational Physics, 39(1), 201–225. https://doi.org/10.1016/0021-9991(81)90145-5
- Jafari, A., & Shamloo, A. (2023). CFD study of droplet formation in a cross-junction microfluidic device: investigating the impact of outflow channel design and viscosity ratio. Engineering Applications of Computational Fluid Mechanics, 17(1), 2243091. https://doi.org/10.1080/19942060.2023.2243091
- Jafari, O., Rahimi, M., & Kakavandi, F. H. (2015). Liquid-liquid extraction in twisted micromixers. Chemical Engineering and Processing: Process Intensification, 101, 33–40. https://doi.org/10.1016/j.cep.2015.12.013
- Kim, I., & Wu, X. L. (2010). Tunneling of micron-sized droplets through soap films. Physical Review E, 82(2), 026313. https://doi.org/10.1103/PhysRevE.82.026313
- Leister, N., Vladisavljevi, G. T., & Karbstein, H. P. (2022). Novel glass capillary microfluidic devices for the flexible and simple production of multi-cored double emulsions. Journal of Colloid and Interface Science, 611, 451–461. https://doi.org/10.1016/j.jcis.2021.12.094
- Liu, X., Wang, C., Zhao, Y., & Chen, Y. (2018). Shear-driven two colliding motions of binary double emulsion droplets. International Journal of Heat and Mass Transfer, 121, 377–389. https://doi.org/10.1016/j.ijheatmasstransfer.2018.01.021
- Liu, X., Wu, L., Zhao, Y., & Chen, Y. (2017). Study of compound drop formation in axisymmetric microfluidic devices with different geometries. Colloids and Surfaces A: Physicochemical and Engineering Aspects, 533, 87–98. https://doi.org/10.1016/j.colsurfa.2017.08.026
- Nabavi, S. A., Vladisavljević, G. T., Bandulasena, M. V., Arjmandi-Tash, O., & Manović, V. (2017b). Prediction and control of drop formation modes in microfluidic generation of double emulsions by single-step emulsification. Journal of Colloid and Interface Science, 505, 315–324. http://doi.org/10.1016/j.jcis.2017.05.115
- Nabavi, S. A., Vladisavljević, G. T., Gu, S., & Ekanem, E. E. (2015). Double emulsion production in glass capillary microfluidic device: Parametric investigation of droplet generation behaviour. Chemical Engineering Science, 130, 183–196. https://doi.org/10.1016/j.ces.2015.03.004
- Nabavi, S. A., Vladisavljević, G. T., & Manović, V. (2017a). Mechanisms and control of single-step microfluidic generation of multi-core double emulsion droplets. Chemical Engineering Journal, 322, 140–148. http://doi.org/10.1016/j.cej.2017.04.008
- Okushima, S., Nisisako, T., Torii, T., & Higuchi, T. (2004). Controlled production of monodisperse double emulsions by two-step droplet breakup in microfluidic devices. Langmuir the Acs Journal of Surfaces & Colloids, 20(23), 9905–9908. https://doi.org/10.1021/la0480336
- Ong, W., Hua, J., Zhang, B., Teo, T., Zhuo, J., Nguyen, N., Ranganathan, N., & Yobas, L. (2007). Experimental and computational analysis of droplet formation in a high-performance flow-focusing geometry. Sensors and Actuators A: Physical, 138(1), 203–212. https://doi.org/10.1016/j.sna.2007.04.053
- Oomen, P. E., Skolimowski, M. D., & Verpoorte, E. (2016). Implementing oxygen control in chip-based cell and tissue culture systems. Lab on A Chip, 16(18), 3394–3414. https://doi.org/10.1039/C6LC00772D
- Pan, D., Chen, Q., Chen, S., & Li, B. (2020). Experimental study on millimeter-scale W1/O/W2 compound droplets formation in a co-flowing device with two-step structure. Chemical Engineering Science, 216, 115493. https://doi.org/10.1016/j.ces.2020.115493
- Pan, D., Liu, M., Li, F., Chen, Q., Liu, X., Liu, Y., Zhang, Z., Huang, W., & Li, B. (2017). Formation mechanisms of solid in water in oil compound droplets in a horizontal T-junction device. Chemical Engineering Science, 176, 254–263. https://doi.org/10.1016/j.ces.2017.10.049
- Peng, F., Wang, Z., Fan, Y., Yang, Q., & Chen, J. (2022a). Study on the interfacial dynamics of free oscillatory deformation and breakup of single-core compound droplet. Physics of Fluids, 34(4), 7. http://doi.org/10.1063/5.0087738
- Peng, F., Wang, Z., Yang, Q., Fan, Y., & Chen, J. (2022b). Numerical simulation on free oscillation interfacial dynamics of single-core compound droplet driven by shell deformation. European Journal of Mechanics - B/Fluids, 95, 52–62. http://doi.org/10.1016/j.euromechflu.2022.04.001
- Qian, J., & Law, C. K. (1997). Regimes of coalescence and separation in droplet collision. Journal of Fluid Mechanics, 331, 59–80. https://doi.org/10.1017/S0022112096003722
- Sattari, A., Hanafizadeh, P., & Keshtiban, M. M. (2021a). Microfluidic preparation of double emulsions using a high aspect ratio double co-flow device. Colloids and Surfaces A: Physicochemical and Engineering Aspects, 628, 127297. http://doi.org/10.1016/j.colsurfa.2021.127297
- Sattari, A., Tasnim, N., Hanafizadeh, P., & Hoorfar, M. (2021b). Numerical study of double emulsion droplet generation in a dual-coaxial microfluidic device using response surface methodology. Chemical Engineering and Processing - Process Intensification, 162, 108330. http://doi.org/10.1016/j.cep.2021.108330
- Shang, L., Cheng, Y., Wang, J., Ding, H., Rong, F., Zhao, Y., & Gu, Z. (2014). Double emulsions from a capillary array injection microfluidic device. Lab on A chip, 14(18), 3489–3493. https://doi.org/10.1039/C4LC00698D
- Shao, C., Chi, J., Shang, L., Fan, Q., & Ye, F. (2021). Droplet microfluidics-based biomedical microcarriers. Acta biomaterialia, 138, 21–33. https://doi.org/10.1016/j.actbio.2021.10.037
- Shao, T., Feng, X., Jin, Y., & Cheng, Y. (2013). Controlled production of double emulsions in dual-coaxial capillaries device for millimeter-scale hollow polymer spheres. Chemical Engineering Science, 104, 55–63. https://doi.org/10.1016/j.ces.2013.09.001
- Tan, S., Gao, C., Liu, H., Ye, B., & Sun, D. (2020). Research of double emulsion formation and shell-thickness influence factors in a novel six-way junction microfluidic device. Colloids and Surfaces A: Physicochemical and Engineering Aspects, 601, 124917. https://doi.org/10.1016/j.colsurfa.2020.124917
- Utada, A. S., Lorenceau, E., Link, D. R., Kaplan, P. D., Stone, H. A., & Weitz, D. A. (2005). Monodisperse double emulsions generated from a microcapillary device. Science, 308(5721), 537–541. https://doi.org/10.1126/science.1109164
- Visser, C. W., Kamperman, T., Karbaat, L. P., Lohse, D., & Karperien, M. (2018). In-air microfluidics enables rapid fabrication of emulsions, suspensions, and 3D modular (bio)materials. Science Advances, 4(1), eaao1175. https://doi.org/10.1126/sciadv.aao1175
- Vu, T. V., Homma, S., Tryggvason, G., Wells, J. C., & Takakura, H. (2013). Computations of breakup modes in laminar compound liquid jets in a coflowing fluid. International Journal of Multiphase Flow, 49, 58–69. https://doi.org/10.1016/j.ijmultiphaseflow.2012.10.004
- Wang, N., Semprebon, C., Liu, H., Zhang, C., & Kusumaatmaja, H. (2020). Modelling double emulsion formation in planar flow-focusing microchannels. Journal of Fluid Mechanics, 895. https://doi.org/10.1017/jfm.2020.299
- Wu, L., Liu, X., Zhao, Y., & Chen, Y. (2017). Role of local geometry on droplet formation in axisymmetric microfluidics. Chemical Engineering Science, 163, 56–67. https://doi.org/10.1016/j.ces.2017.01.022
- Yang, S., Guo, F., Kiraly, B., Mao, X., Lu, M., Leong, K. W., & Huang, T. J. (2012). Microfluidic synthesis of multifunctional Janus particles for biomedical applications. Lab on A Chip, 12(12), 2097. https://doi.org/10.1039/c2lc90046g
- Yoshida, K., Sekine, T., Matsuzaki, F., Yanaki, T., & Yamaguchi, M. (1999). Stability of vitamin A in oil-in-water-in-oil-type multiple emulsions. Journal of the American Oil Chemists’ Society, 76(2), 1–6. https://doi.org/10.1007/s11746-999-0212-2
- Yu, C., Wu, L., Li, L., & Liu, M. (2018). Experimental study of double emulsion formation behaviors in a one-step axisymmetric flow-focusing device. Experimental Thermal and Fluid Science, 103, 18–28. https://doi.org/10.1016/j.expthermflusci.2018.12.032
- Yu, W., Li, B., Liu, X., & Chen, Y. (2021). Hydrodynamics of triple emulsion droplet generation in a flow-focusing microfluidic device. Chemical Engineering Science, 243, 116648. https://doi.org/10.1016/j.ces.2021.116648
- Zeng, W., Li, S., & Wang, Z. (2015). Closed-loop feedback control of droplet formation in a T-junction microdroplet generator. Sensors and Actuators A: Physical, 233, 542–547. https://doi.org/10.1016/j.sna.2015.08.002
- Zhang, T., Zou, X., Xu, L., Pan, D., & Huang, W. (2021). Numerical investigation of fluid property effects on formation dynamics of millimeter-scale compound droplets in a co-flowing device. Chemical Engineering Science, 229, 116156. https://doi.org/10.1016/j.ces.2020.116156
- Zhou, Y., Ma, X., Wang, S., & Liu, D. (2022). Generation and evolution of double emulsions in a circular microchannel. Chemical Engineering Science, 255, 117683. https://doi.org/10.1016/j.ces.2022.117683
- Zhu, P., & Wang, L. (2016). Passive and active droplet generation with microfluidics: a review. Lab on a Chip, 17(1), 34–75. https://doi.org/10.1039/C6LC01018K