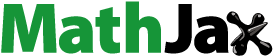
Abstract
Fiber-reinforced polymer (FRP) composites are utilized as a substitute material for metal structures in maritime industries. The durability of composites depends on varying harsh marine environments. The continuous exposure of FRPs to the harsh marine environment affects their static and dynamic properties. In this study, the impact of moisture absorption on the fatigue characteristics of autoclave-cured quasi-isotropic carbon/epoxy laminates is examined. Specimens were aged in artificial seawater under ambient, sub-zero, and humid conditions for 365 d. The fatigue test was conducted at different stress levels on aged laminates. The S-N curve, stiffness degradation, damage accumulation, and rate of damage growth using energy dissipation method were evaluated based on the experimental results. The results showed that fatigue property of quasi-isotropic carbon/epoxy specimens largely depends on aging conditions. The S-N curve of aged specimens showed a greater fatigue life at higher stress levels than the pristine specimen. Under different aging conditions and varying stress levels, specimens exhibited a standard damage curve. A total of 60–70% damage was developed during the initial stages of fatigue loading. The presence of moisture, multiple cracks, and interphase debonding between fibers and matrix were responsible for the reduction in fatigue life of quasi-isotropic carbon/epoxy specimens.
Introduction
Fiber-reinforced polymer (FRP) composites are gaining importance in marine industry due to their better fatigue properties and damage tolerance limit than metals [Citation1–3]. Glass fiber-reinforced polymer (GFRP) and Carbon fiber-reinforced polymer (CFRP) composite materials are widely used in the marine structure. When there is a need for critical load conditions, CFRP finds more suitable than GFRP composites due to their better strength-to-weight ratio, low density, and better fatigue strength [Citation4–6]. CFRP laminates find various applications in marine industry, such as stiffer hulls, girders, masts, and propeller shaft. The use of CFRP composites in marine hull structures reduces a 30% weight, resulting in better mechanical and fatigue properties [Citation7, Citation8]. In the service life of marine structures, FRP composites are frequently subjected to tension-tension fatigue loading, wave loading, shock loading, etc. The fatigue resistance of the FRP laminates mainly depends on the constituent materials, lamination sequence, types of resin, and environmental conditions [Citation9–11]. The combined effect of fatigue loading and harsh marine environments, like hygrothermal conditions, elevated temperatures, and corrosion environments, induce a higher percentage of fatigue damage accumulation in marine structures. The changes in fatigue properties under these conditions occur over an extended service life period. To understand the behavior of marine structures in these environments, researchers focused on developing different accelerated aging processes [Citation12, Citation13]. Aging study was performed to investigate the changes in mechanical, chemical and fatigue properties of FRP specimens over a time when exposed to different environmental conditions. Aging study provides a better understanding of effect of moisture absorption on mechanical behavior of FRP structures under different environmental conditions [Citation14, Citation15]. Moisture diffusion into FRP relays on several varibles, such as the rate of diffusion of moisture into matrix phases, types of matrix, and an interface between fiber and matrix [Citation16–18]. Exposure of FRP laminates to moisture induces hydrolysis, which leads to the swelling of the matrix material. The swelling of matrix decreases the interface adhesion between the matrix and the fiber, ultimately reducing fatigue properties of the material [Citation19–21].
In recent years, many research studies have concentrated on aging and the effect of aging on fatigue properties of FRP materials. Wu et al. [Citation22] examined the effect of water and alkaline solution aging on the fatigue properties of pultruded CFRP plates. The aging process continued for a duration of two years. CFRP specimens aged under alkaline solution showed higher degradation in fatigue properties than in distilled water. The Scanning electron microscope (SEM) observation of fatigue failure surfaces of aged CFRP specimens showed the presence of matrix cracks. The analysis of fatigue properties of glass/epoxy composites exposed to salt water for 18 weeks indicated a notable decrease in fatigue life. This reduction can be attributed to a decline in the interphase between the fiber and matrix. The moisture absorption reduced the shear properties. SEM studies indicated the weak interphase between fiber and matrix, resulting in delamination of the structure and resulted in a reduction of fatige life of the material [Citation23].
Behera et al. [Citation24] performed an experiment to study the effect of tap water aging on the fatigue properties of multidirectional CFRP laminates. Aging of CFRP laminates for a duration of one year resulted in 18% decrement in fatigue properties as compared to the pristine specimens. SEM studies revealed that existence of matrix erosion and fiber debonding, which further accelerated moisture absorption and led to a reduction in fatigue life. Koshima et al. [Citation25] evaluated the fatigue life of carbon/vinyl ester composites immersed in natural seawater for 100, 225, and 385 d. Experimental fatigue results showed that fatigue life reduced as the aging duration increased. Decreased load transmission efficiency and deterioration of the fiber-matrix interphase reduced the fatigue life of structures. The fatigue life of flax/epoxy composite aged for 240 h in salt water showed a reduction in fatigue properties compared to a pristine specimen. Water diffusion into matrix material-induced plasticization resulted in decreased fatigue properties, and the initial fatigue modulus of structure was reduced to 70% as compared to pristine specimens [Citation26].
The fatigue damage developed in polymer composites was studied using stiffness degradation, energy dissipation, acoustic emission or infrared methodologies. Wang et al. [Citation27] developed a stiffness degradation model to study the fatigue damage developed in salt water aged basalt fiber composites. Based on the experimental results, it has been observed that the stiffness of the aged specimens was degraded by 30% as compared to pristine specimens. According to fatigue life prediction model, the fatigue strength of composite is decreased by 11% as compared to pristine specimens. The fatigue strength of seawater-aged CFRP laminates showed a significant reduction in fatigue life. The reduction in fatigue life due to aging is substantial at higher stress ratios in comparison with the lower stress ratios. The stiffness is reduced significantly for aged specimens than for the pristine specimens [Citation28]. Shan and Liao [Citation29] developed a phenomenological model to study the fatigue damage of glass/carbon/epoxy hybrid laminates aged under distilled water. The aged specimens showed a reduction in fatigue life at lower stress levels compared to higher stress levels. The phenomenological model developed can predict the fatigue behavior of hybrid composites.
Artificial seawater aging had a significant impact on the fatigue properties of glass/epoxy composites, resulting in a 38% reduction in stiffness compared to pristine specimens. The stiffness degradation study revealed that the damage progression followed the classic FRP damage curve. For pristine and aged glass/epoxy specimens, 60–70% of the damage was observed in the initial fatigue loading cycle [Citation30]. Zhao et al. [Citation31] developed an energy dissipation model to predict the fatigue strength of aged basalt/epoxy composites subjected to different environmental temperature conditions. The temperature conditions had an adverse effect on fatigue life at lower stress levels compared to higher stress levels. As per the energy dissipation model, the extent of energy dissipated was small at higher stress levels due to the limited difference between maximum and minimum applied loads.
According to authors’ understanding, several research works have been performed on impact of aging on fatigue properties of fiber-reinforced composites. However, these studies focused on unidirectional or angle ply laminates. Limited research has been conducted on the influence of aging on fatigue properties on quasi-isotropic carbon/epoxy laminates. This study investigates the effect of aging on tension–tension fatigue behavior of quasi-isotropic carbon/epoxy laminates. The aging study was performed for one year by immersing specimens in artificial seawater at ambient, sub-zero and humid conditions. Fatigue study was of aged carbon/epoxy specimen was performed under tension-tension loading condition with a stress ratio of R = 0.1. Damage developed during cyclic loading was evaluated by considering the energy dissipated in each loading cycle. Material characteristics were estimated and compared with published results of pristine specimens reported in Padmaraj et al. [Citation32].
Experimental details
Materials and methodology
Quasi-isotropic carbon/epoxy specimen was fabricated using an autoclave curing process. The unidirectional carbon/epoxy prepreg, containing 38% resin content, was obtained from Bhor Chemicals and Private Ltd. in Nasik, India. Reinforcement material used was carbon fiber, having a density of 1.8 g/cm3 and a tensile modulus of 240 GPa. Matrix material used was A45, having a tensile modulus of 2.91 GPa. A total of eight layers of carbon/epoxy laminate ([0˚/±45˚/90˚]s) fabricated utilizing a hand layup method and cured in a lab-scale autoclave using a two-stage curing process. The average thickness, density and void content of the fabricated carbon/epoxy laminate were 1.7 mm, 1.54 ± 0.041 g/cm3 and 1.315% and is reported in Padmaraj et al. [Citation32, Citation33].
Fatigue test
The aim of this work is to investigate the effect of moisture absorption on the fatigue properties of quasi-isotropic carbon/epoxy laminates. Artificial seawater was prepared as per ASTM D1141 [Citation34]. The specimens were aged in ambient, sub-zero (−15 °C) and humid conditions (70% RH and 40 °C) in seawater for 365 d. The details of aging process of quasi-isotropic carbon/epoxy laminate were discussed in previously published research articles [Citation35]. The moisture absorption in the specimen was measured at regular intervals in accordance with ASTM D5529. On completion of aging process, the specimens were cut from the laminates as per ASTM standards using a waterjet cutting machine at Stonemax Waterjet Pvt Ltd Kochi, Kerala, India. The quasi-static tensile test was performed as per ASTM D 3039 using a BiSS India make, the universal testing machine with a maximum capacity of 50 kN and at a crosshead speed of 2 mm/min [Citation36]. The tension–tension fatigue test was performed on the pristine and aged quasi-isotropic carbon/epoxy laminates as per ASTM D 3479 in a load-controlled mode [Citation37]. The test was conducted at a stress ratio R = 0.1 and a frequency of 2 Hz under three different stress levels (0.8, 0.7, and 0.6). To avoid self heating of specimens, a low frequency was chosen. The fatigue test was conducted until complete failure of specimen or up to 106 cycles.
Fatigue damage characterization
The progression of damage during fatigue study was assessed by evaluating two parameters: Stiffness degradation and Energy dissipation. The reduction in stiffness during fatigue loading was estimated using experimental stress-strain data. Evaluating the dissipation of energy during cyclic loading can help in comprehending the growth of fatigue damage. The energy dissipation (H) during the fatigue loading is estimated based on EquationEquation (1)(1)
(1) [Citation32, Citation38],
(1)
(1)
where N – total number of cycles, σ – stress (MPa) applied, and ε – strain developed during each cycle. The strain generated during fatigue test was determined within the specimen’s gauge length (150 mm).
The damage growth in the specimens during fatigue testing was calculated at different stress levels based on the energy dissipated during each loading cycle and is shown in EquationEquation (2)(2)
(2) [Citation38–40],
(2)
(2)
where D(n) – damage developed during cyclic loading and varies from 0 to 1, H0, Hn, and Hf are the energy dissipation at initial loading cycle, nth cycle and at failure, respectively.
Theoretical fatigue damage of carbon/epoxy specimens at different stress levels at all fatigue loading cycles was developed as per EquationEquation (3)(3)
(3) [Citation39],
(3)
(3)
where n – numebr of cycles completed, N – total number of cycles, and A and B – material parameters.
By modifying EquationEquation (3)(3)
(3) , the rate of fatigue damage growth at the initial and final loading cycle can be estimated by using EquationEquation (4)
(4)
(4) [Citation32, Citation41],
(4)
(4)
where
- rate of fatigue damage, x =
A and B – material parameters obtained as per EquationEquation (3)
(3)
(3) .
Results and discussion
Effect of aging on the static properties
The moisture absorption revealed that moisture content in the specimens was increased with aging duration. The specimens aged under ambient conditions absorbed more moisture compared to other two aged conditions. Specimens aged under ambient conditions gained a moisture content of 0.83% compared to 0.46% in sub-zero and 0.64% in humid conditions [Citation35]. The tensile test determines in-plane tensile properties of polymeric composite materials. Five specimens were tested to determine average failure stress and strain. The tensile properties of quasi-isotropic carbon/epoxy specimens aged under three aging conditions are shown in [Citation35].
Table 1. Tensile properties of pristine and aged carbon/epoxy specimens.
The tensile properties of quasi-isotropic carbon/epoxy laminates were degraded as the aging time progressed. The reduction in tensile properties followed the sequence of Pristine > Humid > Sub-zero > Ambient. After being immersed in artificial seawater for 365 d under ambient conditions, specimens exhibited a 33.01% reduction compared to the pristine value. Under sub-zero conditions, specimens demonstrated an intermediate decrease of 24.68% in tensile properties, whereas specimens subjected to humid conditions experienced a reduction of 21.31% compared to pristine value. During the initial stages, carbon/epoxy specimens exhibited rapid moisture absorption leading to a reduced internal stress and resulting in faster decline in tensile strength. Sub-zero conditions characterized by high moisture content and freezing temperature environment, due to which matrix material experienced swelling and plasticization, resulting in the reduced tensile strength [Citation42–44]. Despite the penetration of water into the sample following saturation, water molecules continue to react with the epoxy over time, leading to irreversible chemical changes and resulting in a diminished performance. For quasi-isotropic carbon/epoxy specimens aged under seawater at elevated temperatures showed an irreversible chemical change in matrix and resulted in interfacial debonding between fiber and matrix. This phenomenon had adverse affect on mechanical properties compared to pristine specimens [Citation45]. The results indicated that both aging method and moisture absorption had lowered the matrix-dominated properties.
Effect of moisture absorption on fatigue properties
In thist study, a tension-tension fatigue study was conducted with a stress ratio of 0.1. The specimens were tested at 0.8, 0.7, and 0.6 stress levels to construct the S-N curve for different aging conditions. shows the S-N curve for pristine, ambient, sub-zero and humid conditions. The maximum stress applied during fatigue loading at different stress levels was estimated based on ultimate tensile strength of each specimen under specific condition. Fatigue testing was carried out until either complete specimen failure or up to 106 cycles.
For both pristine and aged carbon/epoxy specimen, a greater dispersion in fatigue life was observed at higher stress levels. Due to the higher amplitude of applied load and shorter duration of cyclic loading at higher stress levels (0.8 and 0.7), the pristine and aged specimens showed low fatigue life. Using least square method, a linear regression was performed between the applied stress level and material’s life and was represented using Equation (5) [Citation10],
In Equation (5), σmax represents maximum stress applied in MPa, σu average tensile in MPa, A and B are the model parameters, A corresponda to slope of the curve and represents fatigue life detoriration rate and B represents curve intercept which should be >1. The model parameters for the present configuration for both pristine and aged specimens were calculated from Equation (5) and are shown in .
Table 2. Values of model parameters of pristine and aged carbon/epoxy specimens.
The term R2 signifies the quality of fit between experimental and regression data. A unified value of R2 suggests a good agreement between the curves. The deterioration rate in fatigue life is nearly same for aged specimens but it is higher when compared to pristine specimens. Higher value of A indicates that the influence of moisture and temperature reduced the fatigue life of quasi-isotropic carbon/epoxy composites.
As shown in , the specimens aged under ambient and sub-zero conditions showed an average fatigue life of 7.05 × 104 and 4.95 × 104, respectively, at higher stress levels (0.8) than the pristine and humid-aged specimens. Low-cycle fatigue is typically characterized by higher stress levels. It is generally associated with an elastic strain range where a small amplitude of applied load is enough to create fatigue damage. In the case of ambient and sub-zero aged specimens, the load applied at a higher stress level was insufficient to create a fiber breakage and resulted in higher life. Based on these results at higher stress levels, it can be inferred that hygrothermal aging, although causing a reduction in static stiffness and strength, actually enhances fatigue resistance. This improvement can likely be attributed to increased damage tolerance resulting from fiber swelling and the potential strengthening of the fiber-matrix interface. The lower stress levels are always associated with a plastic strain range, requiring a higher fatigue load for developing fatigue damage and resulting in higher fatigue life. The pristine specimens showed a better fatigue life at lower stress levels than the aged specimens. The reason behind such a reduced fatigue life in an aged specimen is the lack of ability of the composite to drain the salt water entrapped in the pores, which acts as a stress concentrator and accelerates onset failures. The presence of such damage may not be visual but significantly reduces the fatigue life.
Stiffness degradation
Stiffness degradation is a critical issue affecting structures’ performances and safety. Monitoring stiffness degradation during fatigue loading is essential because stiffness of a material is a key parameter which determines their ability to withstand load and stress. The normalized stiffness degradation curve plot of both pristine and aged quasi-isotropic carbon/epoxy laminate for 0.7 and 0.6 stress levels is shown in . Stiffness degradation curve can be divided into three phases. Phase I, known as initiation phase, showed a rapid stiffness reduction due to crack formation. The damage in Phase I is usually small and localized. Phase II, the propagation phase, observed a steady degradation in stiffness. The second phase covers a large portion of fatigue life. Within this phase, damage was due to growth of matrix cracks between the interplay. As the cyclic loading proceeds, the propagation of cracks leads to a reduction in fiber matrix interply adhesion and results in fiber and matrix separation. Phase III, the localization phase, observed various damage accumulation, causing a significant reduction in the stiffness of the specimens and leading to their eventual catastrophic failure [Citation46].
Figure 2. Stiffness degradation curve for pristine and aged carbon/epoxy specimens a) 0.7 stress level and b) 0.6 stress level.

As shown in , the stiffness is degraded more in sub-zero conditions than in other conditions at 0.7 stress level. At 0.6 stress level, the specimens aged under ambient conditions showed more degradation in stiffness. From the above , it can be clearly understood that the effect of moisture caused more significant initial damage in the specimen due to the changes in the chemical and physical properties. The moisture induced in the specimen resulted in plasticization and swelling, thereby reducing the modulus of the composite. The experimental findings revealed that aging effect has minimal influence on rate of damage propagation at lower stress levels. There were a few variations at the end of the stiffness degradation curve, which can be attributed to measurement errors or experimental conditions or maybe because of the testing variability.
Damage growth analysis based on energy dissipation
The fatigue loading cycle comprises two phases: loading and unloading. In the loading phase, energy is dissipated, while in the unloading phase, energy is released. Estimating and analyzing such a phenomenon during cyclic loading helps in comprehending the fatigue damage growth behavior within specimen. The energy dissipated in pristine and aged specimens at 0.7, and 0.6 stress levels were calculated based on EquationEquation (1)(1)
(1) and is shown in .
The energy dissipation curve for pristine and aged quasi-isotropic carbon/epoxy specimens shown in can be divided into three regions. Region I is associated with a initial rapid increase in energy dissipation, representing the formation of matrix cracks. Region II is associated with slow and steady energy dissipation, and Region III again observed a sudden rise in energy dissipation, representing final failure of the specimens. At stress level of 0.7, the energy dissipation is lower in ambient aged specimens than the other aging conditions. This is attributed to the fact that during ambient aging, the material produces more resistance to deformation and cracking of CFRP material, reducing the degradation of material properties. Whereas, at 0.6 stress levels, the specimens aged in humid conditions showed less energy dissipation than those aged in other conditions. As the specimens aged in humid conditions, they exhibited a significant improvement in ductility, resulting in improved toughness. Due to these factors, energy dissipation was less in humid conditions compared to ambient and sub-zero conditions. The moisture absorption caused three main effects: increase in mean strain, decrease in initial dynamic modulus and increase in energy dissipation [Citation26, Citation47]. At higher stress levels, the initial rapid energy dissipation in all the aging conditions is observed, which may be attributed to post-curing and physical aging. At lower stress levels, decreased energy dissipation is observed, which may be attributed to decreased fiber strength, matrix plasticization and interface degradation [Citation48]. Based on the energy dissipated data obtained, the damage developed in the quasi-isotropic carbon/epoxy specimen was estimated using EquationEquation (2)(2)
(2) and is shown in . The theoretical damage growth was calculated based on EquationEquation (3)
(3)
(3) and is shown in . The damage growth trend for pristine and aged specimens aligned with published literature [Citation49, Citation50].
Figure 4. Experimental and Theoretical damage growth behavior of pristine and aged carbon/epoxy specimens (a) 0.7 stress level and (b) 0.6 stress level.

For both pristine and aged carbon/epoxy specimens at 0.7 and 0.6 stress levels, the initial stages of fatigue loading exhibited a rapid damage growth with a logarithmic evaluation [Citation32]. This phase corresponds to rapid formation of matrix cracks and several damages. In the second phase, the damage growth was linear and is mainly attributed to micro and macro cracks’ development due to the delamination and fiber breakage. During final phase of fatigue loading, the damage was rapid and demonstrated exponential evaluation of damage growth near failure points. Model parameters for pristine and aged specimens A and B in EquationEquation (3)(3)
(3) were determined through linear fitting and is shown in . The term R2 in indicates accuracy of curve fitting, and the values of R2 are close to unity in both pristine and aged conditions exhibiting the accuracy of the fitting. Using the values of model parameters A and B, it is possible to determine damage growth at any cycle for both pristine and aged carbon/epoxy specimens.
Table 3. Damage model parameters for pristine and aged carbon/epoxy quasi-isotropic laminates.
The rate of damage growth at distinct stages of loading cycle for pristine and aged quasi-isotropic carbon/epoxy laminate was calculated as per EquationEquation (4)(4)
(4) and is shown in .
Figure 5. Damage growth rate at different loading cycles a) 0.7 stress level and b) 0.6 stress level.

When comparing damage growth rate during initial loading cycle, it is evident that for both pristine and aged quasi-isotropic carbon/epoxy specimens exhibit quite similar behavior at a stress level of 0.7 and 0.6 (). At 0.7 and 0.6 stress levels, comparing damage growth rate with experimental damage growth behavior (), it can be observed that for both pristine and aged specimens about 60% damage was accumulated during the initial loading cycles. After the initial cyclic loading, damage growth rate was stable and linear compared with experimental damage growth behavior (). During the final stages of fatigue loading, damage growth was rapid due to specimen’s catastrophic failure at 0.7 and 0.6 stress levels. Hence, near failure points damage growth rate is varied in comparison with initial loading cycles under both pristine and aged conditions.
It is expected that the energy dissipation method can be effectively used to predict the damage criteria during fatigue loading. However, further investigation can be undertaken to study the fatigue damage by developing mathematical modeling.
Conclusions
This study analyses the effect of moisture absorption on fatigue properties of quasi-isotropic carbon/epoxy specimens. The fatigue test was performed at a stress ratio of 0.1 and with a frequency rate of 2 Hz. The following conclusions were drawn from the results obtained.
The moisture absorption of carbon/epoxy laminates was predominantly influenced by aging medium and duration. The absorption of moisture had an adverse impact on mechanical properties of the carbon/epoxy laminates. As the aging time increased, the tensile strength decreased.
The moisture absorption has substantially affected the fatigue life of quasi-isotropic carbon/epoxy laminates. In both pristine and aged carbon/epoxy specimens the initial loading cycle exhibited a greater rate of stiffness degradation. The S-N curve of aged specimens showed a greater fatigue life at higher stress levels than the pristine specimen.
The stiffness degradation, energy dissipation, and damage growth curve for both pristine and aged conditions are composed of three stages. A total of 60–70% damage was developed during the initial stages of fatigue loading. The combined influence of humidity and temperature is the reason for the higher damage growth rate. The theoretical damage model based on energy dissipation could predict the damage at any loading cycle.
Supplemental Material
Download Zip (24.8 KB)Acknowledgments
The authors express their gratitude to the Naval Research Board, Government of India (Project No: NRB-438/MAT/18-19) for providing the funding. Additionally, the authors thank the Manipal Institute of Technology, Manipal, Manipal Academy of Higher Education, Manipal, for providing fabrication and testing facilities.
Disclosure statement
The authors declare that they have no known competing financial interests or personal relationships that could have appeared to influence the work reported in this article.
Data availability statement
The authors confirm that the data supporting the findings of this study are available within the article and its Supplementary Materials.
Additional information
Funding
References
- Mayya HB, Pai D, Kini VM, et al. Effect of marine environmental conditions on physical and mechanical properties of Fiber-Reinforced composites—a review. J Inst Eng India Ser C. 2021;102(3):843–849. doi: 10.1007/s40032-021-00676-w.
- Subhani M, Al-Ameri R. Strength reduction in square columns confined with CFRP under marine environment. Compos B Eng. 2016;97:183–192. doi: 10.1016/j.compositesb.2016.05.016.
- Rao YS, Mohan NS, Shetty N, et al. Drilling and structural property study of multi-layered fiber and fabric reinforced polymer composite - a review. Mater Manuf Process. 2019;34(14):1549–1579. doi: 10.1080/10426914.2019.1686522.
- Manjunatha CM, Srihari S. A brief review on the fatigue behavior of continuous fiber reinforced thermosetting epoxy polymer based nanocomposites. Trans Indian Natl Acad Eng. 2022;7(2):501–507. doi: 10.1007/s41403-022-00320-8.
- Kühn F, Rehra J, May D, et al. Dry fiber placement of carbon/steel fiber hybrid preforms for multifunctional composites. Adv Manuf Polym Compos Sci. 2019;5(1):37–49. doi: 10.1080/20550340.2019.1585027.
- Achukwu EO, Owen MM, Shuib SB, et al. Effect of reprocessing on the service life properties of glass fiber-reinforced in-house polypropylene waste composites. Adv Manuf Polym Compos Sci. 2023;9(1):2023.
- Rubino F, Nisticò A, Tucci F, et al. Marine application of fiber reinforced composites: a review. JMSE. 2020;8(1):26. doi: 10.3390/jmse8010026.
- Gargano A, Pingkarawat K, Blacklock M, et al. Comparative assessment of the explosive blast performance of carbon and glass fibre-polymer composites used in naval ship structures. Compos Struct. 2017;171:306–316. doi: 10.1016/j.compstruct.2017.03.041.
- Guo R, Li C, Niu Y, et al. The fatigue performances of carbon fiber reinforced polymer composites - A review. J Mater Res Technol. 2022;21:4773–4789. doi: 10.1016/j.jmrt.2022.11.053.
- Vieira PR, Carvalho EML, Vieira JD, et al. Experimental fatigue behavior of pultruded glass fibre reinforced polymer composite materials. Compos B Eng. 2018;146:69–75. doi: 10.1016/j.compositesb.2018.03.040.
- Maragoni L, Carraro PA, Quaresimin M. Influence of manufacturing-induced defects on the fatigue performances of autoclave moulded laminates. Adv Manuf Polym Compos Sci. 2021;7(2):36–47. doi: 10.1080/20550340.2021.1967650.
- Černý I, Mayer RM. Evaluation of static and fatigue strength of long fiber GRP composite material considering moisture effects. Compos Struct. 2010;92(9):2035–2038. doi: 10.1016/j.compstruct.2009.11.024.
- Ansari MTA, Singh KK, Azam MS. Fatigue damage analysis of fiber-reinforced polymer composites—a review. J Reinf Plast Compos. 2018;37(9):636–654. doi: 10.1177/0731684418754713.
- R. D. Karbhari VM, Chin JW, Hunston D, et al. Durability gap analysis for fiber-reinforced polymer composites in civil infrastructure. J Compos Constr. 2003;7(3):238–247. doi: 10.1061/(ASCE)1090-0268(2003)7:3(238).
- Hota G, Barker W, Manalo A. Degradation mechanism of glass fiber/vinylester-based composite materials under accelerated and natural aging. Constr Build Mater. 2020;256:119462. doi: 10.1016/j.conbuildmat.2020.119462.
- Wang J, GangaRao H, Liang R, et al. Durability and prediction models of fiber-reinforced polymer composites under various environmental conditions: a critical review. J Reinf Plast Compos. 2016;35(3):179–211. doi: 10.1177/0731684415610920.
- Balcıoğlu HE, Sakin R, Gün H. Effects of hostile solutions on the static and dynamic behavior of carbon/epoxy composites. J Compos Mater. 2021;55(25):3671–3685. doi: 10.1177/00219983211020094.
- Kootsookos A, Mouritz AP. Seawater durability of glass- and carbon-polymer composites. Compos Sci Technol. 2004;64(10–11):1503–1511. doi: 10.1016/j.compscitech.2003.10.019.
- Idrisi AH, Mourad AI, Abdel-Magid BM, et al. Investigation on the durability of E-Glass/epoxy composite exposed to seawater at elevated temperature. Polymer. 2021;13(13):2182. doi: 10.3390/polym13132182.
- Vijaya Kini M, Pai D, Shreepannaga. The ageing effect on static and dynamic mechanical properties of fibre reinforced polymer composites under marine environment- A review. Mater Today Proc. 2022;52(3);689–696. doi: 10.1016/j.matpr.2021.10.084.
- Resch-Fauster K, Džalto J, Anusic A, et al. Effect of the water absorptive capacity of reinforcing fibers on the process ability, morphology, and performance characteristics of composites produced from polyfurfuryl alcohol. Adv Manuf Polym Compos Sci. 2018;4(1):13–23. doi: 10.1080/20550340.2018.1436234.
- Wu P, Xu L, Luo J, et al. Influences of long-term immersion of water and alkaline solution on the fatigue performances of unidirectional pultruded CFRP plate. Constr Build Mater. 2019;205(2):344–356. doi: 10.1016/j.conbuildmat.2019.01.227.
- Gibhardt D, Doblies A, Meyer L, et al. Effects of hygrothermal ageing on the interphase, fatigue, and mechanical properties of glass fibre reinforced epoxy. Fibers. 2019;7(6):55. doi: 10.3390/fib7060055.
- Behera A, Dupare P, Thawre MM, et al. Effects of hygrothermal aging and fiber orientations on constant amplitude fatigue properties of CFRP multidirectional composite laminates. Int J Fatigue. 2020;136:105590. doi: 10.1016/j.ijfatigue.2020.105590.
- Koshima S, Yoneda S, Kajii N, et al. Evaluation of strength degradation behavior and fatigue life prediction of plain-woven carbon-fiber-reinforced plastic laminates immersed in seawater. Compos A Appl Sci Manuf. 2019;127:105645. doi: 10.1016/j.compositesa.2019.105645.
- Habibi M, Laperrière L, Hassanabadi HM. Effect of moisture absorption and temperature on quasi-static and fatigue behavior of nonwoven flax epoxy composite. Compos B Eng. 2019;166:31–40. doi: 10.1016/j.compositesb.2018.11.131.
- Wang X, Zhao X, Wu Z. Fatigue degradation and life prediction of basalt fiber-reinforced polymer composites after saltwater corrosion. Mater Des. 2019;163:107529. doi: 10.1016/j.matdes.2018.12.001.
- Attukur Nandagopal R, Chai GB, Narasimalu S. Influence of stress ratio and stress concentration on the fatigue behaviour of hygrothermal aged multidirectional CFRP composite laminate. Int J Fatigue. 2020;137:105651. doi: 10.1016/j.ijfatigue.2020.105651.
- Shan Y, Liao K. Environmental fatigue behavior and life prediction of unidirectional glass-carbon/epoxy hybrid composites. Int J Fatigue. 2001;24(2–4):847–859. doi: 10.1016/S0142-1123(01)00210-9.
- Padmaraj NH, Vijaya KM, Dayananda P. Experimental investigation on fatigue behaviour of glass/epoxy quasi-isotropic laminate composites under different ageing conditions. Int J Fatigue. 2021;143:105992. doi: 10.1016/j.ijfatigue.2020.105992.
- Zhao X, Wang X, Wu Z, et al. Temperature effect on fatigue behavior of basalt fiber-reinforced polymer composites. Polym Compos. 2019;40(6):2273–2283. doi: 10.1002/pc.25035.
- N H P, Pai K D, Shreepannaga S, et al. Fatigue behaviour and damage characterization of quasi-isotropic carbon/epoxy laminates. Cogent Eng. 2022;9(1):2077680. doi: 10.1080/23311916.2022.2077680.
- Padmaraj NH, Vijaya KM, Amritha U, et al. Slurry erosion behaviour of carbon/epoxy quasi-isotropic laminates based on Taguchi’s optimization method. Eng Fail Anal. 2021;123:105274. doi: 10.1016/j.engfailanal.2021.105274.
- ASTM D1141-98(2013). Standard practice for the preparation of substitute ocean water. West Conshohocken (PA): ASTM International; 2008. p. 98–100.
- Aithal S, Hossagadde PN, Kini MV, et al. Durability study of quasi-isotropic carbon/epoxy composites under various environmental conditions. Iran Polym J. 2023;32(7):873–885. doi: 10.1007/s13726-023-01172-x.
- ASTM. D3039/D3039M standard test method for tensile properties of polymer matrix composite materials. Annu B ASTM Stand. 2014;15:1–13.
- ASTM D3479/D3479M. Standard test method for tension-tension fatigue of polymer matrix composite materials. Annu B ASTM Stand. 2012;96:6.
- Giancane S, Panella FW, Dattoma V. Characterization of fatigue damage in long fiber epoxy composite laminates. Int J Fatigue. 2010;32(1):46–53. doi: 10.1016/j.ijfatigue.2009.02.024.
- De Finis R, Palumbo D, Galietti U. An experimental procedure based on infrared thermography for the assessment of crack density in quasi-isotropic CFRP. Eng Fract Mech. 2021;258:108108. doi: 10.1016/j.engfracmech.2021.108108.
- Padmaraj NH, Vijaya KM, Dayananda P. Experimental study on the tension-tension fatigue behaviour of glass/epoxy quasi-isotropic composites. J King Saud Univ Eng Sci. 2020;32(6):396–401. doi: 10.1016/j.jksues.2019.04.007.
- Wu F, Yao W. A fatigue damage model of composite materials. Int J Fatigue. 2010;32(1):134–138. doi: 10.1016/j.ijfatigue.2009.02.027.
- Niu YF, Yan Y, Yao JW. Hygrothermal aging mechanism of carbon fiber/epoxy resin composites based on quantitative characterization of interface structure. Polym Test. 2021;94:107019. doi: 10.1016/j.polymertesting.2020.107019.
- Ghabezi P, Harrison NM. Indentation characterization of glass/epoxy and carbon/epoxy composite samples aged in artificial salt water at elevated temperature. Polym Test. 2022;110:107588. doi: 10.1016/j.polymertesting.2022.107588.
- Wang Z, Zhao XL, Xian G, et al. Long-term durability of basalt- and glass-fibre reinforced polymer (BFRP/GFRP) bars in seawater and sea sand concrete environment. Constr Build Mater. 2017;139:467–489. doi: 10.1016/j.conbuildmat.2017.02.038.
- Ghabezi P, Harrison NM. Hygrothermal deterioration in carbon/epoxy and glass/epoxy composite laminates aged in marine-based environment (degradation mechanism, mechanical and physicochemical properties). J Mater Sci. 2022;57(6):4239–4254. doi: 10.1007/s10853-022-06917-2.
- Vassilopoulos AP. The history of fiber-reinforced polymer composite laminate fatigue. Int J Fatigue. 2020;134:105512. doi: 10.1016/j.ijfatigue.2020.105512.
- Ansari MTA, Singh KK, Azam MS. Observations of fatigue damage development in woven glass fiber-reinforced polymer composite using transmission light photography technique. Polym Polym Compos. 2022;30:096739112211013. doi: 10.1177/09673911221101300.
- Ugochukwu S, Ridzuan MJM, Abdul Majid MS, et al. Effect of thermal ageing on the scratch resistance of natural-fibre-reinforced epoxy composites. Compos Struct. 2021;261:113586. doi: 10.1016/j.compstruct.2021.113586.
- Alam P, Mamalis D, Robert C, et al. The fatigue of carbon fibre reinforced plastics - A review. Compos B Eng. 2019;166:555–579. doi: 10.1016/j.compositesb.2019.02.016.
- Ziemian CW, Ziemian RD, Haile KV. Characterization of stiffness degradation caused by fatigue damage of additive manufactured parts. Mater Des. 2016;109:209–218. doi: 10.1016/j.matdes.2016.07.080.