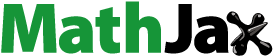
ABSTRACT
Human activities interfere with natural Phosphorus (P) cycles by introducing increased levels of P emissions to air, land, and water. A supply-side analysis of P supply chains and associated P emissions can provide insights into underlying economic activities and transitions responsible for human-induced P emissions. Taking the mainland China as the case, this study constructs time-series physical input-output tables to describe P supply chains during 1949–2012. Subsequently, it identifies critical products and influencing factors of P supply chains enabling P emissions to the environment (including air, land, and water) from the supply perspective. The results show that phosphate rock, an important initial supplier of P from natural environment to China’s P supply chain, was responsible for 86% of P emissions in 2012. Moreover, food crops and livestock are important initial suppliers of P from soil to China’s P supply chain, through cultivation and pasturing, respectively. From 1949 to 2012, the change in primary input level was the largest driver of P emission increments, followed by changes in population, emission intensity, and primary input structure. On the contrary, changes in production structure reduced P emissions. These findings could support supply-side policy decisions on P emission control.
Introduction
Phosphorus (P) plays an important role in human survival and biogeochemical cycles of other elements (Hunter Citation2012; Smil Citation2000). Rapid socioeconomic development and population growth have significantly influenced the P cycle. Economic production and consumption activities produce a large number of P emissions to the environment (including air, land, and water), resulting in water eutrophication and P resource depletion (Chen and Graedel Citation2016; Carpenter et al. Citation1998; Cordell, Drangert, and White Citation2009). The global P cycle has become more open due to human intervention, which cannot be repaired in the short term and has a significant impact on ecosystem functions (Yuan et al. Citation2018). This poses a twofold challenge to humanity: P scarcity in the coming years due to its increasing demand and deteriorating environmental quality due to P emissions (Chen and Graedel Citation2016; Liu et al. Citation2020, Citation2016).
China is a large producer as well as consumer of P. However, the status of the P cycle in China is not optimistic (Chen and Graedel Citation2016; Liu et al. Citation2020, Citation2016). China is a populous and agricultural country, with about 18% of the world’s population and less than half of the world’s average arable land per capita, made possible by China’s agricultural revolution and the use of mineral fertilizers (Li et al. Citation2016). Excessive use of P fertilizers will cause not only resource scarcity but also environmental problems (Huang et al. Citation2017; Bai et al. Citation2016; Wang et al. Citation2018; Hou et al. Citation2013; Smith and Siciliano Citation2015). When comparing 1950 to 2010, China’s annual loss of P from crop production grew 10-fold to 0.4 Mt, and the total loss of P to surface water bodies increased 12-fold (Huang et al. Citation2017; Bai et al. Citation2016). By 2020, 23.6% of China’s major lakes monitored for eutrophication were found to be mildly eutrophic, 4.5% were moderately eutrophic, and 0.9% were severely eutrophic (China Citation2021). It is, therefore urgent for China to control its P emissions.
Previous studies mainly focused on P flow pathways in environmental-economic systems and P emissions from each stage (Li et al. Citation2016; Wang et al. Citation2011; Li et al. Citation2010; Antikainen et al. Citation2005; Liu et al. Citation2008; Villalba et al. Citation2008; Kalmykova et al. Citation2012; Chen, Chen, and Sun Citation2008; Cooper and Carliell-Marquet Citation2013; Jeong et al. Citation2009; Senthilkumar et al. Citation2012; Suh and Yee Citation2011; Ma et al. Citation2011; Wu et al. Citation2014; Jiang et al. Citation2019). For instance, Li et al. used material flow analysis to investigate the P flow and its relationship to water pollution in Hefei, China, in 2008 (Li et al. Citation2010). Based on the mass balance method, Li et al. performed a thorough analysis of P flows, P stocks, P use efficiency, and environmental impacts in China in 2010 using a static material flow analysis model (Li et al. Citation2016). Jiang et al. used the P footprint model to conduct a quantitative analysis of the P footprint and drivers in China from 1961 to 2014, focusing on the main P-containing products consumed by humans (Jiang et al. Citation2019). Yan et al. investigated P flow pathways in the Dianchi basin from 1960 to 2017 and evaluated human’s influence on the P cycle (Yan et al. Citation2021). They identified direct P emitters and hotspots for end-of-pipe control measures. Human activities can also indirectly lead to P emissions through P supply chains. For example, inputs of P from nature would enable production activities of P supply chains and related P emissions. Thus, it is also urgent to identify primary suppliers of P supply chains that indirectly enable downstream P emissions. Moreover, temporal changes of factors in P supply chains (e.g., primary input level and production structure) would lead to changes in P emissions, but remain unknown. Investigating this point can reveal hotspots for P emission control from a temporal dynamic perspective.
This study identifies critical primary suppliers in P supply chains enabling P emissions in China, based on physical input-output tables for P flows. In this study, P emissions refer to the loss of P from socioeconomic systems to the environment including the flow to air (such as wind erosion), water bodies, and land. In addition, we quantified the contributions of socioeconomic factors to P emission changes in China during 1949–2012 from the supply perspective, using structural decomposition analysis (SDA). This study identifies key products and socioeconomic factors affecting P emissions from the supply side. It provides supply-side policy implications for sustainable P management and P emission reduction. Existing studies have explored the path and scale of P flows in the socioeconomic system, conducted quantitative studies and analyses on direct P emissions focusing on the downstream of P production, built P flow models at different spatial scales, or proposed terminal treatment and control schemes for major emission sources (Li et al. Citation2016; Wang et al. Citation2011; Li et al. Citation2010; Antikainen et al. Citation2005; Liu et al. Citation2008; Villalba et al. Citation2008; Kalmykova et al. Citation2012; Chen, Chen, and Sun Citation2008; Cooper and Carliell-Marquet Citation2013; Jeong et al. Citation2009; Senthilkumar et al. Citation2012; Suh and Yee Citation2011; Ma et al. Citation2011; Wu et al. Citation2014; Jiang et al. Citation2019; Yan et al. Citation2021). However, the key factors affecting P emission in the P cycle network were not identified from a holistic perspective. P is extracted from the natural environment and mainly used in the socioeconomic system, and the uptake of P in this process indirectly contributes to downstream P emissions. The effective sustainable management of P should not only focus on the downstream of the P supply chain, but also control and adjust the upstream of the supply chain. To fulfill the literature gap and respond to practical demand, this study conducted a supply-side accounting and analysis of socioeconomic drivers of P emissions, which aims to identify key primary suppliers and reveal how primary inputs lead to P emissions, thus providing supply-side policy suggestions on the sustainable management of P.
Methods and data
Building physical input-output tables
This study compiles physical supply and use tables (SUTs) of P in mainland China from 1949 to 2012. The P SUTs are then transformed into physical input-output tables (Mahajan et al. Citation2018) (PIOTs) based on the industry technology assumption (where it is assumed that the input structure is the same for all products produced by an industry, irrespective of its product mix). The PIOTs describe the flow of P in natural ecosystems and socioeconomic systems.
P supply chain refers to the upstream products and industries in the industrial chain of P provide P for the products produced by the downstream industries. Primary input represents the input of P from four natural media to the socioeconomic system. The primary supplier to P supply chain indicates the product through which P enters the socioeconomic system.
Building physical SUTs
The SUT is an essential part of the System of National Accounts of a nation (Mahajan et al. Citation2018). It includes the supply table and the use table. The supply table reflects the total supply of various goods and services, whereas the use table reflects the total use of goods and services in the economy. The supply table includes total output, which is the value of all goods and services produced in the study area in a given period, reflecting the total scale of its production activities. The use table includes three parts: intermediate input (which reflects the structure of the production input), final use (such as net export and stock), and added value (primary inputs of nature resource). The basic structures of the supply table and the use table are shown in and , where the gray part has no data.
Table 1. Framework of the supply table.
Table 2. Framework of the use table.
Converting SUTs into PIOTs
SUTs were converted into PIOTs using methods employed in the Eurostat Manual (Eurostat Citation2008) and the Handbook (Mahajan et al. Citation2018) of SUTs (Miller and Blair Citation2021). The P SUTs were built by balancing the input and output data of China’s economic products. The transformation of SUTs into PIOTs refers to the method in Zhong et al. (Citation2022). A P cycle network was established for 15 industries and 83 products. The basic form of the PIOT is shown in .
Table 3. Framework of the physical input-output table.
Production-based and supply-side P emissions
Production-based emissions refer to emissions directly generated during production. Supply-side accounting studies the role of a product or country as a primary supplier to supply chain. A product’s supply-side P emissions include the direct P emissions due to production activities of this product enabled by its added value, as well as the indirect P emissions occurring in downstream products because of its inputs (e.g., natural P inputs from the phosphate rocks product enable downstream production and therefore, associated P emissions from fertilizer production).
The production-based P emissions and total output in China were obtained by collecting data and constructing PIOT. The emissions by unitary output of products can be calculated by EquationEquation (1)(1)
(1) :
Where e represents the P emissions per unit output of the products which denotes the intensity of P emissions by products; the column vector x represents the total output of each product; the symbol ep indicates production-based emissions.
Based on the column balance of PIOT, the Ghosh input-output model can be constructed to reflect the relationship between primary inputs and total inputs, considering that primary inputs drive downstream production (Miller and Blair Citation2021). The P flow within the economy is described from the perspective of supply using an environmentally extended Ghosh input-output model, and supply-side P emissions of China can be calculated using EquationEquations (2)(2)
(2) -(Equation3
(3)
(3) ):
Where EI indicates supply-side emissions. The vector v indicates the primary input of each product. The symbol G reflects the output of each economic system product as a direct and indirect result of a unit of primary input in a given product. The symbol ‘ means transposition. The matrix I is an identification matrix, and the block matrix B is the direct output coefficient matrix.
Structural decomposition analysis (SDA)
The SDA can quantify the relative contributions of various influencing factors to environmental pressure changes in a certain period. In this study, it is used to find the key socioeconomic factors that drive P emissions in the dynamic evolution process of the P cycle network from a supply perspective. The basic principle of SDA is to divide the overall change of a dependent variable in the economic system into changes in several independent variables, and calculate the contribution of each variable to the total change (Li, Gao, and Liu Citation2020; Liang et al. Citation2016, Citation2013). Using the newly prepared PIOTs for P, the P emissions were converted into a product of five factors according to the input-output model: emission intensity, production output structure, primary input structure, primary input level, and population. Emission intensity is obtained by dividing emissions by total output, reflecting the emissions per unit of physical output; production output structure represents the product allocation structure in the economic system; the primary input structure reflects how P is distributed from nature to the products; the primary input level indicates the quantity of P from soil, air, and water that is extracted by human beings. The amount of P emissions caused by the change in each socioeconomic factor during 1949–1978, 1978–1992, 1992–2002, 2002–2011, and 2011–2012 was determined.
The primary input matrix can be expressed as the product of primary input structure, primary input level and population size, as shown in EquationEquation (4)(4)
(4) :
where the vector vs indicates primary input structure, the parameter vL indicates primary input level and the parameter P indicates population size.
Therefore, the P emissions in a certain period can be expressed as,
Then, the change in P emissions can be expressed as shown in EquationEquation (6)(6)
(6) .
The notation ∆p represents the change in population size; the notation ∆vL represents the change in primary input level which equals to the total primary input divided by the population of China in a particular year; the notation ∆vs represents the change in primary input structure which equals the proportion of each product in the primary input; the notation ∆G represents the change in production output structure, and the notation ∆e represents the change in P emission intensity.
SDA has a non-uniqueness problem in the process of the decomposition of variables. We address this by calculating the average of all possible forms of the first-order decomposition (Li, Gao, and Liu Citation2020; Wang, Cui, and Peng Citation2019; Peters et al. Citation2007; Guan et al. Citation2008).
Data sources
The system boundary of this study extends from natural subjects to include human subjects, which can be seen more intuitively from Figure S1. China’s P flow in the socioeconomic system was obtained from the P cycle model in recent studies by other scholars (Liu et al. Citation2016; Liang et al. Citation2020). The tables used in the calculations were obtained by working with the elements mentioned in the previous method section.
Sensitivity and uncertainty analysis
To verify the reliability of this study, a sensitivity analysis was performed on the calculated results using 2012 as an example (Heijungs Citation2010; Heijungs and Lenzen Citation2014). We calculate the sensitivity coefficients (Equationequations (7)(7)
(7) , (Equation9
(9)
(9) ), (Equation11
(11)
(11) ), (Equation13
(13)
(13) ) and (Equation15
(15)
(15) )) and elasticity coefficients (Equationequations (8)
(8)
(8) , (Equation10
(10)
(10) ), (Equation12
(12)
(12) ), (Equation14
(14)
(14) ) and (Equation16
(16)
(16) )).
The symbol ə indicates the derivative of the variable.
The elasticity of P emissions to the factors can be seen from the elasticity coefficients. Tables S1-S6 indicate that the results are relatively stable.
Robustness and evidence as well as confidence and consistency are used to further evaluate the overall quality of data (Salemdeeb et al. Citation2021). For details, please refer to SI.
Results
shows the change in P releases to the four environmental media (i.e., air, land, water and sea) in China during 1949–2012. Average annual P emissions were below 0.7 Mt (below 0.03 Mt to air, below 0.4 Mt to land, below 0.3 Mt to water, and 0.005 Mt to sea) until 1969. P emissions showed a prominent increase from 1985 to 2004, and the emissions rose steadily during 2004–2011. Then the emissions increased from 7.1 Mt in 2011 to 9.4 Mt in 2012. The percentage of P released into land rose from 21.9% in 1949 to 83.9% in 2012. Its increase reached nearly 7.8 Mt.
Figure 1. China’s P emissions to the environment (including air, land, water, and sea) from the supply perspective during 1949–2012.

Critical primary suppliers enabling P emissions
In 2012, cultivation products (especially rice, maize, and wheat) were the major sources of P emissions from the production perspective. Rice, maize, and wheat are important constituents of Chinese diet. The three products caused 1.4 Mt, 1.4 Mt, and 1.0 Mt of P emissions, respectively, collectively accounting for about 40.0% of the total P emissions. The waste disposal services product was also an important emitter accounting for 1.0 Mt of the total P emissions. Rapid socioeconomic development is often accompanied by the generation of huge amounts of waste, which contains a large amount of P, especially in the sludge left over from sewage treatment.
The key product enabling P emissions from the supply perspective was related to phosphate rocks from mining (). This product accounted for 8.0 Mt of P emissions in 2012 (representing 85.6% of the total emissions). Moreover, it is estimated that 84.9% of these emissions were to the land. In the years prior to 1954, the P emissions of the phosphate rocks product from the supply-side perspective had virtually no emissions. This is probably because that China only began to develop phosphate fertilizers and promote their use in cultivation using domestically produced phosphate rocks around the 1950s. Emissions of phosphate rocks from the supply perspective are mainly released into the land (). China is one of the world’s largest phosphate rocks consumers. Phosphate rocks is mainly used to produce P chemicals such as phosphate fertilizer. P mining increased, especially after 1970, due to the increasing manufacture of phosphate fertilizer. The annual production of phosphate rocks was 3.9 Mt in 1970 and increased to 95.3 Mt by 2012.
Figure 2. Production perspective and supply perspective P emissions of products in China in 2012. The percentages in the figure indicate P emissions from products as a percentage of total P emissions from the two perspectives.

Other key products responsible for China’s P emissions from the supply side mainly include food crops (0.1 Mt in 2012) and livestock manure (1.0 Mt in 2012). It is noteworthy that supply-side P emissions of livestock products, particularly related to excreta from pig and excreta from cow, changed dramatically over time (). China is the largest livestock producer worldwide, accounting for over 30% of global meat production (Jia et al. Citation2018). The average daily production of pigs in 2012 was 11.1 times the value in 1949, while that of cattle was 3.4 times, which influenced the amount of P emissions. Emissions from this product were mainly to land. Livestock manure is a significant source of P emissions, and its use by farmers for the fertilization of farmland results in massive P releases to land and inland water bodies (Sheldrick, Keith Syers, and Lingard Citation2003; Chadwick et al. Citation2015). Suppliers of animal husbandry are raising livestock in large numbers as technology advances and human living standards improve, resulting in a rapidly growing number of livestock in China. It is believed that the dramatic increase in population caused suppliers of fertilizer production to increase the amount of fertilizer (including phosphate fertilizer and manure) used to increase grain production. Cattle and pigs were the only livestock raised in large numbers in China from 1949 to 1978 (Chadwick et al. Citation2015). Other livestock species were not introduced until the late 1970s to around 1996 when China opened its livestock market (Chadwick et al. Citation2015)().
Key supply-side drivers influencing P emission changes
The key socioeconomic factors that influenced the change in P emissions during the period of 1949–2012 were identified. shows the relative contributions of socioeconomic factors to China’s P emission changes between every successive pair of years from 1949 to 2012 from the supply perspective. We divided the whole study period (i.e., 1949–2012) into five stages (1949–1978, 1978–1992, 1992–2002, 2002–2011, and 2011–2012) according to the development of China’s environmental protection policies. SDA was conducted to analyze the influence of five socioeconomic factors on P emissions changes, namely, emission intensity, production output structure, primary input structure, primary input level, and population.
Figure 5. Relative contributions of socioeconomic factors to China’s P emission changes during different time periods from the supply perspective.

Overall, the change in the primary input level is the key driving force for the increase in P emissions during 1949–2012, followed by the change in population, emission intensity, and primary input structure (). These four socioeconomic factors collectively drove the increase in P emissions by 11.3 Mt. The change in production output structure was the only key driver of the reduction, driving a 2.2 Mt reduction in P emissions.
Figure 6. Relative contributions of socioeconomic factors to China’s P emission changes from the supply perspective during 1949–2012.

From the founding of the People’s Republic of China in 1949 to the reform and opening-up in 1978, China’s industrial pollution prevention measures and environmental regulation policy systems were in the preliminary exploration and construction stage. During this period, the overall trend of P emissions showed a slow rise (+ 0.3 Mt). The change in primary input level was the most critical driver of the increment. On the other hand, changes in the production output structure, emission intensity, and primary input structure drove a reduction in P emissions by 0.3 Mt, 0.2 Mt, and 0.1 Mt, respectively. Emission intensity of cultivation products such as wheat is 42.4% lower than in 1949, driving P reductions. The change in the primary input structure is mainly due to the significantly higher share of phosphate rocks, which was 0.1% in 1949 and 67.7% in 1978.
China’s P emissions increased from 0.6 Mt in 1978 to 2.9 Mt in 1992. The driving forces of the increased in P emission were the changes in emission intensity (+1.1 Mt), production output structure (+0.1 Mt), primary input level (+0.8 Mt), and population (+0.3 Mt). During this period, the emission intensity from cultivation products such as grains increased by 143.3%; the emission intensity of manure such as excreta from cattle and excreta from poultry had tripled; besides, the emission intensity from wastewater treatment services had doubled, all of which contributed to the increase in P emissions. The change in the primary input structure drove a slight decrease of 0.02 Mt. In 1978, China’s phosphate fertilizer industry simultaneously implemented an independent development as well as a technology upgradation policy, and began to adjust the product structure, which led to the aforementioned decrease in P emissions (Xu et al. Citation2021).
China’s P emissions increased to 5.6 Mt in 2002. The change in primary input level was the key driving force of P emission reduction, followed by the primary input structure, and their changes collectively drove a P emission reduction of 0.2 Mt. The factors that drove this increase were changes in the production output structure (+1.6 Mt), emission intensity (+0.9 Mt), and population (+0.4 Mt). Total P emissions declined briefly in 1993, mainly due to reduced emission intensity of wastewater treatment services and cultivation products. Between 1997–1998 and 1999–2000, changes in the production output structure and primary input levels significantly drove an increase of 0.8 Mt and a decrease of 0.8 Mt in P emissions, respectively. Overall, changes in the primary input level and primary input structure collectively contributed to a 0.2 Mt reduction in P emission reduction during 1992–2002.
While there was a significant increase in P emissions due to some factors (+1.5 Mt) during 2002–2011 (), the total P emissions increased by 1.5 Mt mainly due to changes in the primary input level (+6.3 Mt). Changes in population and primary input structure further drove an increase in emissions (0.3 Mt and 0.2 Mt, respectively). The factors contributing to a decrease in P emissions were changes in the production output structure (−4.1 Mt) and emission intensity (−1.3 Mt).
China’s P emissions increased from 7.1 Mt in 2011 to 9.4 Mt in 2012. This significant increase in a timespan of just one year was mainly related to the changes in primary input level (1.1 Mt) and emission intensity (0.8 Mt). Phosphate rocks increased by 14.1 Mt during the year, resulting in a 14.6% increase in primary input level of phosphate rocks. The increase in P emissions driven by emission intensity is mainly due to a 19.4% rise in the emission intensity of cultivation products such as rice compared to 2011. Changes in production output structure and population further drove 0.4 Mt and 0.04 Mt of increases in emissions, respectively. A change in the primary input structure drove a slight decrease in the total P emission (−0.05 Mt).
Discussion
The main contribution of this study is that it identified the key primary suppliers to P supply chain and revealed how the primary inputs lead to P emissions by a supply-side accounting and an analysis of the socioeconomic drivers of P emissions. Different from the production-side and consumption-side analysis of P emissions, the supply-side analysis complements the literature in this area and provides policy suggestions on P emissions reduction and shared responsibility design for the sustainable management of P.
Previous research mainly focused on direct emissions of P from Wastewater Treatment in China. Jiang et al. found that population contributed the most to China’s P flow changes during 1961–2014 from the production and consumption perspectives using the STIRPAT model. While important direct emitters are identified from the production perspective, supply-side analysis reveals the products whose primary inputs enable production and associated P emissions from downstream products. The degree of responsibility for environmental stress depends on the choice of liability principles, and the use of different principles can produce different results, mainly due to the different boundaries used (Miller and Blair Citation2021). In contrast to the production perspective where only the direct emissions from production activities of a product are considered, the supply-side calculation considers the entire supply chain and takes into account both direct and indirect emissions enabled by the primary inputs of a product (Liang et al. Citation2016; Marques et al. Citation2012; Liang et al. Citation2017; Zhang et al. Citation2018). The supply-side analysis additionally reveals products whose direct P emissions may not be significant, but whose inputs enable downstream production and associated P emissions. In this avenue, the focus of policy decisions is on direct emission control at the end of supply chains. For example, promoting resource utilization in sewage treatment and solid waste treatment. Supply-side accounting, on the other hand, identifies key products whose primary input led to a large amount of downstream P emissions (such as in the case of the phosphate rocks product) and explores how primary supply drives P emissions down the chain. Based on such findings, policymakers can reduce primary inputs (such as phosphate rock) and support for products or enterprises of products with low supply-side P emissions. For instance, subsidies and vigorous publicity may be used to promote enterprises with less P emissions, while taxes may be imposed on enterprises with high P emissions. This study, therefore, provides important revelations for multi-perspective P reduction policy decisions, which are not possible with merely production-based accounting.
Moreover, we investigated the relative contributions of socioeconomic factors to the changes in China’s P emissions during 1949–2012 using SDA. It is worth noting that this study analyzes P emissions in terms of socioeconomic impacts (i.e., P supply chains). The scope of this study focuses on the socioeconomic factors and key products that influence P emissions from a macro perspective. It can better raise awareness of industry-wide P emission reduction and provide macro and targeted regulation suggestions for policy decisions. There are other factors influencing P emissions which are not considered in this study, such as path curbs, field ridges, and human perturbation (Yan et al. Citation2021; Yuan et al. Citation2021). The impacts of other factors on P emissions can be explored in future studies. Cooper and Carliell-Marquet (Citation2013) showed that reducing loss to water and soil had the greatest potential for reducing P emissions in the UK . Meanwhile, some studies have shown that cultivation and the application of animal manure contributed the most to soil and water of P losses (Wang et al. Citation2018; Liu et al. Citation2008; Cooper and Carliell-Marquet Citation2013). Based on these findings, they advocated strengthening P recycling (Liu et al. Citation2020; Cooper and Carliell-Marquet Citation2013). Consistent with previous researches, this research also showed that soil and water are the main carriers of P emissions. Moreover, this study further revealed that the acquisition of phosphate rocks from the soil is the main driving force of downstream P emission. Due to the requirements of phosphate rock as raw materials for industries as well as in the form of fertilizers to cultivation products, it occupies an important position in economic development in China and other countries. Considering the non-renewability of phosphate rock and its influence on downstream P emission, it is suggested to reduce the dependence on phosphate fertilizer. Furthermore, due to limited purification technology, a large amount of unsatisfactory phosphate rock is abandoned or lost to the environment when phosphate mining is conducted (Li et al. Citation2016). We propose to reduce phosphate mining while ensuring that economic needs are met, for example, by promoting the application of advanced sorting and purification technology and improving the comprehensive utilization consciousness of relevant enterprises. In addition, abandoned phosphate rock should be stored pending the possibility of reuse.
Moreover, reducing emission intensity and changing the primary input structure can play an important role in driving P emission reductions. With regard to emission intensity, key focus products should be phosphate rocks, wastewater treatment services, and cultivation products. Raising and maintaining appropriate wastewater treatment discharge standards or introducing new water treatment technologies can reduce the P discharge intensity of wastewater treatment plants. In the case of cultivation products (such as rice), the use of P in the form of fertilizers needs to be reduced. At present, there is the problem of excessive fertilization in cultivation product, which not only increases the possibility of eutrophication but also increases the P emission intensity of cultivation products. Primary inputs to the phosphate rocks product increase year on year, leading to its higher proportion in the nation’s primary input structure. This product then enables downstream P emissions from cultivation sectors due to the use of phosphate fertilizer. Policy makers can reduce the pressure on P resources by improving the efficiency of recycling P resources.
Furthermore, the production output structure must be improved by optimizing the supply allocation of downstream products. For example, improving the fertility of the soil itself to reduce the supply of phosphate fertilizers to the cultivation industry. Additionally, downstream products with lower P emission intensity should be chosen in production activities, which will help reduce the total P emissions.
Conclusions
This study reveals the impact of the upstream economic activities of the P supply chain by human beings on P emissions in China. We first constructed PIOTs for P from 1949 to 2012, based on mass balance and input-output analysis, and investigated the crucial suppliers for P emissions. Then we quantified the relative contributions of various socioeconomic factors affecting P emissions during 1949–2012 using the SDA method. The study offered a new insight into the regulatory control of P emissions.
The results show that phosphate rock was the dominant primary supplier to P supply chain in 2012, but it was not influential from the production perspective. The increase in phosphate rock causes a change in the primary input structure, leading to emissions downstream from the P supply chain. The changes in primary input level was the key driver of increased P emissions based on the supply perspective during 1949–2012. It is recommended to improve technology and consciousness of integrated use in mining, and support enterprises or sectors with low supply-side P emissions. Phosphate rocks, wastewater treatment services, and agricultural products are significant sectors driving P emission reductions by lowering emission intensities, and thus further improvements in wastewater treatment efficiency are needed. Reducing the supply of phosphate fertilizer to the agricultural growing industry can promote changes in the production output structure and thus reduce P emissions, which can be achieved, for instance, by improving soil fertility. These findings can support the supply-side policy decisions regarding P emission control and provide further understanding for sustainable development.
This study is not without limitations. Although an uncertainty analysis has been made to the overall quality of the data in this study, there is still room to conduct a comprehensive and quantitative analysis of how the results will vary due to the uncertainty of all the inputs. Given the many activity data and parameters involved in the calculations, such an uncertainty analysis work is very difficult and deserves another work in the future. In addition to that, updating the time scale to the most recent year would also be a potential future work.
Acknowledgments
Supplemental Material
Download MS Word (962.8 KB)Disclosure statement
No potential conflict of interest was reported by the author(s).
Supplementary material
Supplemental data for this article can be accessed online at https://doi.org/10.1080/20964129.2022.2107578
Additional information
Funding
References
- Antikainen, R., R. Lemola, J. I. Nousiainen, L. Sokka, M. Esala, P. Huhtanen, and S. Rekolainen . 2005. “Stocks and Flows of Nitrogen and Phosphorus in the Finnish Food Production and Consumption System.” Agriculture, Ecosystems & Environment 107 (2–3): 1–11. doi:10.1016/j.agee.2004.10.025.
- Bai, Z. H., L. Ma, W. Ma, W. Qin, G. L. Velthof, O. Oenema, and F. Zhang . 2016. “Changes in Phosphorus Use and Losses in the Food Chain of China during 1950–2010 and Forecasts for 2030.” Nutrient Cycling in Agroecosystems 104 (3): 361–372. doi:10.1007/s10705-015-9737-y.
- Carpenter, S. R., N. F. Caraco, D. L. Correll, R. W. Howarth, A. N. Sharpley, and V. H. Smith . 1998. “Nonpoint Pollution of Surface Waters with Phosphorus and Nitrogen.” Ecological Applications 8 (3): 559–568. doi:10.1890/1051-0761(1998)008[0559:NPOSWW]2.0.CO;2.
- Chadwick, D., J. Wei, T. Yan’an, Y. Guanghui, S. Qirong, and C. Qing . 2015. “Improving Manure Nutrient Management Towards Sustainable Agricultural Intensification in China.” Agriculture, Ecosystems & Environment 209: 34–46. doi:10.1016/j.agee.2015.03.025.
- Chen, M., J. Chen, and F. Sun. 2008. “Agricultural Phosphorus Flow and Its Environmental Impacts in China.” Science of the Total Environment 405 (1–3): 140–152. doi:10.1016/j.scitotenv.2008.06.031.
- Chen, M. P., and T. E. Graedel. 2016. “A half-century of Global Phosphorus Flows, Stocks, Production, Consumption, Recycling, and Environmental Impacts.” Global Environmental Change 36: 139–152. doi:10.1016/j.gloenvcha.2015.12.005.
- China, M.o.E.a.E.o.t.P.s.R.o. 2021 “2020 Report on the State of the Ecology and Environment in China.” Ministry of Ecology and Environment, 30.
- Cooper, J., and C. Carliell-Marquet. 2013. “A Substance Flow Analysis of Phosphorus in the UK Food Production and Consumption System.” Resources, Conservation and Recycling 74: 82–100. doi:10.1016/j.resconrec.2013.03.001.
- Cordell, D., J.-O. Drangert, and S. White. 2009. “The Story of Phosphorus: Global Food Security and Food for Thought.” Global Environmental Change 19 (2): 292–305. doi:10.1016/j.gloenvcha.2008.10.009.
- Eurostat. 2008. “Eurostat Manual of Supply Use and Input-Output Tables.” 51–53, 295–297,348–349.
- Guan, D., K. Hubacek, C. L. Weber, G. P. Peters, and D. M. Reiner . 2008. “The Drivers of Chinese CO2 Emissions from 1980 to 2030.” Global Environmental Change 18 (4): 626–634. doi:10.1016/j.gloenvcha.2008.08.001.
- Heijungs, R. 2010. “Sensitivity Coefficients for matrix-based LCA.” The International Journal of Life Cycle Assessment 15 (5): 511–520. doi:10.1007/s11367-010-0158-5.
- Heijungs, R., and M. Lenzen. 2014. “Error Propagation Methods for LCA-a Comparison.” International Journal of Life Cycle Assessment 19 (7): 1445–1461. doi:10.1007/s11367-014-0751-0.
- Hou, Y., L. Ma, Z. L. Gao, F. H. Wang, J. T. Sims, W. Q. Ma, and F. S. Zhang . 2013. “The Driving Forces for Nitrogen and Phosphorus Flows in the Food Chain of China, 1980 to 2010.” Journal of Environmental Quality 42 (4): 962–971. doi:10.2134/jeq2012.0489.
- Huang, J., -C.-C. Xu, B. G. Ridoutt, X.-C. Wang, and P.-A. Ren . 2017. “Nitrogen and Phosphorus Losses and Eutrophication Potential Associated with Fertilizer Application to Cropland in China.” Journal of Cleaner Production 159: 171–179. doi:10.1016/j.jclepro.2017.05.008.
- Hunter, T. 2012. “Why Nature Chose Phosphate to Modify Proteins.” Philosophical Transactions of the Royal Society B-Biological Sciences 367 (1602): 2513–2516. doi:10.1098/rstb.2012.0013.
- Jeong, Y.-S., K. Matsubae-Yokoyama, H. Kubo, -J.-J. Pak, and T. Nagasaka . 2009. “Substance Flow Analysis of Phosphorus and Manganese Correlated with South Korean Steel Industry.” Resources, Conservation and Recycling 53 (9): 479–489. doi:10.1016/j.resconrec.2009.04.002.
- Jia, W., W. Qin, Q. Zhang, X. Wang, Y. Ma, and Q. Chen . 2018. “Evaluation of Crop Residues and Manure Production and Their Geographical Distribution in China.” Journal of Cleaner Production 188: 954–965. doi:10.1016/j.jclepro.2018.03.300.
- Jiang, S. Y., H. Hua, H. Sheng, H. P. Jarvie, X. Liu, Y. Zhang, Z. Yuan, Zhang, L., and Liu, X. . 2019. “Phosphorus Footprint in China over the 1961–2050 Period: Historical Perspective and Future Prospect.” Science of the Total Environment 650: 687–695. doi:10.1016/j.scitotenv.2018.09.064.
- Kalmykova, Y., R. Harder, H. Borgestedt, and I. Svanäng . 2012. “Pathways and Management of Phosphorus in Urban Areas.” Journal of Industrial Ecology 16 (6): 928–939. doi:10.1111/j.1530-9290.2012.00541.x.
- Li, M., Y. Gao, and S. Liu. 2020. “China’s Energy Intensity Change in 1997–2015: Non-vertical Adjusted Structural Decomposition Analysis Based on input-output Tables.” Structural Change and Economic Dynamics 53: 222–236. doi:10.1016/j.strueco.2020.03.001.
- Li, G., M. K. van Ittersum, P. A. Leffelaar, S. Z. Sattari, H. Li, G. Huang, and F. Zhang . 2016. “A multi-level Analysis of China’s Phosphorus Flows to Identify Options for Improved Management in Agriculture.” Agricultural Systems 144: 87–100. doi:10.1016/j.agsy.2016.01.006.
- Li, S. S., Z. Yuan, J. Bi, and H. Wu . 2010. “Anthropogenic Phosphorus Flow Analysis of Hefei City, China.” Science of the Total Environment 408 (23): 5715–5722. doi:10.1016/j.scitotenv.2010.08.052.
- Liang, S., S. Qu, Z. Zhu, D. Guan, and M. Xu . 2017. “Income-Based Greenhouse Gas Emissions of Nations.” Environmental Science & Technology 51 (1): 346–355. doi:10.1021/acs.est.6b02510.
- Liang, S., H. Wang, S. Qu, T. Feng, D. Guan, H. Fang, M. Xu, et al. 2016. “Socioeconomic Drivers of Greenhouse Gas Emissions in the United States.” Environmental Science & Technology 50 (14): 7535–7545. doi:10.1021/acs.est.6b00872.
- Liang, S., M. Xu, Z. Liu, S. Suh, and T. Zhang . 2013. “Socioeconomic Drivers of Mercury Emissions in China from 1992 to 2007.” Environmental Science & Technology 47 (7): 3234–3240. doi:10.1021/es303728d.
- Liang, S., Y. Yu, A. Kharrazi, B. D. Fath, C. Feng, G. T. Daigger, S. Chen, Ma, T., Zhu, B., Mi, Z., and Yang, Z. . 2020. “Network Resilience of Phosphorus Cycling in China Has Shifted by Natural Flows, Fertilizer Use and Dietary Transitions between 1600 and 2012.” Nature Food 1 (6): 365–375. doi:10.1038/s43016-020-0098-6.
- Liu, X., H. Sheng, S. Jiang, Z. Yuan, C. Zhang, and J. J. Elser . 2016. “Intensification of Phosphorus Cycling in China since the 1600s.” Proceedings of the National Academy of Sciences of the United States of America 113 (10): 2609–2614. doi:10.1073/pnas.1519554113.
- Liu, Y., G. Villalba, R. U. Ayres, and H. Schroder . 2008. “Global Phosphorus Flows and Environmental Impacts from a Consumption Perspective.” Journal of Industrial Ecology 12 (2): 229–247. doi:10.1111/j.1530-9290.2008.00025.x.
- Liu, X. W., Z. Yuan, X. Liu, Y. Zhang, H. Hua, and S. Jiang . 2020. “Historic Trends and Future Prospects of Waste Generation and Recycling in China’s Phosphorus Cycle.” Environmental Science & Technology 54 (8): 5131–5139. doi:10.1021/acs.est.9b05120.
- Ma, W., L. Ma, J. Li, F. Wang, I. Sisák, and F. Zhang . 2011. “Phosphorus Flows and Use Efficiencies in Production and Consumption of Wheat, Rice, and Maize in China.” Chemosphere 84 (6): 814–821. doi:10.1016/j.chemosphere.2011.04.055.
- Mahajan, S., Beutel, J., Guerrero, S., and Inomata, S., et al. 2018. Handbook on Supply, Use and Input-Output Tables with Extensions and Applications (UNITED NATIONS PUBLICATION, Department of Economic and Social Affairs, Statistics Division), 321–336.
- Marques, A., J. Rodrigues, M. Lenzen, and T. Domingos . 2012. “Income-based Environmental Responsibility.” Ecological Economics 84: 57–65. doi:10.1016/j.ecolecon.2012.09.010.
- Miller, R. E., and P. D. Blair. 2021. Input-Output Analysis: Foundations and Extensions (United States of America: Cambridge University Press).
- Peters, G. P., C. L. Weber, D. Guan, and K. Hubacek . 2007. “China’s Growing CO2 EmissionsA Race between Increasing Consumption and Efficiency Gains.” Environmental Science & Technology 41 (17): 5939–5944. doi:10.1021/es070108f.
- Salemdeeb, R., R. Saint, W. Clark, M. Lenaghan, K. Pratt, and F. Millar . 2021. “A Pragmatic and industry-oriented Framework for Data Quality Assessment of Environmental Footprint Tools.” Resources, Environment and Sustainability 3: 100019. doi:10.1016/j.resenv.2021.100019.
- Senthilkumar, K., Nesme, T., Mollier, A., and Pellerin, S. . 2012. “Conceptual Design and Quantification of Phosphorus Flows and Balances at the Country Scale: The Case of France.” Global Biogeochemical Cycles 26. doi:10.1029/2011GB004102.
- Sheldrick, W., J. Keith Syers, and J. Lingard. 2003. “Contribution of Livestock Excreta to Nutrient Balances.” Nutrient Cycling in Agroecosystems 66 (2): 119–131. doi:10.1023/A:1023944131188.
- Smil, V. 2000. “PHOSPHORUS IN THE ENVIRONMENT: Natural Flows and Human Interferences.” Annual Review of Energy and the Environment 25 (1): 53–88. doi:10.1146/annurev.energy.25.1.53.
- Smith, L. E. D., and G. Siciliano. 2015. “A Comprehensive Review of Constraints to Improved Management of Fertilizers in China and Mitigation of Diffuse Water Pollution from Agriculture.” Agriculture, Ecosystems & Environment 209: 15–25. doi:10.1016/j.agee.2015.02.016.
- Suh, S., and S. Yee. 2011. “Phosphorus use-efficiency of Agriculture and Food System in the US.” Chemosphere 84 (6): 806–813. doi:10.1016/j.chemosphere.2011.01.051.
- Villalba, G., Y. Liu, H. Schroder, and R. U. Ayres . 2008. “Global Phosphorus Flows in the Industrial Economy from a Production Perspective.” Journal of Industrial Ecology 12 (4): 557–569. doi:10.1111/j.1530-9290.2008.00050.x.
- Wang, Z., C. Cui, and S. Peng. 2019. “How Do Urbanization and Consumption Patterns Affect Carbon Emissions in China? A Decomposition Analysis.” Journal of Cleaner Production 211: 1201–1208. doi:10.1016/j.jclepro.2018.11.272.
- Wang, M. R., L. Ma, M. Strokal, W. Ma, X. Liu, and C. Kroeze . 2018. “Hotspots for Nitrogen and Phosphorus Losses from Food Production in China: A County-Scale Analysis.” Environmental Science & Technology 52 (10): 5782–5791. doi:10.1021/acs.est.7b06138.
- Wang, F., J. T. Sims, L. Ma, W. Ma, Z. Dou, and F. Zhang . 2011. “The Phosphorus Footprint of China’s Food Chain: Implications for Food Security, Natural Resource Management, and Environmental Quality.” Journal of Environmental Quality 40 (4): 1081–1089. doi:10.2134/jeq2010.0444.
- Wu, H., Z. Yuan, Y. Zhang, L. Gao, and S. Liu . 2014. “Life-cycle Phosphorus Use Efficiency of the Farming System in Anhui Province, Central China.” Resources, Conservation and Recycling 83: 1–14. doi:10.1016/j.resconrec.2013.12.002.
- Xu, D., Zhong, B., Wang, X., Li, X., Zhong, Y., Yan, Z., Yang, J., Li, X., Wang, Y., and Zhou, X. . 2021. “The Development Road of Ammonium Phosphate Fertilizer in China.” Chinese Journal of Chemical Engineering. doi:10.1016/j.cjche.2021.08.015.
- Yan, K., J.-C. Xu, W. Gao, M.-J. Li, Z.-W. Yuan, F.-S. Zhang, and J. Elser . 2021. “Human Perturbation on Phosphorus Cycles in One of China’s Most Eutrophicated Lakes.” Resources, Environment and Sustainability 4: 100026. doi:10.1016/j.resenv.2021.100026.
- Yuan, Z. W., S. Jiang, H. Sheng, X. Liu, H. Hua, X. Liu, and Y. Zhang . 2018. “Human Perturbation of the Global Phosphorus Cycle: Changes and Consequences.” Environmental Science & Technology 52 (5): 2438–2450. doi:10.1021/acs.est.7b03910.
- Yuan, Z., Y. Pang, J. Gao, X. Liu, H. Sheng, and Y. Zhuang . 2021. “Improving Quantification of Rainfall Runoff Pollutant Loads with Consideration of Path Curb and Field Ridge.” Resources, Environment and Sustainability 6: 100042. doi:10.1016/j.resenv.2021.100042.
- Zhang, H. R., L. Chen, Y. Tong, W. Zhang, W. Yang, M. Liu, L. Liu, Wang, H., Wang, X. . 2018. “Impacts of Supply and Consumption Structure on the Mercury Emission in China: An input-output Analysis Based Assessment.” Journal of Cleaner Production 170: 96–107. doi:10.1016/j.jclepro.2017.09.139.
- Zhong, Q. R. Huang, Yu, Y., Feng, C., S. Liang . 2022. “Phosphorus Emissions from Changing Phosphorus Supply Chain Networks in China.” Journal of Cleaner Production, 369: 133259 . doi:10.1016/j.jclepro.2022.133259 .