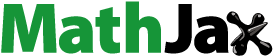
Abstract
Fabrication of solid biopolymer electrolytes (SBEs) was mainly to cater the drawbacks of lithium-ion electrolytes in terms of leakage, high cost, and toxic material. In this present work, solid biopolymer electrolytes system comprises of 2-Hydroxyethyl Cellulose (2-HEC) as polymer host, and various weight percentage (wt.%) of Ammonium Formate (AF) as ionic doping salt are produced by using solution casting technique. The relation between structural and ionic conductivity of 2-HEC/AF was analyzed and investigated by using X-Ray Diffraction (XRD), Fourier-Transform Infrared Spectroscopy (FTIR), and Electrical Impedance Spectroscopy (EIS). Through the analysis of XRD, it can be observed that the amorphous region of SBEs increases as the concentration of AF salt increases. The amorphous region of SBEs can be confirmed due to the interaction of salt concentration and polymer. However, in this present work, the broadest peak in XRD of the sample does not necessarily correlate with the highest conductivity among the samples. The interaction between polymer-salt complexes is proved through FTIR spectrum at the range of 700 cm−1 to 1300 cm−1. The highest ionic conductivity of 2-HEC/AF achieved is 2.40 × 10−3 S/cm at room temperature for SBEs containing 40 wt.% of AF. The findings from this study made it clear that the concentration of Ammonium Formate salt affects the increase in ionic conductivity.
1. Introduction
Due to the effects of fossil fuels towards climate change, environment, and health issues make transition of fossil fuels to renewable energy is necessary. Thus, adoption of battery storage system is key for this. Batteries are the essential component of modern technology and in human’s daily life such as for cell phones, household appliances and even in electric vehicles. Battery is known as electrochemical device as it generates electrical energy through the conversion of chemical energy [Citation1]. Anode, cathode, and electrolyte are the three primary components consisting in a battery which aid in the flow of chemical processes in the battery. The electrolyte, which is material containing free ions that acts as an electrically conductive medium, is one of the important components in the battery [Citation2]. Electrolyte is an ionic solution that can conduct electric current by dissociating into two charged ions which are cations and anions which enhance the performance of electrodes in the energy storage [Citation3]. Electrolytes can be divided into three types which are liquid electrolytes, gel electrolytes and solid electrolytes. Liquid electrolyte has been widely developed and used for a long time such as in lithium-ion batteries, as it provides substantial advantages in terms of its conductivity and excellent electrode-electrolyte contact. In lithium-ion batteries, high purity electrolytes are important and usually comprises of lithium salt, for instance lithium hexafluorophosphate (LiPF6) is often dissolved in a highly polar organic solvent mixture such as ethylene carbonate (EC) in liquid electrolytes due to its high ionic conductivity and stability [Citation4]. However, it still has significant drawbacks in terms of flammability, possible hazards, liquid leakage, corrosion, and limited chemical stability due to the reaction between solutions with the unstable anode [Citation5].
Based on this viewpoint, many researchers are developing new and safer materials by exploring the potential use of solid polymers as polymer electrolytes to replace the conventional liquid-based electrolytes. One of polymer electrolytes development is Solid Biopolymer Electrolytes (SBEs) that have been studied by many researchers for numerous years. When it comes to developing safe and high-energy batteries, SBEs with high stability show significant potential over conventional liquid electrolytes, which typically encounter irreversible breakdown, high flammability, and leakage concerns. SBEs shows advantages as a safer electrolyte due to its solid materials since it is in solid physical state, high in electrochemical stability and significantly higher transference number which aid in the issue of battery polarization [Citation6]. Biopolymer electrolyte which comes from natural resources have been used to replace the synthetic (petroleum-based) polymer and studies has shown several great properties in terms of biodegradable, non-toxic, eco-friendly and it is affordable since it come from replenished natural resources compared to synthetic petroleum-based polymer, which takes billions of years to be created which currently in rapid depletion [Citation7]. Lignin, cellulose, agar, carrageenan, chitosan, starch, alginates, guar gum, and xanthan gum are the most researched materials in the context of battery electrolyte as each of it has its own characteristics [Citation8]. Based on previous research, cellulose-based solid-state electrolyte system is proven to have nontoxicity, flexibility, and biocompatibility film properties [Citation9].
To be a great electrolyte, the host polymer must have excellent characteristics in terms of mechanical properties, electrochemical properties, and chemical stability [Citation10]. The host polymers with high number of polar groups and have amorphous state are preferable in polymer electrolytes as it will increase the mobility of ions [Citation11]. In recent years, interest in cellulose material is starting to increase in the host polymer application due to the acknowledgement of its properties. The structure properties of cellulose which made in form of helical microfibrils that contain both crystalline and amorphous areas make them one of the suitable host polymers in electrolyte [Citation12]. This structure plays an important role for cellulose’s unique features which give high hydrophilicity, greater energy and biocompatibility due to the presence of -OH groups [Citation13, Citation14]. However, the conductivity of pure cellulose-based film is reportedly low and does not indicate electrical conductivity due to its non-conductive behaviour [Citation15]. One of the well-known techniques to cater for this problem is by adding ionic dopants that will enhance the polymer conductivity due to the ion attraction by allowing the exchange of free mobile ions between the host polymer and salt [Citation16]. The ammonium salt is reported by researchers as a great ionic dopant for electrolyte and other chemical applications [Citation17]. Ammonium salt has crystalline structures which have high portability towards the exchange of ions compared to strong salts or strong acids which optimize its performance as ionic dopant [Citation18]. In addition, ammonium salt can enhance the performance of electrolyte by providing a higher number of mobile ions while facilitating the movement of charge carriers in polymer thus increasing the ionic conductivity [Citation19].
In this research, cellulose-based biopolymer, which is 2-Hydroxyethyl Cellulose (2-HEC) is used as the host in the polymer-salt electrolyte system. 2-HEC is a derivative from cellulose-based which can be easily obtained from cell walls of plant and its structure is reported to have a high ion affinity as it contains a lot of hydroxyl functional groups which act as ion attachments [Citation11, Citation17]. Meanwhile, Ammonium Formate is used as the ionic dopant due to its structure where one of the hydrogen ions (H+) is loosely attached to its ammonium ion, NH4+ making its easy to transfers via hopping mechanism to the coordinating sites in the host polymer thus, increase the conductivity [Citation18]. To the best of the author’s current knowledge, there have been no prior studies conducted regarding on bio-based 2-Hydroxyethyl Cellulose polymer electrolytes doped with Ammonium Formate as proton conductor.
2. Materials and methods
2.1. Preparation of 2-hydroxyethyl cellulose ammonium formate (2-HEC/AF) biofilm electrolytes
A total of 2 grams of 2-Hydroxyethyl Cellulose (Sigma Aldrich) were dissolved in 100 mL of distilled water until it fully dissolved. Then, a varied amount of Ammonium Formate salts (5 wt.% to 45 wt.%) were added into the 2-HEC solution and stirred continuously using magnetic stirrer until a homogenous solution was formed. The amount of AF used was calculated using percent by weight formula shown in EquationEquation (1)(1)
(1) . One (1) undoped sample was prepared as a control sample in this experiment. The homogenous 2-HEC/AF solution was then poured into petri dishes and covered to prevent sample contamination and left to dry at room temperature until the formation of thin film. Then, the dried thin films were transferred into desiccators containing silica gel before further analysis. shows the translucent, flexible, and free-standing thin film that was obtained from the solution casting technique where the physical thin film is observed in a flexible and free-standing state. The composition for 2-HEC/AF and their designations were listed in .
Table 1. List of composition and designation for 2-HEC/AF.
Ammonium Formate
(1)
(1)
2.2. Characterization of 2-hydroxyethyl cellulose ammonium formate (2-HEC/AF) biofilm electrolytes
2.2.1. X-ray diffraction (XRD)
The X-Ray Diffraction (XRD) method was performed to determine SBE’s nature if the biopolymer compounds were in amorphous or crystalline state. Sample with size of 2 cm × 2 cm was placed in the sample holder with fixed position before the data was being collected. The Rigaku MiniFlex 600 was used to conduct the X-Ray diffraction (XRD) at 2θ angle, range between 10° to 80° initiated by CuKα radiation.
2.2.2. Fourier transform infrared spectroscopy (FTIR)
The infrared spectra and the structure of solid biopolymer electrolyte were further studied as each sample was tested by using FTIR spectrometer brand Thermo Nicolet iS50 with attenuated total reflection (ATR) attachment and connected to a computer. After the background spectrum was collected, the tested sample with size of 1 cm × 1 cm was placed on the germanium crystal probe of the ATR. At room temperature, the FTIR spectra wavelength range from 700 cm−1 to 4000 cm−1 with a resolution of 4 cm−1 were collected and recorded.
2.2.3. Electrical impedance spectroscopy (EIS)
The conductivities and dielectric properties of 2-HEC/AF were measured using the Electrical Impedance Spectrometer brand HIOKI IM 3570-50 LCR Hi-Tester in the frequency range of 50 Hz to 1 MHz. The SBE films were cut using sharp razor blade into 2.0 cm diameter disk and sandwich in the sample holder of two stainless steel electrodes with spring pressure. The HIOKI IM 3570-50 LCR Hi-Tester was connected to a computer. From the data obtained, a graph of negative imaginary impendence (-Zi) versus real impedance (Zr) also known as Cole-Cole plot were plotted. In addition, both the horizontal and vertical axes of the graph must be in the same scale and the bulk resistance (Rb) was obtained through the intersection of high and low frequency. The sample’s ionic conductivity was calculated using EquationEquation (2)(2)
(2) below where t is thickness of the electrolytes, A is area of electrode-electrolyte (cm2) and Rb is bulk resistance [Citation20, Citation21].
(2)
(2)
3. Results and discussions
3.1. XRD analysis
depicts the XRD spectrum of pure 2-Hydroxyethyl Cellulose (2-HEC) and pure Ammonium Formate (AF) salt. The pure 2-HEC can be seen through the broad peak which is located at 2θ = 21.36°. According to Asnawi and co-workers, the broad diffraction peak of the polymer electrolyte is associated to its amorphous nature [Citation22]. The XRD spectrum for AF salt shows crystalline peaks located at 2θ = 22.36°, 28.44°, 31.02°, 36.88°, 44.78° and 46.98°. These patterns illustrate the crystallinity phase of a pure AF salt.
XRD spectra patterns of 2-HEC/AF SBE samples with variation of AF salt concentration are shown in . The centre peak of AF0 is located at 2θ = 22.1° and peak shifting occured to the left as the addition of salt increases. The centre peak of AF40 can be seen located at 2θ = 20° and it depicts the broadening of the amorphous hump. The intensity of the broad peak decreases with the addition of salt may be due to the interaction of polymer and salt [Citation23]. The trend in this XRD pattern shows that the hydrogen ion (H+) from Ammonium Formate have formed an interaction with hydroxyl group (-OH) of 2-HEC structure through the direct transport mechanism in polymer chains. Thus, the samples of 2-HEC/AF exhibit an identical pattern to pure 2-HEC and shows that the amorphous phase of the samples were increased. It has been reported widely that SBE samples with an amorphous phase are crucial because it supports the transport of (H+) ions of the salt to the polymer resulting to the ionic conductivity enhancement [Citation24, Citation25].
In addition, from the XRD results, it can be observed that the samples peaks shifted to the left alongside the addition of salts. This peaks shift indicates that lattice strain occurred in the polymer regions where the presence of hydrogen ion from salt disrupted the structure of polymer matrix thus increase the amorphousness which allowing the contribution of ion for conductivity [Citation26]. The addition of salt provides a lot more spaces in polymer as the polymer structure is in unordered structure resulting in peak shifted to the left due to expansion of lattice strain in polymer [Citation27]. Thus, it can be seen that lattice strain induced the amorphousness of the sample, thereby contributing to enhancement of ionic conductivity.
3.2. FTIR analysis
illustrates the FTIR spectra for pure 2-HEC and pure AF salt respectively. Based on (a), the FTIR spectrum for pure 2-HEC shows the broad peak that be seen located at 3390 cm−1 which attributed as the stretching band of O–H group [Citation16, Citation18]. The stretching mode of C–H can be assigned at 2921 cm−1 for axial symmetric and 2867 cm−1 for symmetric stretching peak [Citation20]. The bending vibration mode of –CH2 can be seen located at 1448 cm−1 meanwhile at 1353 cm−1 and 1311 cm−1 can be classified as bending vibration of hydroxyl group, OH [Citation21]. In addition, the peak band at 1049 cm−1 and 884 cm−1 were assigned as C–O stretching mode and C–O–C stretching vibration of 2-HEC [Citation28, Citation29].
(b) shows the FTIR spectra for AF salt, the broad peak located at 3176 cm−1 and 2982 cm−1 were assigned as the strong stretching band of N-H from the salt itself [Citation24]. Besides, C-H stretching bond can be observed at the peak of 2791 cm−1 [Citation30]. The peak located at 1559 cm−1 can be assigned to the stretching of C=O of AF salt meanwhile peak at 1452 cm−1 is corresponding to stretching of -NH [Citation31]. The band at 1339 cm−1 can be ascribed to the vibration of C-H bending from formate ions and a sharp peak located at 771 cm−1 represents the bending vibration of O=C–O [Citation32].
The FTIR spectrums for AF0, AF5, and AF40 SBEs samples at range of 700 cm−1 to 1300 cm−1, are shown in . From the figure, it can be observed that vibration mode of C–O exhibited by AF0 were located at 1045 cm−1 and the wavenumber increases as the addition of salt increases. It shows changes in wavenumber as the number increases from 1050 cm−1 for AF5 to 1059 cm−1 for AF40. The increase in wavenumber or known as blue shift shows that the changes of bond length occurred at the polymer chain of 2-HEC due to the dopant salt [Citation33, Citation34]. The change in the C–O bond length in polymer occurred due to the hydrogen bonding from the ammonium ion (NH4+) with the structure of 2-HEC which will lead to the increase in ionic conductivity in terms of providing a pathway for the migration of ions [Citation35]. This interaction between 2-HEC and AF salt resulted in the changes of wavenumber for C–O stretching vibration in backbone of cellulose due to the modification in cellulose structure. The wavenumber of 764 cm−1 for vibration mode of O=C–O has increased in intensity, however there were no shifted occurred.
(a) depicts a clearer region of the presence of dopant salt, AF in the polymer host. complexation for 2-HEC and AF salt which can be seen located at range of 3110 cm−1 to 3350 cm−1. It can be observed that the intensity vibration of N-H peak has increased with the addition of AF salt. In (b), at range of 1500 cm−1 – 1650 cm−1, it can be observed that the stretching vibration mode of C=O has shifted from higher to lower wavenumber which is 1589 cm−1 for AF5 sample compared to AF40, 1578 cm−1. The peak intensity increases as the addition of AF salt concentration increases in the polymer host system, 2-HEC. The changes occurred at this peak also caused by interaction of the dipole-dipole forces that forced the attraction of electrons from salt towards 2-HEC resulting in a changed of intensity [Citation36]. By adding 45 wt.% AF salt concentration, the peak start to shifted back to higher number due to the excessive amount of AF salt which block the movement of ion at the polymer system and resulting in the decrease of ionic conductivity of samples as reported by Ghazali and co-workers [Citation31]. In (c), the bending mode of O-H can be seen located at 1350 cm−1 where the intensity of the peak band increases along with the addition of AF salt. The band later shifted to the left with the addition of 25 wt.% of salt. Thus, it shows that AF salt is presence in the polymer.
3.3. EIS analysis
Based on the impedance analysis, the Cole-Cole plots of 2-HEC/AF samples were obtained and the bulk resistance, Rb was determined for each sample with varied Ammonium Formate concentration. The bulk resistance (Rb) of the sample is often defined as the intercept along the real axis on the small frequency side [Citation37]. The value of Rb for the samples was determined at the intersection of the high frequency and the low frequency region on x-axis. shows the Cole-Cole plot for pure sample of AF0 and sample of AF40 respectively and the value of bulk resistance of the samples were recorded in . Based on AF0, the spectra show two distinct sections of the spectrum which are partial semicircle at high frequency region and spike line at low frequency region. Meanwhile, for AF40, it only shows an inclined spike. Increasing AF salt lead in narrowing the semicircle arc, indicating a decrease in resistivity of SBE [Citation38].
Table 2. Values of thickness, bulk resistance, area, and ionic conductivity for sample AF0 until AF45.
The ionic conductivity graph can be divided into two sections in order to best describe the ionic conductivity of the SBE samples. Based on , it can be observed that the addition of the concentration of the salt increases gradually with the conductivity of the SBE samples in region 1. This steady rise in ionic conductivity was caused by the increase in the number density (n) and the mobility of charge carrier ions (µ) [Citation32, Citation39]. The highest ionic conductivity achieved at room temperature is 2.40 × 10−3 S/cm for AF40. The present study shows greater conductivity performance in contrast with previous electrolyte systems which tabulated in . The table highlights the current work SBE remarkable gains compared to other SBE thus emphasize the current work potentials for energy storage application. The increases of ionic conductivity of SBEs samples were due to the increase of H+ ion from the addition of salt which produced more free mobile ions to the polymer host system [Citation38]. The increase in conductivity is related to their amorphousness as mentioned in XRD analysis before, where the amorphous structure increases the ionic conductivity of the samples. The conductivity of samples improved as H+ increases which supports the ion movement where it can be seen through the increment of -NH peak intensity in . In region 2, the conductivity decreases after it reaches its limit of AF40. According to Muthukrishnan and co-workers, the crowded formation of complexation NH4+ making the ions to clump together which reduced the ionic mobility of H+ and caused the decrease in ionic conductivity for sample AF45 [Citation18]. The Impedance test for AF50 was not taken as the sample was physically unstable and in jelly-like texture therefore was not used for testing.
Table 3. Conductivity of polymer electrolytes doped with various ammonium salts.
4. Conclusion
In conclusion, a solid and flexible biopolymer electrolyte thin film of 2-Hydroxyethyl Cellulose doped with Ammonium Formate was successfully fabricated by using solution casting technique. The physical appearance for the SBEs were transparent and behaved like plastic behaviour. From the electrical analysis, the ionic conductivity of 2-HEC/AF samples increases until it reaches 40 wt.% of Ammonium Formate, 2.40 × 10−3 S/cm. Due to its outstanding conductivity by using cellulose-based materials, this present solid biopolymer electrolyte has the potential to be used in energy storage applications such as batteries. However, the conductivity can be improved by introducing plasticizer into the polymer system where it acts as a catalyst that causes more ion dissociation, which opens more charge carriers for ion transport in the electrolyte hence increase in ionic conductivity.
Acknowledgements
The authors would like to acknowledge officers and laboratory staff of Applied Physics department at the Faculty of Science and Technology, USIM. Special thanks to Advanced Materials Team (AMT) members for the consultations and supports provided.
Data availability statement
The data that support the findings of this study are available from the corresponding author, M.I.N. Isa, upon reasonable request.
Disclosure statement
The authors declare that there is no conflict of interest in the design of study, in the data collection, analyses, or interpretation data, in the writing of the manuscript, or in the decision in publishing the results.
Additional information
Funding
References
- Schmidt-Rohr K. How batteries store and release energy: explaining basic electrochemistry. J Chem Educ. 2018;95(10):1801–1810. doi: 10.1021/acs.jchemed.8b00479.
- Abe H, Kubota M, Nemoto M, et al. High-capacity thick cathode with a porous aluminum current collector for lithium secondary batteries. J Power Sources. 2016;334:78–85. doi: 10.1016/j.jpowsour.2016.10.016.
- Iqbal MZ, Zakar S, Haider SS. Role of aqueous electrolytes on the performance of electrochemical energy storage device. Electroanal Chem. 2020;858:113793. (doi: 10.1016/j.jelechem.2019.113793.
- Horowitz Y, Schmidt C, Yoon D, et al. Between liquid and all solid: a prospect on electrolyte future in lithium‐ion batteries for electric vehicles. Energy Tech. 2020;8(11):2000580. doi: 10.1002/ente.202000580.
- Yao Y, Zhao X, Razzaq AA, et al. Mosaic rGO layers on lithium metal anodes for the effective mediation of lithium plating and stripping. J Mater Chem A. 2019;7(19):12214–12224. doi: 10.1039/C9TA03679B.
- Zhao R, Wu Y, Liang Z, et al. Metal–organic frameworks for solid-state electrolytes. Energy Environ Sci. 2020;13(8):2386–2403. doi: 10.1039/D0EE00153H.
- Fuzlin AF, Saadiah MA, Yao Y, et al. Enhancing proton conductivity of sodium alginate doped with glycolic acid in bio-based polymer electrolytes system. J Polym Res. 2020;27(8):207. doi: 10.1007/s10965-020-02142-0.
- Lizundia E, Kundu D. Advances in natural biopolymer‐based electrolytes and separators for battery applications. Adv Funct Mater. 2020;31(3):2005646. doi: 10.1002/adfm.202005646.
- Diana MI, Selvin PC, Selvasekarapandian S, et al. Investigations on Na-ion conducting electrolyte based on sodium alginate biopolymer for all-solid-state sodium-ion batteries. J Solid State Electrochem. 2021;25(7):2009–2020. doi: 10.1007/s10008-021-04985-z.
- Teo LP, Buraidah MH, Arof AK. Development on solid polymer electrolytes for electrochemical devices. Molecules. 2021;26(21):6499. doi: 10.3390/molecules26216499.
- Rayung M, Aung MM, Azhar SC, et al. Bio-based polymer electrolytes for electrochemical devices: insight into the ionic conductivity performance. Materials (Basel). 2020;13(4):838. doi: 10.3390/ma13040838.
- Zhang H, Zhang F, Yuan R, Applications of natural polymer-based hydrogels in the food industry. In: Chen Y, editor. Hydrogels based on natural polymers. Elsevier; 2020. p. 357–410. doi: 10.1016/b978-0-12-816421-1.00015-x.
- Ghazali NM, Samsudin AS. Progress on biopolymer as an application in electrolytes system: a review study. Mater Today: Proc. 2022;49:3668–3678. doi: 10.1016/j.matpr.2021.09.473.
- Chen C, Xi Y, Weng Y. Recent advances in cellulose-based hydrogels for tissue engineering applications. Polymers (Basel). 2022;14(16):3335. doi: 10.3390/polym14163335.
- Xie P, Ge Y, Wang Y, et al. Mechanically enhanced nanocrystalline cellulose/reduced graphene oxide/polyethylene glycol electrically conductive composite film. Nanomaterials (Basel). 2022;12(24):4371–4371. doi: 10.3390/nano12244371.
- Zainuddin NK, Rasali NMJ, Mazuki NF, et al. Investigation on favourable ionic conduction based on CMC-K carrageenan proton conducting hybrid solid bio-polymer electrolytes for applications in EDLC. Int J Hydrogen Energy. 2020;45(15):8727–8741. doi: 10.1016/j.ijhydene.2020.01.038.
- Ramlli MA, Bashirah NAA, Isa MIN. Ionic conductivity and structural analysis of 2-hyroxyethyl cellulose doped with glycolic acid solid biopolymer electrolytes for solid proton battery. IOP Conf Ser: Mater Sci Eng. 2018;440:012038. doi: 10.1088/1757-899X/440/1/012038.
- Muthukrishnan M, Shanthi C, Selvasekarapandian S, et al. Impact of ammonium formate (AF) and ethylene carbonate (EC) on the structural, electrical, transport and electrochemical properties of pectin-based biopolymer membranes. Ionics. 2021;27(8):3443–3459. doi: 10.1007/s11581-021-04106-w.
- Zheng W, Hu X, Wu M, et al. Advanced ammonium salt materials for electrochemical energy storage: recent progress and future perspectives. Chem Eng J. 2023;454:140194–140194. doi: 10.1016/j.cej.2022.140194.
- Fuzlin AF, Rasali NMJ, Samsudin AS. Effect on ammonium bromide in dielectric behavior based alginate solid biopolymer electrolytes. IOP Conf Ser: Mater Sci Eng. 2018;342:012080. doi: 10.1088/1757-899X/342/1/012080.
- Abdullah AM, Aziz SB, Brza MA, et al. Glycerol as an efficient plasticizer to increase the DC conductivity and improve the ion transport parameters in biopolymer based electrolytes: XRD, FTIR and EIS studies. Arabian J Chem. 2022;15(6):103791. doi: 10.1016/j.arabjc.2022.103791.
- Asnawi ASFM, Aziz SB, Brevik I, et al. The study of plasticized sodium ion conducting polymer blend electrolyte membranes based on chitosan/dextran biopolymers: ion transport, structural, morphological and potential stability. Polymers (Basel). 2021;13(3):383. doi: 10.3390/polym13030383.
- Abirami M, Saratha R, Shilpa R, et al. Preparation and characterization of guar gum-based solid biopolymer electrolyte doped with lithium bis(trifluoromethanesulphonyl)imide (LiTFSI) plasticized with glycerol. Bull Mater Sci. 2020;43(1):254. doi: 10.1007/s12034-020-02218-z.
- Hafiza MN, Isa MIN. Correlation between structural, ion transport and ionic conductivity of plasticized 2-hydroxyethyl cellulose based solid biopolymer electrolyte. J Membr Sci. 2020;597:117176. doi: 10.1016/j.memsci.2019.117176.
- Abdullah MM. Consequences of frequency and temperature on the ac-conductivity in ε-GaSe semiconductor single crystal. Results Phys. 2021;25:104220. doi: 10.1016/j.rinp.2021.104220.
- Yang X, Liu J, Pei N, et al. The critical role of fillers in composite polymer electrolytes for lithium battery. Nano-Micro Lett. 2023;15(1):74. doi: 10.1007/s40820-023-01051-3.
- Owen L, Jones N. Lattice distortions in high-entropy alloys. J Mater Res. 2018;33(19):2954–2969. doi: 10.1557/jmr.2018.322.
- El-Sakhawy M, Kamel S, Salama A, et al. Preparation and infrared study of cellulose based amphiphilic materials. Cellulose Chem. Technol. 2018;52(3–4):193–200. http://www.cellulosechemtechnol.ro/pdf/CCT3-4(2018)/p.193-200.pdf
- Ayouch I, Kassem I, Kassab Z, et al. Crosslinked carboxymethyl cellulose-hydroxyethyl cellulose hydrogel films for adsorption of cadmium and methylene blue from aqueous solutions. Surf Interf. 2021;24(101124):101124–101124. doi: 10.1016/j.surfin.2021.101124.
- Liu J, Dong C, Wei D, et al. Multifunctional antibacterial and hydrophobic cotton fabrics treated with cyclic polysiloxane quaternary ammonium salt. Fibers Polym. 2019;20(7):1368–1374. doi: 10.1007/s12221-019-1091-2.
- Ghazali NM, Fuzlin AF, Saadiah MA, et al. Studies on H + ions conducting bio-polymer blend electrolyte based on alginate-PVA doped with NH4NO3. J Non-Cryst Solids. 2022;598:121939. (doi: 10.1016/j.jnoncrysol.2022.121939.
- Sohaimy MIH, Isa MIN. Proton-conducting biopolymer electrolytes based on carboxymethyl cellulose doped with ammonium formate. Polymers (Basel). 2022;14(15):3019. doi: 10.3390/polym14153019.
- Kim Y-H, Hwang MS, Kim HJ, et al. Infrared spectroscopy study of low-dielectric-constant fluorine-incorporated and carbon-incorporated silicon oxide films. J Appl Phys. 2001;90(7):3367–3370. doi: 10.1063/1.1402152.
- Tomar D, Rana B, Jena KC. The structure of water–DMF binary mixtures probed by linear and nonlinear vibrational spectroscopy. J Chem Phys. 2020;152(11):114707. doi: 10.1063/1.5141757.
- Wang Y, Wu L, Lin Z, et al. Hydrogen bonds enhanced composite polymer electrolyte for high-voltage cathode of solid-state lithium battery. Nano Energy. 2022;96:107105–107105. doi: 10.1016/j.nanoen.2022.107105.
- Tian Z, Zou Y, Liu G, et al. Electrolyte solvation structure design for sodium ion batteries. Adv Sci (Weinh). 2022;9(22):e2201207. doi: 10.1002/advs.202201207.
- Devi SS, Chary AS. Cole-Cole analysis and electrical conduction of nano (1-X)Ba(No3)2-Xkno3. RJC. 2021;14(03):1959–1964. doi: 10.31788/RJC.2021.1436397.
- Ramlli MA, Mohamad Isa MIN, Kamarudin KH, Universiti Malaysia Terengganu. 2-Hydroxyethyl cellulose-ammonium thiocyanate solid biopolymer electrolytes: ionic conductivity and dielectric studies. JSSM. 2022;17(7):121–132. doi: 10.46754/jssm.2022.07.009.
- Majumdar S, Ray R. Ionic conduction and charge carrier relaxation in chitosan acetate based solid biopolymer electrolyte embedded with LiClO4. J Polym Res. 2021;28(5):157. doi: 10.1007/s10965-021-02509-x.
- Abd Majid SN, Ishak AQ, Nik Ali NA, et al. The effect of ammonium bromide on methylcellulose biopolymer electrolytes for electrical studies. SSP. 2021;317:426–433. doi: 10.4028/www.scientific.net/SSP.317.426.
- Rehila Karolin Blesstina S, Mathavan T, Buvaneshwari P, et al. Conducting behaviour of a novel solid biopolymer electrolyte for electrochemical application. Ionics. 2023;29(9):3437–3450. doi: 10.1007/s11581-023-05087-8.
- Nithya M. Evaluation of conducting properties of biopolymer electrolyte K-Carrageenan with the effect of three different ammonium salts. Enenstrg. 2023;3(2):42–44. doi: 10.52924/YTMK4887.