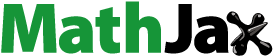
ABSTRACT
Sustainable agricultural development is a topic of global concern aiming at satisfying food demand of the growing population while reducing impact on environment. Circular agriculture has become a promising development pattern with the advantages of complementing environmental resources, replacing feedback from outside system, and processing waste of bottom of system, etc. This study combined economic analysis, life cycle assessment and emergy accounting to evaluate sustainability of an integrated maize-pig system (IS) driven by indigenous microbes in Northeast China, compared with separated maize and pig system (SS). IS reduced input of concentrate feed, coal, vaccines, disinfectants, fertilizer and labor by 14.35%, 100%, 46.11%, 100%, 60.1% and 60.8% driven by indigenous microbes. Economic analysis results showed that IS has 82.88% higher profit than that of SS. The sum of potential environmental impacts of IS was lower than SS for 80.65% according to lifecycle assessment. The emergy results revealed that IS performed better sustainability. Internal feedback emergy for fertilizer and local resources for maize straw increasing of IS significantly improved emergy efficiency. The reduction resulted in nonrenewable resources decreased 33.01% than that of SS, and increased renewable resources by 67.81%, which reduced environmental load ratio of IS by 60.08% of IS. Meanwhile, these factors were the primary factors generating higher sustainability of IS. Overall, the integrated maize-pig system driven by indigenous microbes in this study can effectively alleviate the problems caused by agricultural waste and promote sustainable agricultural development.
Introduction
With the increase in population, the intensive agriculture corresponding to the increase in food demand has also increased greatly in recent decades (Muller et al. Citation2017; Sharma et al. Citation2020). Nevertheless, resource waste and chemical fertilizer overuse caused by intensive agriculture have aggravated degradation of soil productivity and environmental pollution including greenhouse gas (GHG) emissions, eutrophication of land and waterbodies (Li et al. Citation2021; Steffen et al. Citation2015; Wang et al. Citation2021b; Yang et al. Citation2018; Zhang et al. Citation2009). COVID-19 threatens China’s food security and reduced reliance on food imports, putting high demand for high-quality animal protein at risk (Bai et al. Citation2020). Yet, these challenges are further exacerbated by excessive fertilizer implication and intensive livestock rearing to ensure yield. It is, therefore, crucial to innovate agricultural development models to accelerate agricultural sustainable construction, responding to no poverty, clean water, and clean energy of Sustainable Development Goals (SDGs) (FAO Citation2015; Adegbeye et al. Citation2020). Circular agriculture has been developed against agricultural challenges at home and abroad. AgroCycle project in European Union (Toop et al. Citation2017; AgroCycle Citation2017), the program of “Agriculture, nature and food: valuable and connected” in the Netherlands (Ministry of Agriculture, Nature and Food Quality of Netherlands Citation2018), “Conserving and saving resources, streamlining the production process, recycling industrial chains and waste resources” in China (Fan et al. Citation2018b) have been proposed to construct sustainable agricultural waste utilization approaches to improve eco-economic benefits.
Currently, multiple technologies and theories of circular agriculture have been developed in China, primarily containing energy conservation and emission reduction of agriculture, agricultural production system coupling, and biomass energy industry (biogas digester) (Fan et al. Citation2018a; Xian et al. Citation2020; Xiong et al. Citation2020). Various circular agricultural models and technologies have been employed to address the problems of agricultural waste through years of practice. Integrated planting and breeding is an important measure to solve agricultural waste problems at home and abroad, such as the “pig-methane-vegetable” model, “rice-shrimp” model, and “cattle-biogas-vegetable” models (Chen, Chen, and Sun Citation2010; Wang et al. Citation2021a; Wu et al. Citation2013). Moreover, the extension of the industrial chain has formed a new agricultural production model, which achieves crop-animal-biogas-fertilizer-soil, indicating fitted industrial chain can reduce GHGs for the whole system (Xian et al. Citation2020). Technologies such as intercropping rotation, returning straw to the field, organic fertilizer utilization, three-dimensional planting, and manure treatment are gradually being considered as circular agriculture. Anaerobic digestion technology and microbial biotechnology are the main technologies for agricultural waste management (Angouria-Tsorochidou, Teigiserova, and Thomsen Citation2021; Nielsen Citation2017).
Microbial fermentation technology in circular agriculture has developed rapidly to decompose agricultural waste over the past decades. Anaerobic digestion, composting, co-composting, vermicomposting, and biochar production and activation are the major green methods, which are widely adopted for bio-waste transformation into valuable organic manure (Ravindran et al. Citation2021). Anaerobic digestion is an effective method to recover energy from bio-waste and reproduce valuable biogas, which is used in large-scale biogas plant construction (Chen et al. Citation2017a; Li, Chen, and Wu Citation2019). Bacteroidetes, Firmicutes, Proteobacteria, and so on influence degradation process and substrate maturity in composting and co-composting process, as well as large and bench-scale composting units have been established worldwide (Malamis et al. Citation2017). Agricultural waste recycling benefits from microbial fermentation technology, manifesting in that the microbial community structure changes corresponding to fermentation status (Peng, Nges, and Liu Citation2016). Microbial-driven maize straw and pig manure combined with liquid biogas recycling can increase methane production and agricultural waste utilization, with 81.9% liquid digestate reduction, 70.4% COD reduction, and 65.4% water saving (Zheng et al. Citation2020).
Overall, previous studies have tried to explore a scientific and effective recycling model to reduce the impact of agricultural waste on the environment and improve resource utilization while ensuring food safety and economic benefits. Nevertheless, most of the circular agriculture models are proposed by universities and scientific research institutes recently. Meanwhile, most microorganisms are cultivated in the laboratory, that is, the “factory” bacteria. This study used a case of a circular maize-pig system with indigenous microbes-driven in Northeast China to explore the sustainability of integrated planting and breeding system. Economic analysis, life cycle assessment (LCA), and emergy accounting (EME) were conducted to evaluate the economic profit, potential environmental impacts, and emergy efficiency of maize-pig system. The objectives of this study were to (1) quantify economic profit, potential environmental impacts, and emergy efficiency from integrated maize-pig system (IS) and separated system (SS) between maize production and pig rearing system; (2) compare the differences of economic and ecological performance between integrated and separated systems and analyze their contribution factors; (3) propose suggestions for improvement. This study will help stakeholders gain a new understanding of the indigenous microbes-driven integrated maize-pig system.
Materials and methods
System description
Research site
Yunfeng Agricultural and Animal Husbandry Cooperative (Yunfeng Cooperative) is located in Guojiazhuang village (125°52ʹE, 44°50ʹN) of Changchun City, Jilin Province, China, conformed by 100 production employees. It is in the northeast of China, with an annual average temperature of 5.5°C and total annual precipitation of 573 mm (mostly from June to August). The enterprise adds a “fermentation bed” to cultivate beneficial microorganisms and then uses the microorganisms to decompose pig manure into organic fertilizer, and the microorganisms themselves turn into bacterial protein, which becomes the protein feed required by pigs. This technology is known as the deep-litter-system, breeding pig on litter, or in situ decomposition of pig manure (Morrison, Johnston, and Hilbrands Citation2007). The enterprise contains 16 pigsties, breeding 1,000 pigs, and consumes 3,000 tons of corn stalks each year for food and as bedding for the fermentation bed. The output of the planting base can be used as feed. The fattening period of one pig is more than 8 months, and livestock manure combined with fermented bedding material (maize straw) can be directly used as organic fertilizer to return to the field. The technical flow chart is shown in , with a pig rearing pattern diagram on the left and an integrated maize-pig agriculture flow chart on the right. In the integrated maize-pig system, the fermentation bed waste can be used as organic fertilizer to return to the field, and the maize straw can be directly used as feed under the drive of microorganisms. Meanwhile, microbe picked from the local mountain forest needs to be cultivated in an indoor lab. In addition, joining the indigenous microbial agents in drinking water contributes to the consumption of maize straw. The environmental factors of the corresponding separation system referred to Guojiazhuang Village.
Characteristics of the systems
summarizes the system emergy flow of a separated maize-pig system (SS) and an integrated maize-pig system (IS) from the “input-output” perspective and shows the system boundary. ) shows the separated system including conventional maize production system (MS) and conventional pig rearing system (PS). Purchased resources including seeds, fertilizer, pesticide, electricity, diesel, and labor were from outside MS. PS contained feed processing, feeding management, medical breeding, and transportation. Diesel, electricity, coal, labor, vaccine, forage, and equipment fund came from outside the system. ) is an integrated maize-pig system driven by indigenous microbes of Yunfeng Cooperative. Maize production, feed processing, feeding management, medical breeding, and transportation were included in the system. Maize and straw provided forage to the pigs, and pig manure was directly used as organic fertilizer and returned to the maize field through a 5-year microbial decomposition and fermentation. The standard ecological pig house covered an area of 380 m2 and raised 150 pigs in Yunfeng Cooperative, responding to 2.14 ha corn field for feed. The size of the field corresponded to the amount of straw needed for the pig system. The functional unit of this study was 150 pigs in all of economic analysis, emergy accounting, and life cycle assessment. See Appendix A for full names of all relevant abbreviations in the article
Figure 2. System emergy flow of separated and integrated maize-pig system.

Differences were significant in purchased resources input, output, and potential environmental impacts between SS and IS, shown in red lines in . In IS, there was no pesticide in maize planting due to its green pollution-free planting and fermented bedding material replaced part of chemical fertilizers that was applied to farmland. Spraying the medicament with indigenous microbes into the maize straw could be directly used for pig feed, which saved concentrated feed to a certain extent and improved the utilization of waste resources. Purchased resources such as coal and electricity with fermentation technique were much lower than ordinary pig rearing because the fermentation bed generated heat during microbial fermentation. Also, maize yield, maize straw, and pork of the SS were lower than that of IS. The integrated system contained much lower groundwater and coal usage resulting from no waste management, which led to less labor input for the whole system. Detailed raw data are shown in .
Table 1. Agricultural input and output inventory lists of separated and integrated maize-pig systems.
Data collection
The objective of this study was to evaluate sustainability for an integrated maize-pig system driven by indigenous microbes in Northeast China from the aspects of economic profits, emergy accounting, and potential environmental impacts. Four parts of raw data of environmental resources, purchased resources, output, and prices were obtained in this study. Data for sun, wind, and rain were collected from the Shuangyang District Meteorological Bureau of Changchun City. Data of IS for purchased resources (seeds, fertilizer, electricity, diesel, coal, vaccine, labor, and so on) and output of maize production system and pig rearing system were collected from Yunfeng Cooperative manager and workers through face-to-face questionnaire survey, and data of SS were gained from local farmers in the year 2019. Prices information of grain and pork was gathered from Yunfeng Cooperative and the sales markets. The survey was conducted by the authors in 2019 and the entire survey period lasted 20 days. The raw data for inputs and outputs are shown in .
Economic analysis
The economic benefit is a key factor in whether to adopt this kind of breeding behavior for farmers. This study used two factors (profit of unit ($/UF) and ratio of income to cost) to estimate the economic benefit between the separated and integrated maize-pig systems. The higher these two indicators are, the better the economic benefits of the system are. The yield of maize and pork is an indicator to compare whether the integrated system has advantages in ensuring food safety. The yield of maize and pork, and prices of each item came from the investigation of local farmers and companies, which were in line with local actual conditions. The exchange rate between RMB and USD used in this study was the value of 2019.
Life cycle assessment
A life-cycle perspective was used to provide an objective framework for estimating and evaluating the potential environmental impacts of SS and IS. The MS boundary included the material production subsystem and farming subsystem. The PS was divided into four parts (concentrated feed, feed processing, medical breeding, feeding management), which has experienced gilts, non-pregnant, pregnant, farrowing, nursery, grow-finisher, and market from the cradle to the grave (). Data on emission parameters deriving from seeds, fertilizer, pesticide, diesel, and electricity were from the study of Liang (Citation2009). Those on the production of manure and the emissions of total nitrogen (TN), total phosphorus (TP), Cu, and Zn from manure were from CAAS (Chinese Academy of Agricultural Sciences) and MEP (Ministry of Environmental Protection) of China. Transport parameters of diesel consumption, electricity consumption, and labor came from Ecoinvent 2.2. Global warming potential (GWP), acidification potential (AP), eutrophication potential (EP), freshwater aquatic ecotoxicity (AT), human toxicity (HT), and soil ecotoxicity (ST) were included in this study according to the potential environmental impact of agricultural system emissions. This study used global per-person environmental factors to normalize characteristic results. shows global benchmarks and weight factors according to (Liang Citation2009). The calculation formula for potential environmental impact was formula (1).
Figure 3. Boundaries of systems.

Table 2. Normalization and weight factors of potential environmental impacts.
where WEi is the ith weighted evaluation result, EPi is the ith potential environmental impacts, Si is the global benchmark of ith potential environmental impacts, Wi is the weight factor of ith potential environmental impacts.
Emergy evaluation
Emergy accounting
is the emergy flow diagrams of SS and IS, which were based on the standard emergy theory put forward by Odum (Citation1996). Emergy accounting calculated the energy, materials, and services inputs with the same unit (solar equivalent joule (sej)) used in natural resources, economic resources, and social resources together. Three types of emergy resources were contained in the agricultural system in this study, which were (1) renewable resources, such as sun, wind, and rain; (2) nonrenewable resources, such as groundwater; (3) purchased resources, such as seeds, fertilizer, diesel, electricity, construction investment, labor services, and so on. Different energy inputs were converted into emergy by multiplying a suitable unit emery value (UEV) (Brown et al. Citation2016a, Citation2016b; Cristiano, Ulgiati, and Gonella Citation2021; Lan, Qin, and Lu Citation2002), it could be obtained by formula (2), and the global emergy baseline used in this paper was 1.20 × 1025 sej/yr (Brown et al. Citation2016a).
where, Emi is the emergy of ith type flow of material or energy; Qi is the quantity of item i; UEVi is the unit emergy value of item i, which was referenced to previous literature.
Emergy indices
Emergy indices in this study included Unit Energy Value (UEV), Resource Use Efficiency (RUE), Emergy Yield Ratio (EYR), Environmental Loading Ratio (ELR), and Emergy Sustainability Index (ESI). UEV, EYR, and RUE were system efficiency indexes, which evaluated the transformation efficiency of total emergy inputs and nonrenewable resources. UEV was the ratio of the total energy value of the system to the total output energy. RUE was the nonrenewable resource and potential environmental service to output energy (Wang et al. Citation2015). EYR was an economic indicator to observe own system development ability by promoting external resources utilization. ELR was the ratio of nonrenewable resources to renewable resources, which represented the environmental pressure of the system. ESI was the ratio of EYR to ELR, measuring the sustainability of the system (Odum Citation1996; Wang et al. Citation2015). All items are shown in .
Table 3. Expressions and description of the emergy indexes.
Results
Economic analysis
shows economic indices of SS and IS, and both subsystems including maize production of the separated system (MS), pig rearing of the separated system (PS), maize production of the integrated system (IMS), and pig rearing of the integrated system (IPS). From a yield perspective, the yield of IMS (2.28E+04 kg/UF) is much higher than that of MS (1.58E+04 kg/UF) for 44.30%, and the yield of IPS (2.25E+04 kg/UF) is higher than that of PS (1.91E+04 kg/UF) for 17.83%. However, the yield of IS (2.25E+04 kg/UF) is lower than that of SS (3.49E+04 kg/UF) because all corn have been recycled as forage to feed pigs in the IS. Prices of maize and pork of IS are higher than SS due to its high quality under the ecological planting and breeding processes, causing the profit of IMS, IPS is higher than that of MS and PS by 124.71% and 63.71%, respectively. IS has a lower cost than SS, as well as with higher profit than that of SS by 82.88%. Moreover, the ratio of income to cost of IMS, IPS, and IS are 2.51, 3.97, and 5.12, respectively, which are higher than MS (1.97), PS (2.89), and SS (2.80). These results indicate that the economic benefits of the maize-pig system of the Yunfeng Cooperative are better than those of separated planting and breeding systems.
Table 4. Economic indices of separated systems and integrated systems.
Environmental impacts
Potential environmental impacts
This study uses the idea of LCA to evaluate the potential environmental impact of each system from the cradle to the grave. The results of characterization, normalization, and weighted steps for 150 pigs of the pig rearing system and 2.14 ha of the maize production system are shown in . From the characterization results, the total potential environmental impacts of IS are lower than that of SS by 80.65%, with the range from 10.28% to 100% reduction for each item (GWP, AP, EU, HT, AT, and ST). The significance of the normalization results is to compare the relative size of different types of potential environmental impacts under the same criteria. The larger the normalization results, the greater the environmental impact. Among all the categories of the two systems, AT is the largest environmental impaction of SS, and GWP is the largest environmental impaction of IS. Weighted evaluation results reflect the total potential environmental impacts of SS and IS. shows SS causes thousands of times more environmental impaction than that of IS. Overall, the integrated maize-pig system performs much better than the separated system in environmental pollution.
Table 5. Potential environmental impacts of separated and integrated maize-pig systems.
Component for potential environmental impacts
The potential environmental impacts of the system are greatly affected by the input–output structure. displays the composition of both subsystems and maize-pig systems. The sources of potential environmental impacts of the separated subsystems (MS, PS, SS) and integrated subsystems (IMS, IPS, IS) are quite different. In MS, electricity use creates mostly of GWP and AP, pesticide use is the dominant cause of EU, and seeds input is the most important source of HT, AT, and ST. In IMS, electricity use is the main source of GWP, AP, and EU, seeds inputs accounted for all above 90% in HT, AT, and ST. The most production of AP, EU, HT, AT, and ST in PS is influenced by manure for all above 95%, and the electricity use of GWP accounted for 41.50%. However, there is no manure in IPS so that the electricity use is the dominant factor in the production of GWP, AP, and EU. Concentrated feed input produced HT, AT, and ST in IPS. Electricity use causes 73.35% GWP in SS, and other impacts are produced mostly by manure. In IS, electricity use is the governing source of GWP, AP, and EU, and seeds input caused HT, AT, and ST. Overall, waste management greatly mitigates environmental pollution and reducing electricity use for planting and breeding is the key factor to alleviate potential environmental impacts.
Emergy evaluation
Emergy indices analysis
Emergy indices analysis is shown in . Unit emergy value (UEV) represents the emergy efficiency of the system. The smaller the UEV, the less emergy required per unit output, and the higher the emergy efficiency of the system. The UEV of the IMS, IPS, and IS is 1.05E+04 sej/j, 2.05E+05 sej/j, and 2.14E+05 sej/j, which is 37.78%, 46.96%, and 14.44% of that of MS, PS, and SS, respectively. The results indicate that subsystems of the integrated system for planting and breeding contain higher productivity efficiency than separated systems.
Table 6. Results of emergy indices of separated and integrated systems.
The emergy yield ratio (EYR) measures the ability to obtain local resources through external resources, which shows the higher EYR, the better the socio-economic efficiency of the system. In this study, the EYR values of MS, IMS, PS, IPS, SS, and IS are 1.14, 1.25, 1.00, 1.39, 1.01, and 1.01, respectively. This shows that both maize production and pig rearing systems of the integrated system have better performance than the separated systems. That is to say, the maize-pig system of Yunfeng Cooperative can make better use of local resources with the perspective of input emergy. SS contains the same EYR compared to IS, which indicates that there is no difference in input efficiency between the two.
Nevertheless, UEV and EYR only represent the overall input–output efficiency of the system, but the resulting high efficiency may also be caused by the large proportion of nonrenewable resources. Therefore, the lower the RUE, the better the system performance. The RUE values of IMS, IPS, and IS are 4.32E+03 sej/j, 1.83E+05 sej/j, and 1.87E+05 sej/j, which is 82.06%, 56.41%, 87.28% lower than MS, PS, and SS, respectively. Internal feedback emergy (organic fertilizer in MS) and local economic resources (maize and maize straw in PS) increasing of the integrated system significantly improved emergy efficiency. Considering the three indicators of UEV, EYR, and RUE, IS has higher emergy transform efficiency.
The environmental loading ratio (ELR) reflects the pressure on the environment caused by the environmental pollution discharged by the system during the production process. The value of ELR is proportional to the pressure of the system on the environment. ELR values of MS, IMS, PS, IPS, SS and IS are 5.84, 0.69, 18.96, 8.32, 17.27, and 6.89, respectively. These results suggest that the planting and breeding system do have a certain impact on the environment. However, IMS, IPS, and IS decrease environmental pressure by 88.15%, 56.13%, and 60.08%. Nonrenewable resources of the integrated system (such as synthetic fertilizer, concentrated feed, disinfectants, and labor) with 33.01% reduction than that of separated system decrease ELR. The integrated maize-pig system helps reduce the environmental impact of agricultural production systems.
Emergy sustainability index (ESI) is an indicator of system sustainability evaluation, which combines system input and output efficiency and pressure on the environment. A sustainable system would provide sufficient resources, increase output by increasing feedback resources, and at the same time have less environmental pressure. The higher the ESI, the higher the sustainability of the system. ESI values of MS, IMS, PS, IPS, SS, and IS are 0.19, 1.81, 0.05, 0.17, 0.06, and 0.15, respectively. The integrated maize-pig system greatly improves system sustainability, with 828.59% for the planting system, 193.16% for the breeding system, and 199.52% for the integrated system. Nonrenewable resources decreasing and renewable resources increasing primarily lead to the improvement of ESI. The results indicate that the maize-pig system driven by indigenous microbes implemented by Yunfeng Cooperative has good prospects for sustainable development.
Emergy input structure
Different emergy input structures lead to different UEV, RUE, EYR, ELR, and ESI. expresses the emergy input structure of the systems. Economic feedback emergy of IS is 33.40% lower than that of SS. Fodder and maize straw emergy inputs are 9.98E+16 sej/j and 6.13E+16 sej/j in SS and IS, respectively, with 75.30% proportion in SS and 48.64% proportion in IS (). While comparing the two systems, IS decreases 38.68%, 56.98%, 100%, 60.02%, 60.80%, 100%, 46.11%, and 100% in fodder, fertilizer, pesticides, diesel, labor, coal, vaccine, and disinfectants, respectively, than SS (). Electricity and construction investment inputs of IS are 35.51% and 218.27% higher than that of SS. There is no difference in seeds and equipment investment emergy input of the two systems.
Considering planting production system, fertilizer and labor input accounted most, with 74.22% of fertilizer and 24.75% of labor in MS, and 54.28% of pig manure and 45.37% of labor in IMS. For the comparison between the two systems, pig manure input is 3.37E+14 sej/j of IMS, which is 60.10% lower than the fertilizer input of MS. Pesticides input of MS with 4.77E+13 sej/j accounts for 0.84% of total emergy input, and that of no input of IMS. There is no difference in the input of seeds, electricity, diesel, and labor in the two systems, which is 1.62E+10 sej/j, 3.54E+09 sej/j, 1.08E+13 sej/j, and 1.41E+15 sej/j, respectively. On the whole, economic feedback emergy of IMS with 45.44% reduction compares to MS, showing in fertilizer and pesticides input.
Economic feedback emergy of IPS is 26.86% lower than PS. Vitamin premix and disinfectants input in PS account for a relatively large proportion for 57.85% and 9.51%, respectively. Vitamin premix, construction investment, and maize straw input account for a large proportion for 29.19%, 19.69%, and 18.34% in IPS. Moreover, disinfectants make up 9.51% of PS. However, there is no such investment in the IPS because pigs eat microbial metabolites, such as organic acids, bacterial proteins, antibacterial peptides, and so on, which can aid digestion and improve pig immunity. Pigs of Yunfeng cooperative rely on back feeding and epidemic prevention to reduce the sickness rate. As known to us from , fodder input of PS accounts for 78.68%, and that of 54.48% of IPS. Fodder emergy input is 9.98E+16 sej/j in PS, which increases 97.47% than that of IPS. This result occurs because the pigs directly eat maize straw sprayed with microbial inoculants in IPS, replacing part of the concentrated feed, and the emergy input of maize straw is 1.83E+16 sej/j. In addition to this, IPS has the advantages of no need to clean up manure, and less feeding times, thus reducing the input of labor by 77.33%. In IPS, the heat produced by the fermentation bed itself reduces the input of coal for heating but increases the input of electricity in heating equipment (). In general, IPS has lower emergy input than PS in fodder, diesel, labor, coal, vaccine, and disinfectants.
Discussion
Economic and social contribution advantages of indigenous microbes-driven circular agriculture
Applying organic manures can increase soil organic carbon (SOC) and grain yield across the consist of one continuous maize planting (Zhang, Xu, and Zhang Citation2008). Crop-livestock integrated system can improve farm productivity and reduce risk (Bell, Moore, and Kirkegaard Citation2014; Lemaire et al. Citation2014). Szymczak et al. proved that crop-livestock systems were more adaptable and economically valuable than specialized soybean system, as well as improved nutrient cycling and resource-use efficiency (Ma et al. Citation2022). The results of this study are consistent with previous research results. Maize yield in this study was 10,669.6 kg/ha with pig manure return, increasing 44.29% compared with no recycling maize production system. In addition, the output of fattening pigs was higher than that of ordinary pigs. The cost of the integrated system was higher than that of the traditional system, reflecting in diesel, electricity, and labor in IMS was higher than that of MS by 43.70%, 43.93%, and 43.93%, as well as in soybean meal, electricity, and construction investment of IPS was higher than that of PS by 47.68%, 35.51%, and 218.27%. Construction investment of IPS accounted for mostly by 40.47%, containing pigsty build, fermentation build, and other input. Increasing the life of the pig house can significantly reduce the cost of the integrated system. However, the economic profit of IS was higher due to its high product quality and high price, which was the primary driving factor for farmers when choosing production behaviors.
Apart from this, the construction of the cooperative production and operation mode has also brought some social benefits, focusing on increasing farmers’ income and employment opportunities and improving farmers’ professional knowledge in the previous studies (Bell, Moore, and Kirkegaard Citation2014; Garrett et al. Citation2017; Gil, Garrett, and Berger Citation2016). Yunfeng Cooperative used advanced scientific breeding technology to drive farmers to get rich together through technical training, medicine with indigenous microbial support, and basing a rural revitalization model of “company + cooperative + base + farmer.” Under the guidance of the rural revitalization strategy, various parts of China have innovated a variety of new agricultural and rural development models, such as “organic agriculture + beautiful rural construction + ecologically happy tourism + decent work to support aspirations” model of Chehe village (Xu et al. Citation2021), leading enterprises driven of Tengtou village (Zang et al. Citation2020). This development model for circular agriculture relying on indigenous microbes of Yunfeng Cooperative promoted employment and increased farmers’ income, with huge social benefits.
Sustainability of agricultural circular systems
To meet the demands for food and meat consumption by increasing population in China, agriculture gradually depends on energy, resources, and other input from outside support. The characteristics of intensive production and obvious separation have become more and more remarkable in crop and livestock production. A large amount of agricultural waste has an impact on the environment and human health so that the sustainability of agricultural system is widely concerned. A series of studies on cropping systems and breeding scales were heatedly discussed (Lyu et al. Citation2020; Wang et al. Citation2014; Zhai et al. Citation2018; Zhang et al. Citation2016). Circular agriculture in China has been encouraged by the government and benefits, such as crop–crop system, crop-livestock system, and crop-livestock-biogas system. Zhang, Yang, and Chen (Citation2007) analyzed sustainability of the integrated cropping-grazing system in Inner Mongolia was higher than that of the cropping system but lower than that of the grazing system. An eco-agricultural model of integrated production systems for cattle, biogas, and greenhouse vegetables was constructed in Northwest China, which improved resource utilization and mitigated environmental emission with a low input–output ratio (Wu et al. Citation2013). Wu et al. (Citation2015) compared the integrated system of multistoried cropping, orchard planting, and biogas production by waste fermentation with the conventional system, which indicated that the integrated system improved yield, resources efficiency, environment, and sustainability, despite high environmental load and low sustainability. Substituting animal manure for synthetic fertilizers decreases CH4 and N2O emissions in the integrated crop-livestock system (Bell, Moore, and Kirkegaard Citation2014; Du et al. Citation2022; Salton et al. Citation2014). In order to measure sustainability between separated and integrated maize-pig systems, this study combined efficiency indicators (UEV, EYR, and RUE), environmental impact indicators (ELR, potential environmental impacts), and emergy sustainability index (ESI) to express. The results of this study showed the performance of economic, environmental pollution and sustainability for the integrated maize-pig system appeared advantages, compared with the separated system, which was the same tendency of previous studies.
IS performed a lot of advantages in the emergy evaluation and potential environmental impacts compared with SS. As an upstream method, emergy accounting reflects the sustainability of the system through the direct and indirect consumption structure. System efficiency is related with the emergy input and output, as well as the use of renewable resources. From , we can see that the ratio of renewable resource use of IS (8.94%) is higher than that of SS (4.52%), mainly due to the replacement of chemical fertilizers with organic fertilizers and the replacement of concentrated feeds with maize straw. Comparing the results of LCA between SS and IS, manure and concentrated feed input are also important factors to produce potential environmental pollution. Manure reuse and straw feed are effective means of promoting the sustainability of the system. Moreover, electricity use is also the key factor in both SS and IS, and how to reduce the use of electricity is the key point of energy conservation and emission reduction in the future.
Research limitations of this study
Compared with non-recycling systems, the systems that include reusing and recycling of agricultural waste driven by indigenous microbes, effectively reduced environmental impacts, improved economic profits and sustainability. However, the limitations of fermentation technology hindered the widespread application of technology. For instance, standard pigsty and fermentation bed needed large cost input, dynamic changes of bacterial community during fermentation process driven by indigenous microbes (Chen et al. Citation2017b), and the temperature in the pigsty was too high in summer, affecting the growth and development of pigs (Liang, Deng, and Qin Citation2021). Additionally, the long-term application of livestock manure was a predominant source for heavy metal accumulation in agricultural soils, increasing GHGs emissions (Shakoor et al. Citation2021; Zhou et al. Citation2017). In previous studies, Zn, Cu, Cs, and As occurred as fairly serious pollution in the pig manure of China (Liu et al. Citation2020). Applying animal manure usually enhanced carbon content and then converted into CO2, and can be considered as the best predictor of CH4 emission from cropland, as well as can lead to N2O production in soils through the biotic and abiotic processes (Gattinger et al. Citation2012; Shakoor et al. Citation2016, Citation2020). There has been no report related to reducing this phenomenon of fermentation technology driven by indigenous microbes (Shi et al. Citation2018). Thirdly, different scales controlled economic and environmental consequences for the planting and breeding systems. Compared to fragmented farmland, concentrated farmland performed advantages in economic and environmental pollution (Yang et al. Citation2019). Currently, China has to expand the scale of livestock production to some extent despite the larger production scale causing better economic benefits and worse environmental consequences (Wang et al. Citation2016). Although much work remains on indigenous microbes-driven integrated maize-pig system, it performs considerable advantages in reducing waste emissions and improving sustainability.
Conclusion
The long-term single intensive planting model and the separation of crop production and livestock rearing have led to the serious accumulation of agricultural waste, and there is an urgent need to innovate circular agricultural models. This study compared integrated maize-pig system driven by indigenous microbes in Northeast China with separated maize production system and pig rearing system, employing a combination of economic analysis, emergy accounting, and life cycle assessment to estimate system sustainability. The results indicate that indigenous microbes-driven circular system showed higher emergy efficiency and sustainability, and reduced potential environmental impact compared with systems that do not recycle. IS increased economic profit by 82.88% compared to SS, although with higher costs. Meanwhile, IS reduced environmental impacts by 80.65% than that of SS due to the reduction of fertilizer, diesel, coal, vaccine, and disinfectants driven by microbes. Organic fertilizer and maize straw increasing of IS significantly improved emergy efficiency by 87.28% than that of SS. Nonrenewable resources reduction and renewable resources growth reduced environmental pressure, resulting in higher sustainability. In addition, subsystems of IS (IMS, IPS) performed better than the subsystems of SS (MS, PS) in economic, environmental impacts and sustainability. Upgrading the fermentation bed technology and the scale of the combination of planting and breeding are the focus points in future research. Although much work remains for the development of recycling agriculture, the integrated maize-pig system driven by indigenous microbes is a credible circular model to improve agricultural sustainability.
Disclosure statement
No potential conflict of interest was reported by the author(s).
Additional information
Funding
References
- Adegbeye, M. J., P. Ravi Kanth Reddy, A. I. Obaisi, M. M. M. Y. Elghandour, K. J. Oyebamiji, A. Z. M. Salem, O. T. Morakinyo-Fasipe, M. Cipriano-Salazar, and L. M. Camacho-Díaz. 2020. “Sustainable Agriculture Options for Production, Greenhouse Gases and Pollution Alleviation, and Nutrient Recycling in Emerging and Transitional Nations - an Overview.” Journal of Cleaner Production 242: 1. doi:10.1016/j.jclepro.2019.118319.
- AgroCycle. 2017. “Agrocycle Protocol Rules 2017.” http://www.agrocycle.eu/
- Angouria-Tsorochidou, E., D. A. Teigiserova, and M. Thomsen. 2021. “Limits to Circular Bioeconomy in the Transition Towards Decentralized Biowaste Management Systems.” Resources, Conservation and Recycling 164: 105207. doi:10.1016/j.resconrec.2020.105207.
- Bai, Z., G. Schmidt-Traub, J. Xu, L. Liu, X. Jin, and L. Ma. 2020. “A Food System Revolution for China in the post-pandemic World.” Resources, Environment and Sustainability 2: 100013. doi:10.1016/j.resenv.2020.100013.
- Bell, L. W., A. D. Moore, and J. A. Kirkegaard. 2014. “Evolution in crop–livestock Integration Systems that Improve Farm Productivity and Environmental Performance in Australia.” European Journal of Agronomy 57: 10–15. doi:10.1016/j.eja.2013.04.007.
- Brown, M. T., D. E. Campbell, C. De Vilbiss, and S. Ulgiati. 2016a. “The Geobiosphere Emergy Baseline: A Synthesis.” Ecological Modelling 339: 92–95. doi:10.1016/j.ecolmodel.2016.03.018.
- Brown, M. T., D. E. Campbell, S. Ulgiati, and P. P. Franzese. 2016b. “The Geobiosphere Emergy Baseline: A Synthesis.” Ecological Modelling 339: 89–91. doi:10.1016/j.ecolmodel.2016.09.009.
- Chen, M., J. Chen, and F. Sun. 2010. “Estimating Nutrient Releases from Agriculture in China: An Extended Substance Flow Analysis Framework and a Modeling Tool.” Science of the Total Environment 408 (21): 5123–5136. doi:10.1016/j.scitotenv.2010.07.030.
- Chen, L., R.-G. Cong, B. Shu, and Z.-F. Mi. 2017a. “A Sustainable Biogas Model in China: The Case Study of Beijing Deqingyuan Biogas Project.” Renewable and Sustainable Energy Reviews 78: 773–779. doi:10.1016/j.rser.2017.05.027.
- Chen, Q., B. Liu, J. Wang, J. Che, G. Liu, and X. Guan. 2017b. “Diversity and Dynamics of the Bacterial Community Involved in Pig Manure Biodegradation in a Microbial Fermentation Bed System.” Annals of Microbiology 67 (7): 491–500. doi:10.1007/s13213-017-1278-y.
- Cristiano, S., S. Ulgiati, and F. Gonella. 2021. “Systemic Sustainability and Resilience Assessment of Health Systems, Addressing Global Societal Priorities: Learnings from a Top Nonprofit Hospital in a Bioclimatic Building in Africa.” Renewable and Sustainable Energy Reviews 141 141: 110765. doi:10.1016/j.rser.2021.110765.
- Du, H., J. Cui, Y. Xu, Y. Zhao, L. Chen, Z. Li, P. Sui, W. Gao, and Y. Chen. 2022. “Nitrogen Footprint of a Recycling System Integrated with Cropland and Livestock in the North China Plain.” Plants 11 (7): 842. doi:10.3390/plants11070842.
- Fan, W., X. Dong, H. Wei, B. Weng, L. Liang, Z. Xu, X. Wang, et al. 2018a. “Is It True that the Longer the Extended Industrial Chain, the Better the Circular Agriculture? A Case Study of Circular Agriculture Industry Company in Fuqing, Fujian.” Journal of Cleaner Production 189: 718–728. doi:10.1016/j.jclepro.2018.04.119.
- Fan, W., P. Zhang, Z. Xu, H. Wei, N. Lu, X. Wang, B. Weng, Z. Chen, F. Wu, and X. Dong. 2018b. “Life Cycle Environmental Impact Assessment of Circular Agriculture: A Case Study in Fuqing, China.” Sustainability 10 (6): 1810. doi:10.3390/su10061810.
- FAO. 2015. “Transforming Our World: The 2030 Agenda for Sustainable Development, Food and Agriculture Organization of the United Nations Rome.” https://www.un.org/ga/search/view_doc.asp?symbol=A/RES/70/1&Lang=E
- Garrett, R. D., M. T. Niles, J. D. B. Gil, A. Gaudin, R. Chaplin-Kramer, A. Assmann, T. S. Assmann, et al. 2017. “Social and Ecological Analysis of Commercial Integrated Crop Livestock Systems: Current Knowledge and Remaining Uncertainty.” Agricultural Systems 155: 136–146. doi:10.1016/j.agsy.2017.05.003.
- Gattinger, A., A. Muller, M. Haeni, C. Skinner, A. Fliessbach, N. Buchmann, P. Mader, et al. 2012. “Enhanced Top Soil Carbon Stocks under Organic Farming.” Proceedings of the National Academy of Sciences of the United States of America 109 (44): 18226–18231. doi:10.1073/pnas.1209429109.
- Gil, J. D. B., R. Garrett, and T. Berger. 2016. “Determinants of crop-livestock Integration in Brazil: Evidence from the Household and Regional Levels.” Land Use Policy 59: 557–568. doi:10.1016/j.landusepol.2016.09.022.
- Lan, S. F., P. Qin, and H. F. Lu. 2002. Emergy Analysis of Eco-Economic System. Beijing, China: Chemical Industry Press.
- Lemaire, G., A. Franzluebbers, P. C. D. F. Carvalho, and B. Dedieu. 2014. “Integrated crop–livestock Systems: Strategies to Achieve Synergy between Agricultural Production and Environmental Quality.” Agriculture, Ecosystems & Environment 190: 4–8. doi:10.1016/j.agee.2013.08.009.
- Li, Y., Y. Chen, and J. Wu. 2019. “Enhancement of Methane Production in Anaerobic Digestion Process: A Review.” Applied Energy 240: 120–137. doi:10.1016/j.apenergy.2019.01.243.
- Li, H., Y. Yao, X. Zhang, H. Zhu, and X. Wei. 2021. “Changes in Soil Physical and Hydraulic Properties following the Conversion of Forest to Cropland in the Black Soil Region of Northeast China.” Catena 198. doi:10.1016/j.catena.2020.104986.
- Liang, L. 2009. “Environmental Impact Assessment of Circular Agriculture Based on Life Cycle Assessment: Methods and Case Study.” Doctoral thesis, Beijing, China: China Agricultural University.
- Liang, W., F. Deng, and S. Qin. 2021. “Analysis of Advantages and Disadvantages of Fermentation Bed in Pig Production.” Animals Breeding and Feed 20 (3): 27–28. doi:10.13300/j.cnki.cn42-1648/s.2021.03.009.
- Liu, W. R., D. Zeng, L. She, W. X. Su, D. C. He, G. Y. Wu, X. R. Ma, S. Jiang, C. H. Jiang, and G. G. Ying. 2020. “Comparisons of Pollution Characteristics, Emission Situations, and Mass Loads for Heavy Metals in the Manures of Different Livestock and Poultry in China.” Science of the Total Environment 734: 139023. doi:10.1016/j.scitotenv.2020.139023.
- Lyu, Y., J. Li, R. Hou, H. Zhu, W. Zhu, S. Hang, and Z. Ouyang. 2020. “Goats or Pigs? Sustainable Approach of Different Raising Systems Fed by Maize Silage.” Journal of Cleaner Production 254: 120151. doi:10.1016/j.jclepro.2020.120151.
- Ma, Y., Y. Hou, P. Dong, G. L. Velthof, W. Long, L. Ma, W. Ma, R. Jiang, and O. Oenema. 2022. “Cooperation between Specialized Livestock and Crop Farms Can Reduce Environmental Footprints and Increase Net Profits in Livestock Production.” Journal of Environmental Management 302 (Pt A): 113960. doi:10.1016/j.jenvman.2021.113960.
- Malamis, D., A. Bourka, E. Stamatopoulou, K. Moustakas, O. Skiadi, and M. Loizidou. 2017. “Study and Assessment of Segregated Biowaste Composting: The Case Study of Attica Municipalities.” Journal of Environmental Management 203 (Pt 2): 664–669. doi:10.1016/j.jenvman.2016.09.070.
- Ministry of Agriculture. 2018. “Nature and Food Quality of Netherlands.” Vision Ministry of Agriculture, Nature and Food Quality. https://www.government.nl/ministries/ministry-of-agriculture-nature-and-food-quality/vision-anf
- Morrison, R. S., L. J. Johnston, and A. M. Hilbrands. 2007. “The Behaviour, Welfare, Growth Performance and Meat Quality of Pigs Housed in a deep-litter, Large Group Housing System Compared to a Conventional Confinement System.” Applied Animal Behaviour Science 103 (1–2): 12–24. doi:10.1016/j.applanim.2006.04.002.
- Muller, A., C. Schader, N. El-Hage Scialabba, J. Bruggemann, A. Isensee, K. H. Erb, P. Smith, et al. 2017. “Strategies for Feeding the World More Sustainably with Organic Agriculture.” Nature Communications 8 (1): 1290. doi:10.1038/s41467-017-01410-w.
- Nielsen, P. H. 2017. “Microbial Biotechnology and Circular Economy in Wastewater Treatment.” Microbial Biotechnology 10 (5): 1102–1105. doi:10.1111/1751-7915.12821.
- Odum, H. T. 1996. Environmental Accounting: Emergy and Environmental Decision-Making. Vol. 370. Hoboken, NJ, USA: John Wiley Sons.
- Peng, X., I. A. Nges, and J. Liu. 2016. “Improving Methane Production from Wheat Straw by Digestate Liquor Recirculation in Continuous Stirred Tank Processes.” Renewable Energy 85: 12–18. doi:10.1016/j.renene.2015.06.023.
- Ravindran, B., N. Karmegam, A. Yuvaraj, R. Thangaraj, S. W. Chang, Z. Zhang, and M. Kumar Awasthi. 2021. “Cleaner Production of Agriculturally Valuable Benignant Materials from Industry Generated bio-wastes: A Review.” Bioresource Technology 320 (Pt A): 124281. doi:10.1016/j.biortech.2020.124281.
- Salton, J. C., F. M. Mercante, M. Tomazi, J. A. Zanatta, G. Concenço, W. M. Silva, and M. Retore. 2014. “Integrated crop-livestock System in Tropical Brazil: Toward a Sustainable Production System.” Agriculture, Ecosystems & Environment 190: 70–79. doi:10.1016/j.agee.2013.09.023.
- Shakoor, A., M. Abdullah, B. Yousaf, Amina, and Y. Ma. 2016. “Atmospheric Emission of Nitric Oxide and Processes Involved in Its Biogeochemical Transformation in Terrestrial Environment.” Environmental Science and Pollution Research International. doi:10.1007/s11356-016-7823-6.
- Shakoor, A., F. Ashraf, S. Shakoor, A. Mustafa, A. Rehman, and M. M. Altaf. 2020. “Biogeochemical Transformation of Greenhouse Gas Emissions from Terrestrial to Atmospheric Environment and Potential Feedback to Climate Forcing.” Environmental Science and Pollution Research 27 (31): 38513–38536. doi:10.1007/s11356-020-10151-1.
- Shakoor, A., S. Shakoor, A. Rehman, F. Ashraf, M. Abdullah, S. M. Shahzad, T. H. Farooq, et al. 2021. “Effect of Animal Manure, Crop Type, Climate Zone, and Soil Attributes on Greenhouse Gas Emissions from Agricultural soils—A Global meta-analysis.” Journal of Cleaner Production 278: 124019. doi:10.1016/j.jclepro.2020.124019.
- Sharma, P., V. K. Gaur, S. H. Kim, and A. Pandey. 2020. “Microbial Strategies for bio-transforming Food Waste into Resources.” Bioresource Technology 299: 122580. doi:10.1016/j.biortech.2019.122580.
- Shi, T., J. Ma, X. Wu, T. Ju, X. Lin, Y. Zhang, X. Li, et al. 2018. “Inventories of Heavy Metal Inputs and Outputs to and from Agricultural Soils: A Review.” Ecotoxicology and Environmental Safety 164: 118–124. doi:10.1016/j.ecoenv.2018.08.016.
- Steffen, W., K. Richardson, J. Rockstrom, S. E. Cornell, I. Fetzer, E. M. Bennett, R. Biggs, et al. 2015. “Sustainability. Planetary Boundaries: Guiding Human Development on a Changing Planet.” Science 347 (6223): 1259855. doi:10.1126/science.1259855.
- Toop, T. A., S. Waed, T. Oldfied, M. Hull, M. E. Kirby, and M. K. Theodorou. 2017. “AgroCycle – Developing a Circular Economy in Agriculture.pdf.” Energy Procedia 123: 76–80. doi:10.1016/j.egypro.2017.07.269.
- Wang, X., Y. Chen, P. Sui, W. Gao, F. Qin, J. Zhang, and X. Wu. 2014. “Emergy Analysis of Grain Production Systems on large-scale Farms in the North China Plain Based on LCA.” Agricultural Systems 128: 66–78. doi:10.1016/j.agsy.2014.03.005.
- Wang, X., A. Dadouma, Y. Chen, P. Sui, W. Gao, and L. Jia. 2015. “Sustainability Evaluation of the large-scale Pig Farming System in North China: An Emergy Analysis Based on Life Cycle Assessment.” Journal of Cleaner Production 102: 144–164. doi:10.1016/j.jclepro.2015.04.071.
- Wang, X., X. Wu, P. Yan, W. Gao, Y. Chen, and P. Sui. 2016. “Integrated Analysis on Economic and Environmental Consequences of Livestock Husbandry on Different Scale in China.” Journal of Cleaner Production 119: 1–12. doi:10.1016/j.jclepro.2016.01.084.
- Wang, Q., Y. Zhang, S. Tian, X. Yuan, Q. Ma, M. Liu, Y. Li, and J. Liu. 2021a. “Evaluation and Optimization of a Circular Economy Model Integrating Planting and Breeding Based on the Coupling of Emergy Analysis and Life Cycle Assessment.” Environmental Science and Pollution Research International. doi:10.21203/rs.3.rs-161699/v1.
- Wang, S., X. Huang, Y. Zhang, C. Yin, and A. Richel. 2021b. “The Effect of Corn Straw Return on Corn Production in Northeast China: An Integrated Regional Evaluation with meta-analysis and System Dynamics.” Resources, Conservation and Recycling 167: 105402. doi:10.1016/j.resconrec.2021.105402.
- Wu, X., F. Wu, X. Tong, and B. Jiang. 2013. “Emergy-based Sustainability Assessment of an Integrated Production System of Cattle, Biogas, and Greenhouse Vegetables: Insight into the Comprehensive Utilization of Wastes on a large-scale Farm in Northwest China.” Ecological Engineering 61: 335–344. doi:10.1016/j.ecoleng.2013.09.060.
- Wu, X., F. Wu, X. Tong, J. Wu, L. Sun, and X. Peng. 2015. “Emergy and Greenhouse Gas Assessment of a Sustainable, Integrated Agricultural Model (SIAM) for Plant, Animal and Biogas Production: Analysis of the Ecological Recycle of Wastes.” Resources, Conservation and Recycling 96: 40–50. doi:10.1016/j.resconrec.2015.01.010.
- Xian, Y., Y. Chen, C. Chen, R. He, X. Chen, Y. Chen, and X. Wang. 2020. “Does Extending Recycling Chain of Using Rice Straw Contribute to Improving Yield and Reducing GHGs Emissions in Paddy Field? An Integrated Analysis Based on Field Research and System Assessment.” Journal of Cleaner Production 264: 121508. doi:10.1016/j.jclepro.2020.121508.
- Xiong, J., X. Chen, K. Tan, Y. Xian, and X. Wang. 2020. “On Status and Trends of Circular Agriculture in Bibliometrics Method.” Journal of Southwest China Normal University (Natural Science Edition) 45 (8): 58–66. doi:10.13718/j.cnki.xsxb.2020.08.010.
- Xu, Y., Y. Zhao, P. Sui, W. Gao, Z. Li, and Y. Chen. 2021. “Emergy-Based Evaluation on the Systemic Sustainability of Rural Ecosystem under China Poverty Alleviation and Rural Revitalization: A Case of the Village in North China.” Energies 14 (13). doi:10.1016/j.jclepro.2018.05.066.
- Yang, X., L. Cheng, C. Yin, P. Lebailly, and H. Azadi. 2018. “Urban Residents’ Willingness to Pay for Corn Straw Burning Ban in Henan, China: Application of Payment Card.” Journal of Cleaner Production 193: 471–478. doi:10.1016/j.jclepro.2018.05.066.
- Yang, X., P. Sui, X. Zhang, H. Dai, P. Yan, C. Li, X. Wang, and Y. Chen. 2019. “Environmental and Economic Consequences Analysis of Cropping Systems from Fragmented to Concentrated Farmland in the North China Plain Based on a Joint Use of Life Cycle Assessment, Emergy and Economic Analysis.” Journal of Environmental Management 251: 109588. doi:10.1016/j.jenvman.2019.109588.
- Zang, Y., Y. Liu, Y. Yang, M. Woods, and F. Fois. 2020. “Rural Decline or Restructuring? Implications for Sustainability Transitions in Rural China.” Land Use Policy 94: 104531. doi:10.1016/j.landusepol.2020.104531.
- Zhai, X., H. Zhao, L. Guo, D. M. Finch, D. Huang, K. Liu, S. Tang, et al. 2018. “The Emergy of Metabolism in the Same Ecosystem (Maize) under Different Environmental Conditions.” Journal of Cleaner Production 191: 233–239. doi:10.1016/j.jclepro.2018.04.208.
- Zhang, L. X., Z. F. Yang, and G. Q. Chen. 2007. “Emergy Analysis of cropping–grazing System in Inner Mongolia Autonomous Region, China.” Energy Policy 35 (7): 3843–3855. doi:10.1016/j.enpol.2007.01.022.
- Zhang, H., M. Xu, and F. Zhang. 2008. “Long-term Effects of Manure Application on Grain Yield under Different Cropping Systems and Ecological Conditions in China.” The Journal of Agricultural Science 147 (1): 31–42. doi:10.1017/S0021859608008265.
- Zhang, P., Y. Yang, Y. Tian, X. Yang, Y. Zhang, Y. Zheng, and L. Wang. 2009. “Bioenergy Industries Development in China: Dilemma and Solution.” Renewable and Sustainable Energy Reviews 13 (9): 2571–2579. doi:10.1016/j.rser.2009.06.016.
- Zhang, X., R. Zhang, J. Wu, Y. Zhang, L. Lin, S. Deng, L. Li, et al. 2016. “An Emergy Evaluation of the Sustainability of Chinese Crop Production System during 2000–2010.” Ecological Indicators 60: 622–633. doi:10.1016/j.ecolind.2015.08.004.
- Zheng, Z., Y. Cai, Y. Zhao, X. Meng, Y. Zhang, C. Lu, Y. Hu, Z. Cui, and X. Wang. 2020. “Achieve Clean and Efficient Biomethane Production by Matching between Digestate Recirculation and straw-to-manure Feeding Ratios.” Journal of Cleaner Production 263. doi:10.1016/j.jclepro.2020.121414.
- Zhou, M., B. Zhu, S. Wang, X. Zhu, H. Vereecken, and N. Bruggemann. 2017. “Stimulation of N2O Emission by Manure Application to Agricultural Soils May Largely Offset Carbon Benefits: A Global meta-analysis.” Global Change Biology 23 (10): 4068–4083. doi:10.1111/gcb.13648.
Appendix
Appendix A. Nomenclatures of this study for abbreviation and full name.