ABSTRACT
The severe acute respiratory syndrome coronavirus 2 (SARS-CoV-2) pandemic has posed enormous challenges to global public health. The use of antibiotics has greatly increased during the SARS-CoV-2 epidemic owing to the presence of bacterial co-infection and secondary bacterial infections. The antibiotics daptomycin (DAP) is widely used in the treatment of infectious diseases caused by gram-positive bacteria owing to its highly efficient antibacterial activity. It is pivotal to study the antibiotics usage options for patients of coronavirus infectious disease (COVID-19) with pneumonia those need admission to receive antibiotics treatment for bacterial co-infection in managing COVID-19 disease. Herein, we have revealed the interactions of DAP with the S protein of SARS-CoV-2 and the variant Omicron (B1.1.529) using the molecular docking approach and Omicron (B1.1.529) pseudovirus (PsV) mimic invasion. Molecular docking analysis shows that DAP has a certain degree of binding ability to the S protein of SARS-CoV-2 and several derived virus variants, and co-incubation of 1–100 μM DAP with cells promotes the entry of the PsV into human angiotensin-converting enzyme 2 (hACE2)-expressing HEK-293T cells (HEK-293T-hACE2), and this effect is related to the concentration of extracellular calcium ions (Ca2+). The PsV invasion rate in the HEK-293T-hACE2 cells concurrently with DAP incubation was 1.7 times of PsV infection alone. In general, our findings demonstrate that DAP promotes the infection of PsV into cells, which provides certain reference of antibiotics selection and usage optimization for clinicians to treat bacterial coinfection or secondary infection during SARS-CoV-2 infection.
Introduction
The severe acute respiratory syndrome coronavirus 2 (SARS-CoV-2) pandemic has brought huge challenges to global public health in the past four years. More than 774 million confirmed coronavirus infectious disease (COVID-19) cases have been reported, including over 7.03 million deaths, as of March 2024, and these data are still increasing according to the statistics of the World Health Organization (WHO) (https://www.who.int/emergencies/diseases/novel-coronavirus-2019). SARS-CoV-2 not only causes clinical symptoms of pneumonia, including fever, fatigue, cough, and dyspnoea, but it can also severely affect the heart, gastrointestinal system, liver, kidney, and central nervous system, eventually leading to multiple organ failure [Citation1,Citation2]. In fact, the rate of human-to-human transmission of SARS-CoV-2 is higher than that of the previously emerged and endemic resulting coronaviruses, including severe acute respiratory syndrome (SARS) and Middle East respiratory syndrome (MERS) [Citation3].
SARS-CoV-2 is an enveloped single-stranded positive-sense RNA virus [Citation4,Citation5], and SARS-CoV-2 viral genome encodes four main structural proteins, including spike (S) surface glycoprotein, membrane (M) protein, envelope (E) protein, and nucleocapsid (N) protein [Citation1]. The N protein forms the capsid outside the genome, and the viral genome is further packed by an envelope associated with the other three structural proteins [Citation6]. The S-protein binds to the angiotensin-converting enzyme 2 (ACE2) receptor protein located on the host cell surface membrane to mediate SARS-CoV-2 invasion into host cells [Citation7], which is a complicated process involving different domains of the S-protein interacting with host cells and other intracellular and extracellular components [Citation8]. Efficiency in each step could contribute to the virulence and infectivity of SARS-CoV-2 [Citation9].
Since the first SARS-CoV-2 variant of concern (VOC) Alpha (B.1.1.7) appeared in December 2020, there have been multiple VOCs, including Beta (B.1.351), Gamma (P.1), Delta (B.1.617.2), and Omicron (B.1.1.529) [Citation10]. Omicron was first discovered in South Africa in November 2021 [Citation11], and multiple Omicron subvariants are more infectious due to the S-protein carrying up to 32 mutations [Citation12] compared to the previously discovered devastating Delta variant with only nine mutations [Citation11]. In recent months, the Omicron variant has continued to expand into multiple subvariants, including BA.2, BA.2.12.1, BA.4, BA.5, and XBB, which have enhanced transmissibility, resulting in many infections and attracting global attention [Citation13,Citation14].
The SARS-CoV-2 pandemic has led to an increase in the use of antibiotics [Citation15]. More than half of SARS-CoV-2 patients may receive antibiotics, and this number can be higher in hospitalized patients and those with severe disease [Citation16]. There could be several reasons for this: (i) The symptoms of SARS-CoV-2, similar to community-acquired bacterial pneumonia, and doctors add antibiotics empirically. (ii) Bacterial co-infection in patients with SARS-CoV-2 and secondary infections in hospitalized patients had led to the heavy use of antibiotics [Citation17]. (iii) Antibiotics are necessary to treat bacterial co-infections with other respiratory pathogens in patients with SARS-CoV-2.
Antibiotics are not approved for treating SARS-CoV-2 infection [Citation18], and misuse of antibiotics may lead to antibiotic resistance [Citation19]. A meta-analysis of 4721 SARS-CoV-2 infecting patients showed that 2482 patients received 3058 antibiotic agents, with fluoroquinolones being the most used, followed by macrolides, beta-lactamases, cephalosporins, vancomycin (VAN), carbapenems, and tetracyclines [Citation20]. Another study on antibiotic use in a Spanish tertiary care hospital during the COVID-19 pandemic from March to June 2020 found that ceftriaxone, azithromycin, daptomycin (DAP), carbapenems, and linezolid consumption increased, and these findings were more evident in the ICU [Citation21].
DAP is a broad-spectrum polypeptide antibiotic used to treat infections caused by gram-positive bacteria, including complicated skin and skin structure infections (cSSSIs), right-sided infective endocarditis, and bacteraemia associated with cSSSIs or right-sided infective endocarditis [Citation22]. DAP at a dose of 6 mg/kg per day has not less effective than standard treatment for Staphylococcus aureus bacteraemia and right-sided endocarditis [Citation23]. And once-daily 5–10 mg/kg DAP is well tolerated, with safety and efficacy comparable to standard-of-care in children/adolescents with cSSSIs caused by gram-positive pathogens [Citation24]. In a phase 3 trial involving patients with complicated Staphylococcus aureus bacteraemia, a total of 136 of 198 patients (68.7%) in the DAP group had overall treatment success [Citation25]. Moreover, DAP exhibits highly effective in vitro antibacterial activity against most clinically important gram-positive bacteria, including methicillin-resistant Staphylococcus aureus (MRSA), VAN-resistant Enterococcus faecalis (VREF), and VAN-resistant Staphylococcus aureus (VRSA) [Citation26]. The minimal inhibitory concentrations (MIC) of DAP against MRSA, VREF and VRSA were 0.125, 0.5 [Citation27] and 0.5 μg/mL [Citation28] respectively. This antibacterial activity is a significant advantage in critically ill patients and has led to the increased use of DAP in severely ill patients with SARS-CoV-2 co-bacterial infection. For instance, a significant increase in DAP use was observed in ICU patients in April-May 2020 [Citation21]. However, other research has shown that DAP is not suitable for the treatment of pneumonia because it is inactivated by pulmonary surfactant [Citation22]. The antibacterial effect of DAP against Staphylococci decreased with increasing surfactant concentration when pulmonary surfactant was added, even the antibacterial potency of DAP was lost 16–32 fold when 1% surfactant was added [Citation29]. To date, the feasibility of DAP use for SARS-CoV-2 co-bacterial infection remains uncertain.
To study the effect of DAP on SARS-CoV-2 infection, we have the first time preliminarily evaluated the interaction between DAP with S-protein of SARS-CoV-2 and the variant Omicron (B1.1.529) through molecular docking, and further confirmed DAP contribution to the entry of S-protein-containing Omicron (Omicron/S, B1.1.529) pseudovirus (PsV) into cells. Cell invasion efficiency of PsV with 100 μM DAP incubation is 1.7 times of the PsV alone. Our data indicate that DAP is possibly not suitable for the treatment of bacterial co-infection patients with Omicron (B1.1.529) infection due to DAP interaction with the Omicron/S protein to promote Omicron (B1.1.529) invasion to host cells.
Materials and methods
Cell culture
Huh-7 and Vero-E6 cells were ordered from the Type Culture Collection of the Chinese Academy of Sciences (Shanghai, China). HEK-293T and HEK-293T-hACE2 cells were stored in State Key Laboratory of Biotherapy and Cancer Center, Sichuan University [Citation30]. All cells were cultured in DMEM (PM150210, Procell, China) supplemented with 10% foetal bovine serum (FSP500, ExCell Bio, China) and 1% penicillin-streptomycin under 5% CO2 at 37°C. The HEK-293T-hACE2 cell line stably expresses human angiotensin-converting enzyme 2 (hACE2) via hACE2 plasmid-containing lentivirus infection [Citation30].
Molecular docking
Molecular docking analysis was performed using HPEPDOCK software (http://huanglab.phys.hust.edu.cn/hpepdock/) [Citation31]. Antibiotics were docked to the S-protein of SARS-CoV-2 from the Protein Data Bank (https://www.rcsb.org/). Three antibiotics (DAP (PDB: 1xt7), VAN (PDB: 1qd8), and bleomycin (BLE, PDB: 1ugt)) were docked with SARS-CoV-2 viral structural proteins, including the S-protein of SARS-CoV-2 (PDB: 7kj2), S-protein of Alpha variant (B.1.1.7, PDB: 7lwv), S-protein of Beta variant (B.1.351, PDB: 7 lyo), S-protein of Gamma variant (P.1, PDB: 7m8k), S-protein of Delta variant (B.1.617.2, PDB: 7v7q), S-protein of Kappa variant (B.1.617.1, PDB: 7v7e), and S-protein of Omicron variant (B.1.1.529, PDB: 7qo7).
Antibiotics
DAP (MB1260, Melonepharma, China) and VAN (MB1073, Melonepharma, China) hydrochloride were dissolved in saline at the concentration of 10 mM, sterile filtered (0.22 µm), and stored in aliquots at −20°C until use.
Cell viability assay
The cytotoxicity of DAP and VAN on mammalian cell proliferation was detected using the CCK-8 reagent [Citation32]. 4 × 103 HEK-293T-hACE2, Huh-7, and Vero-E6 cells in the logarithmic growth phase were seeded into each well of a 96-well plate and cultured for 24 h at 37°C with 5% CO2. The supernatant was removed, and several series of different final concentrations of DAP or VAN were diluted in DMEM to incubate with cells for 48 h. Finally, DMEM containing 10% CCK-8 solution was added to replace the original medium and incubated for 1 h, and the absorption wavelength at 450 nm was detected with a reference wavelength of 630 nm to observe cell viability.
PsV construction
The gene encoding the green fluorescent protein (GFP) was inserted into the Xho I site of the plasmid pNL4–3-Luc-R-E (Beijing Tsingke Biotech Co., Ltd.) to construct the packaging plasmid pNL4–3-GFP-Luc-R-E. The cDNA (gi 2,200,734,308) encoding the S protein of Omicron (B1.1.529) was synthesized using a mammalian-optimized codon (Beijing Tsingke Biotech Co., Ltd.), which was then inserted between Hind III and Xba I sites of pcDNA3.1 [Citation33], and the recombinant expression plasmid was termed pcDNA3.1-Omicron/S.
In order to construct PsV, the expression plasmid pcDNA3.1-Omicron/S and the helper packaging plasmid pNL4–3-GFP-Luc-R-E at a ratio of 1:4 were co-transfected into HEK-293T cells for 48 h, and the culture medium supernatant was collected to concentrate PsV precipitation via filtering through 0.45 μm filter membrane by centrifugation at 72,000 × g for 2 h at 4°C. Finally, the PsV precipitate was collected and resuspended in fresh medium to determine the PsV titre.
PsV tittering method
The PsV titre was determined referencing dilution counting method [Citation34]. Generally, the original PsV solution was 10-fold serially diluted from 2 × 10−2 (1:50) to 2 × 10−7 (1:500 000) with DMEM medium. 196 µL DMEM was added to the first tube and 180 µL DMEM was added to the other five tubes. 4 µL of the original concentrated PsV was added to the first tube and mix well, from which 20 µL mixture was added to the second tube, and the other dilutions of PsV were similarly performed in other DMEM-containing tubes.
HEK-293T-hACE2 cells were cultured in a 96-well plate to cover 50% confluence, and medium was replaced with 200 µL of each diluted PsV solution to culture 24 h, then 200 µL fresh medium was added to per well. The expression of GFP was monitored under fluorescence microscopy from 48–72 h after infection. The number of GFP-positive cells in wells treated with different amounts of PsV was counted by fluorescence microscopy, and PsV titre (TU/mL) was determined according to the formula as ([GFP-positive cells] × [Dilution Factor] × 100). Finally, PsV concentration was diluted to 1 × 107 TU/mL and stored at −80℃ for use.
PsV infection
The effect of antibiotics on SARS-CoV-2 cell invasion was evaluated using a PsV invasion assay, by observing the expression level of GFP and detecting luciferase activity after PsV infection for 48 h.
After 2 × 104 HEK-293T-hACE2 cells per well were seeded in a 96-well plate for 24 h, 100 μM DAP or VAN was incubated with 5 × 105 TU/mL PsV on ice for 1 h, and then 150 μL of the mixture was added to HEK-293T-hACE2 cells per well for incubation for 6 h at 37°C. The supernatant was removed and replaced with fresh DMEM medium for 48 h. Finally, from the collected cells, the proportion of GFP-expressing cells and the luciferase activity was determined to evaluate the infection efficiency of PsV on HEK-293T-hACE2.
The GFP-positive cells were measured on one automatic counter equipment (Countstar, Fluorescence cell analyser). Generally, 20 μL of cell solution was added to the well of the counter plate, and the proportion of GFP-positive cells was analysed using the fluorescent cell counting mode of the cell counter. Cell luciferase activity was detected using a commercial kit (One-Lumi™ Firefly Luciferase assay, Cat # RG055S, Beyotime, China) on a microplate reader (BioTek, USA) referring to a previous method [Citation35]. The 96-well plate was taken out from the 37 °C incubator to stand at room temperature for 10 minutes. Then 100 μL luciferase assay reagent was added to each well and incubated at room temperature for 5 min to detect chemiluminescence level on a multifunctional microplate reader, and the chemiluminescence level was expressed as the relative light unit (RLU). The original RLU values obtained from the saline samples were the blank background, which was the baseline of comparison with other experimental groups to be deducted.
Ca2+ binding experiments
After 2 × 104 HEK-293T-hACE2 cells were seeded in one well of a 96-well plate to culture with DMEM medium for 24 h at 37°C, saline was added to gently wash three times, and 200 μL of Ca2+-free DMEM was added to each well. The cells cultured with Ca2+-free DMEM were divided into three groups for assay, including the blank saline group without PsV solution and DAP, PsV group (5 × 105 TU/mL) and DAP treatment group with 5 × 105 TU/mL PsV and 100 μM DAP incubation (PsV + DAP).
Meanwhile, the other two groups of HEK-293T-hACE2 cells were cultured in Ca2+-containing normal DMEM. Under the same conditions, one group was added with 5 × 105 TU/mL PsV, the other group was added with 5 × 105 TU/mL PsV and 100 μM DAP, as a comparison control of Ca2+-free culture. The PsV solution and DAP was respectively added into the corresponding four groups to incubate for 6 h, and then replaced with fresh normal DMEM media for culture another 48 h to determine PsV invasion efficiency by detecting GFP-positive cells and luciferase activity.
Statistical analysis
All data represent at least three independent experiments and are shown as mean ± standard error of the mean (SEM). Statistical analyses were performed using the GraphPad Prism 8 software. A two-tailed unpaired Student’s t test was used to determine the differences between two independent groups, unless otherwise indicated (*, p value of < 0.05; **, p value of < 0.01; ***, p value of < 0.001;****, p value of < 0.0001; ns, p value of > 0.05).
Results
Molecular docking indicates DAP binds to S protein of SARS-CoV-2 and Omicron variant
It is well known that the first step of SARS-CoV-2 infection of host cells is the binding of the S1 subunit of the S protein with the host human ACE2 receptor, which indicates that the SARS-CoV-2 S-protein is an important intervention target to inhibit virus entry into host cells. Considering the potential interactions among viruses, host cells and drugs (antibiotics), we investigated whether antibiotics affect the binding of SARS-CoV-2 S-protein to the host cell ACE2 protein. Three commonly used polypeptide antibiotics, DAP, VAN, and BLE, were chosen for molecular docking with the S-protein of SARS-CoV-2 and several VOC variants.
Molecular docking results showed that DAP and VAN had a certain degree of binding ability to the S-protein of SARS-CoV-2 and several derived variants. However, the binding of BLE with SARS-CoV-2 was so weak that it can be ignored (). Among the interactions of DAP with the virus S-protein, the binding free energy of DAP with the S-protein of the Delta variant was the lowest with −115.742 kcal, indicating that DAP had a stronger binding affinity with the Delta variant. Similarly, the binding free energies of VAN interacting with the S-protein of the Delta and Omicron variants were −143.595 and −114.473 kcal respectively, which indicated that VAN had a stronger binding affinity to the Delta variant (B.1.617.2) than the Omicron variant (B1.1.529).
Table 1. Binding energy from the docking analysis of 3 polypeptide antibiotics with S-protein of SARS-CoV-2 and several virus variants.
From the binding profiling of DAP with SARS-CoV-2 by molecular docking, DAP was located in the cavity near the Cys379 site of S-protein. However, in the docking results of DAP with Omicron (B1.1.529) S protein, DAP was located near the Asn135 of omicron (B1.1.529) S-protein and close to the RBD region of the S1 subunit () VAN was located in the RBD region of the S-protein of SARS-CoV-2 and omicron (B1.1.529) (). In contrast, VAN had a stronger affinity for the Omicron (B1.1.529) S protein. Next, we verified the effects of these two antibiotics on virus-infected cells using PsV invasion assay.
Figure 1. The docking profiling of the binding complex of antibiotics with S-protein of SARS-CoV-2 and Omicron variant (B1.1.529). (a). Binding docking of DAP with the WT S-protein. DAP is located in the cavity near the Cys379 site of S-protein. (b). Docking of DAP binding with Omicron (B1.1.529) S-protein. DAP is located near the Asn135 of Omicron (B1.1.529) S-protein and close to the RBD region of the S1 subunit. (c). Docking of VAN binding with WT S-protein. VAN is located in the RBD region of WT S-protein. (d). Docking of VAN binding with Omicron (B1.1.529) S-protein. VAN is located in the RBD region of Omicron (B1.1.529) S-protein. (e-f). Chemical structure of DAP (e) and VAN (f). The S-protein is a trimeric protein, the blue, red, and green part of the figure represents different monomers respectively. The white arrow represents the antibiotics, and the yellow section represents amino acids on the S-protein at the binding site. DAP: daptomycin, VAN: vancomycin. WT: SARS-CoV-2 original strain.
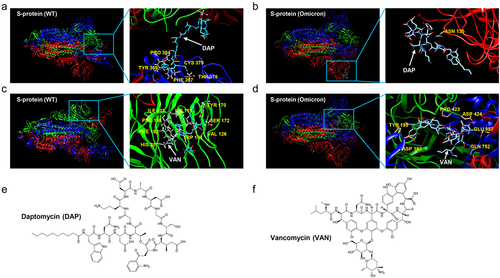
Invasion efficiency of PsV
Because Omicron had become the main epidemic strain in last years, we constructed an Omicron PsV using a retrovirus system to mimic its infection. We constructed the S-protein-containing Omicron PsV, coding Omicron (B1.1.529) S-protein, GFP and luciferase (), to investigate interaction of Omicron/S-protein with antibiotics. The GFP and luciferase are available of two labelling markers due to their sensitivity and simplicity, and they are helpful for easily measuring PsV invasion efficiency in following experiments ( and ).
Figure 2. S-protein-containing PsV production procedure and PsV invasion efficiency on several cell lines. (a). S-protein-containing PsV construction procedure. The gene of GFP was inserted into the plasmid pNL4–3-Luc-R-E to construct a helper packaging plasmid named pNL4–3-GFP-Luc-R-E, which included CMV promoter, GFP and luciferase. The expression plasmid pcDNA3.1-Omicron/S and the packaging plasmid pNL4–3-GFP-Luc-R-E were co-transfected into HEK-293T cells at a ratio of 1:4 to incubate 48h, then the culture medium supernatant was collected to filter by 0.45μm filter membrane, and centrifuged at 72,000×g for 2h at 4℃ to obtain the PsV precipitate. The PsV precipitate was resuspended in fresh medium to determine PsV titers. Finally, 1 × 107 TU/mL PsV was stored at−80℃ for further use. (b). DAP influences on cell viability of Vero-E6, Huh-7 and HEK-293T-hACE2 at different concentrations. (c). VAN influences on cell viability of Vero-E6, Huh-7, and HEK-293T-hACE2 at different concentrations. (d). Invasion efficiency of PsV on Vero-E6, Huh-7 and HEK-293T-hACE2 cells. 6 × 103 cells from each group were collected for luciferase activity assay, and the result was expressed as the relative light unit (RLU). A two-tailed unpaired Student’s t test was used to determine the differences between two independent groups, data were presented as the means±SEM (***, p < 0.001). The experiments were repeated for three times.
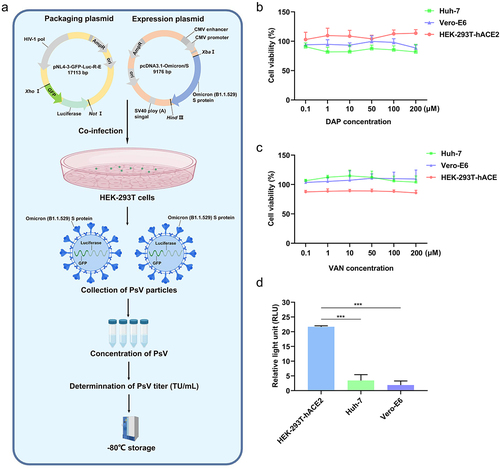
Figure 3. PsV invasion to HEK-293T-hACE2 cells with antibiotics co-operation. (a). Schematic diagram of PsV invasion under antibiotics incubation. After 2 × 104 HEK-293T-hACE2 cells per well were seeded in a 96-well plate to culture 24h, 100μM DAP or VAN was respectively incubated with 5 × 105 TU/mL PsV on ice for 1h, and then 150μL of the mixture was added to HEK-293T-hACE2 cells per well for incubation of 6h at 37°C, the supernatant was removed and replaced with new DMEM medium for culture 48h. Finally, from totally collected cells, the proportion of GFP-tagging cell quantity and the luciferase activity were determined to evaluate infection efficiency of PsV on HEK-293T-hACE2. (b). Fluorescence imaging of the PsV invasion cells. The number of cells expressing GFP protein was directly observed by high magnification microscope (20×) after PsV infection for 48h. (c). The relative percent of GFP-positive host cells in the PsV invasion assay. After 48h of cell culture, the proportion of GFP-expressing cells in each group was counted on the cell counter. 4 × 103 cells were collected and added to the wells of the counter plate, and the proportion of GFP-positive cells was analyzed using the fluorescent cell counting mode of the cell counter. (d). Luciferase activity was measured in the PsV invasion assay. After 48h of cell culture, 1.2 × 105 cells from each group were collected for luciferase activity assay, and the result was expressed as the relative light unit (RLU). (e). Comparison of invasion efficiency of PsV under different incubation concentrations of DAP. 6 × 103 cells from each group were collected for luciferase activity assay to evaluate influence of different concentrations of DAP on the invasion efficiency of PsV, and the result was expressed as the relative light unit (RLU). A two-tailed unpaired Student’s t test was used to determine the differences between two independent groups, data were presented as the means±SEM (***, p < 0.001); ns, p > 0.05). The experiments were repeated for three times.
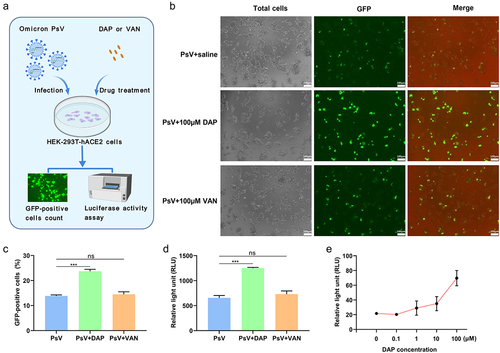
As shown in , the helper packaging plasmid pNL4–3-GFP-Luc-R-E that included the gene encoding GFP and luciferase, was co-infected with the expression plasmid pcDNA3.1-Omicron/S to HEK-293T cells to produce PsV. Once the host HEK-293T-hACE2 cells were infected by PsV, the PsV invasion efficiency was easily checked through GFP-producing fluorescence level of under 488 nm and luciferase activity analysis. Similarly, the simultaneous use of GFP and luciferase in PsV has been widely applied in previous studies [Citation36].
The PsV contained an Omicron spike glycoprotein on the surface of the envelope, and the co-expression of GFP and luciferase was included in the PsV particles (). The expression plasmid encoding the Omicron/S-protein was co-transfected with the packaging plasmid pNL4–3-GFP-Luc-R-E that contained another HIV-1 backbone, GFP and luciferase reporter genes into HEK-293T cells, and the cell medium supernatant was collected after 48 h for filtration and ultracentrifugation to enrich the PsV precipitate. Finally, 1 × 107 TU/mL PsV was resuspended in fresh medium and stored at −80℃ until use. The invasion efficiency of the PsV was determined by detecting the luciferase activity.
Furthermore, we detected the effects of different DAP and VAN concentrations on the viability of Vero-E6, Huh-7, and HEK-293T-hACE2 cells using the CCK-8 reagent. In fact, 0–200 μM DAP and VAN had no significant influence on the viability of Vero-E6, Huh-7, and HEK-293T-hACE2 cells (). To avoid the influence of antibiotic concentration on host cells proliferation, 100 μM DAP or VAN were chosen to measure their effects on PsV invasion.
Luciferase activity assay showed that the PsV significantly infected HEK-293T-hACE2 cells, in contrast, the PsV infected Vero-E6 and Huh-7 cells were less effective (p < 0.001) (). Therefore, we selected HEK-293T-hACE2 cells, which were sensitive to PsV infection, for further analysis of the effect of antibiotics on PsV entry.
DAP promotes invasion of PsV to host cells
The GFP-expressing PsV contained GFP protein and luciferase, and the invasion efficiency of the PsV under DAP exposure was determined by observing the number and fluorescence intensity of green fluorescent cells (). A 150 µL mixture of 5 × 105 TU/mL PsV and 100 μM DAP or VAN was incubated with 2 × 104 HEK-293T-hACE2 cells for 6 h, the supernatant was removed, and 200 µL DMEM medium was added to cells to culture for 48 h. The number of cells expressing GFP protein and the intensity of fluorescence were significantly higher in DAP-incubated HEK-293T-hACE2 cells than in those incubated with VAN and those without antibiotic treatment. According to the percentage of GFP expression, 23.71% of DAP-treated cells were infected by PsV, 14.52% of VAN-incubated cells, and 13.89% of mock cells were infected by PsV (). This indicates that DAP treatment contributed to PsV entry into cells, whereas VAN exposure had almost no influence on PsV invasion.
Similarly, cell luciferase activity data also showed that DAP-exposed cells were more easily infected by PsV than VAN-incubated cells and cells without antibiotic exposure (). The RLU value was 1250 in the DAP-exposed HEK-293T-hACE2 cells by PsV invasion compared with the value of 723.3 and 656.7 for VAN-treated and antibiotics-free PsV infection respectively.
Moreover, the PsV invasion efficiency was improved with an increase in DAP exposure concentration from 0 to 100 μM (). The RLU value was 21.7 in the PsV alone group and up to 69.7 when 100 μM DAP was added. All these results indicate that DAP has a significant promoting effect on PsV entry into human cells, whereas VAN has no obvious influence on this process.
DAP enhances PsV invasion under Ca2+ co-existing
The literature has reported Ca2+ in the cell media is very important for the activation of the S-protein conformation of SARS-CoV-2 [Citation37]. Considering that Ca2+ is pivotal for DAP antibiotic action, we further investigated the influence of Ca2+ on the interaction between DAP and PsV invasion. We compared the efficiency of PsV invasion into cells between Ca2+-free DMEM media and normal Ca2+-containing cellular matrix environments ().
Figure 4. DAP promotes PsV invasion under co-operation with Ca2+. (a). Schematic diagram of PsV invasion under DAP incubation in Ca2+-free or Ca2+-containing medium. 2 ×104 HEK-293T-hACE2 cells were seeded in 96-well plates to culture with normal DMEM medium for 24h at 37°C, and sterile saline was added to gently wash three times, then 200μL of Ca2+-free DMEM or Ca2+-containing DMEM was added to each well, respectively. The cells were divided into five groups: Ca2+-free DMEM+saline group, Ca2+-free DMEM+PsV group (5 × 105 TU/mL), Ca2+-free DMEM+PsV (5 × 105 TU/mL)+DAP (100μM) group, Ca2+-containing DMEM+PsV group (5 × 105 TU/mL), and Ca2+-containing DMEM +PsV (5 × 105 TU/mL)+DAP (100μM) group. The PsV solution and DAP were added according to the grouping as described above, cultured for 6h, and then replaced with fresh medium for culturing another 48h. (b). Luciferase activity was measured to compare the PsV invasion efficiency. 6 × 103 cells from each group were collected for luciferase activity assay to assess the invasion efficiency of the PsV, and the result was expressed as the relative light unit (RLU). (c). Fluorescence imaging of PsV invasion. The number of GFP-expressing cells was directly observed by high magnification microscope (20×) after PsV infection for 48h. A two-tailed unpaired Student’s t test was used to determine the differences between two independent groups, data were presented as the means±SEM (****, p < 0.0001). The experiments were repeated for three times.
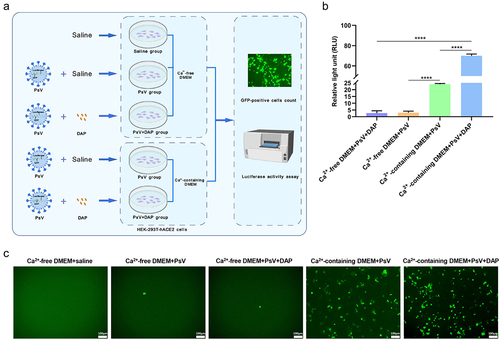
2 × 104 HEK-293T-hACE2 cells were seeded into 96-well plates. After culturing with normal DMEM for 24 h at 37°C, saline was added and gently washed three times, and 200 μL of Ca2+-free DMEM or Ca2+-containing DMEM was added to each well, respectively. The cells were divided into five groups: Ca2+-free DMEM + saline group, Ca2+-free DMEM + PsV group (5 × 105 TU/mL), Ca2+-free DMEM + PsV (5 × 105 TU/mL) + DAP (100 μM) group, Ca2+-containing DMEM + PsV group (5 × 105 TU/mL), and Ca2+-containing DMEM +PsV (5 × 105 TU/mL) + DAP (100 μM) group. The corresponding PsV solution and DAP were added according to the grouping described above, cultured for 6 h, and then changed to fresh medium for another 48 h (). When the PsV was incubated in Ca2+-free medium, only 0.063% of the cells expressed GFP protein, and the RLU value was 3. However, when PsV was incubated in Ca2+-containing medium, 15.4% of the cells expressed GFP protein, and the RLU value was 24, the proportion of GFP positive cells and luciferase activity were significantly increased (p < 0.0001), indicating that Ca2+ was helpful for PsV invasion. At the same time, in the Ca2+-containing medium, the proportion of GFP positive cells and luciferase activity in the PsV + DAP group were also significantly increased compared with the PsV group (p < 0.0001), indicating that DAP promotes the invasion efficiency of pseudovirus ().
In addition, when PsV and DAP were added to the Ca2+-free medium simultaneously, the number of cells expressing GFP protein was 0.067%, and the RLU value was 2.67. However, when PsV with DAP were added to the Ca2+-containing medium, the number of cells expressing GFP protein and luciferase activity were significantly enhanced (p < 0.0001), the proportion of GFP positive cells was 27.41%, and the RLU value was 70. (). These results indicate that DAP does not affect PsV invasion in the absence of Ca2+. In general, cell invasion of PsV requires the participation of Ca2+, and DAP enhances the invasion of PsV under Ca2+ co-existing ().
Figure 5. Schematic summary of PsV invasion to cells under DAP co-operation. Compared to the infection efficiency of PsV alone (the left), the cell number of PsV invasion was increased when DAP was supplemented to Ca2+-containing cell medium (the right). DAP interacts with S-protein of the PsV, then inserts to host cell membrane and promotes invasion of PsV.
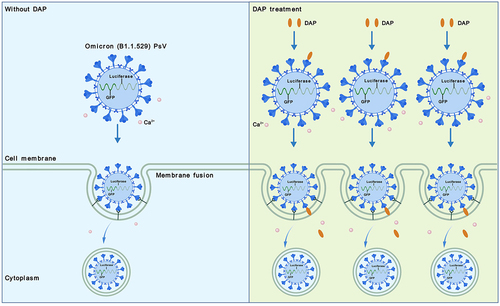
Discussion
DAP, a cyclic lipopeptide antibiotic, is commonly used to treat infections caused by Gram-positive bacteria, including complicated skin and skin structure infections, right-sided infective endocarditis, bacteraemia, meningitis, sepsis and urinary tract infections [Citation22,Citation38]. In July 2010, the U.S. Food and Drug Administration (FDA) issued information alerting patients and medical professionals about the potential risk of eosinophilic pneumonia caused by the use of the intravenous drug DAP during the course of diagnosis and treatment [Citation39]. Eosinophilic pneumonia is a rare and serious disease characterized by fever, cough, dyspnoea, and new infiltrates on imaging [Citation40]. The exact mechanism of DAP causes eosinophilic pneumonitis is unknown, but it has been hypothesized that chronic administration of DAP causes the drug to accumulate near the epithelial alveolar surface leading to epithelial injury and pneumonia [Citation40,Citation41]. In addition, alveolar macrophages detect antigens and activate TH-2 cells, which subsequently release interleukin 5, IL-5 promotes increased production of eosinophils and migration into the lung parenchyma [Citation42]. Therefore, DAP is not recommended for pneumonia treatment.
The use of antibiotics, including DAP, ceftriaxone, carbapenems, and azithromycin, has greatly increased during the new coronavirus outbreak [Citation15,Citation21]. During the spring 2020 pandemic, 83.1% of hospitalized patients received empirical antibiotic treatment [Citation43]. We speculate that there are two reasons for this increase in DAP use. On the one hand, the abuse of antibiotics leads to the emergence of antibiotic resistance [Citation19]. As a broad-spectrum antibiotic, DAP is effective against many drug-resistant bacteria, while its use has increased in patients with resistant strains. Moreover, the increased frequency of serious coinfections and secondary infections in hospitalized and critically ill patients may lead to increased DAP consumption. A study of 254 patients admitted to the ICU due to COVID-19 showed that 35 (13.8%) patients received antibiotics before admission, 228 (89.8%) patients received antibiotics within 48 h after admission, and 241 (94.9%) patients received antibiotics throughout hospital admission. Co-infections occurred in 83 (32.7%) patients, and Staphylococcus aureus was the most common potential co-pathogen within 48 h after admission, including MRSA [Citation43]. DAP has a good inhibitory effect on MRSA, which may be the reason for the increased use of DAP in these patients. Another study showed that bacterial coinfections occur in 6–29% of ICU patients admitted with COVID-19 [Citation44]. Herein, we investigated the possible interaction between DAP and SARS-CoV-2, which provides direct evidence of drug safety and possible side effects of DAP use for SARS-CoV-2 infection.
DAP and VAN both belong to peptide antibiotics, and have broad antibacterial spectrum with strong antibacterial effect, which are widely used in antibacterial infection [Citation22,Citation45]. While DAP and VAN have different antibacterial mechanism. DAP has a specific cyclopeptide structure to confer its highly efficient antimicrobial activity. Studies indicate that DAP can binds to Ca2+ to form a micellar structure in solution and oligomerizes in cell membrane, and this oligomer contributes to delivering DAP to cell membrane of bacteria, which results in the dissipation of cell membrane potential and potassium ion efflux, ultimately leads to cell death [Citation46]. VAN is a glycopeptide antibiotic, which plays a bactericidal role by inhibiting the synthesis of peptidoglycan in the bacterial cell wall [Citation47].
Both DAP and VAN are highly effective against most of bacterial infection, including Staphylococcus aureus, especially MRSA. Staphylococcus aureus is reported to be the most common pathogen causing co-infection and superinfection in patients with COVID-19 [Citation48]. This necessarily leads to increased use of DAP and VAN in the clinic. The difference of structure and antibacterial mechanism of DAP and VAN should be paid attention to evaluate their efficiency and side-effect to treat pneumonia with COVID-19 co-infection or secondary infections.
The wide application of DAP and VAN in patients with COVID-19 complicated with other bacterial infection deserves to further clear about the drug safety and possible side effect. Several studies have shown that most patients of COVID-19 co-infection and secondary infection harbour antibiotic resistance [Citation49]. Although DAP and VAN are effective against a variety of antibiotic-resistant bacteria, including MRSA, yet their availability in patients with COVID-19 co-infection and secondary infections should be confirmed.
The efficacy of DAP treatment for SARS-CoV-2-induced pneumonia is unclear, and several previous studies have different even inconsistent reports on DAP effect on pneumonia treatment. For instance, Renata Abel et al. used an integrated drug repurposing approach to computationally identify potential inhibitors of the main protease of SARS-CoV-2 in databases of the approved and withdrawn drugs, and found DAP to be a candidate to inhibit SARS-CoV-2 [Citation50]. Similarly, another research reported that when the SARS-CoV-2 virus was incubated with Vero cells for 24 h and treated with DAP, DAP showed better antiviral activity with a 50% cytotoxic concentration of 0.74 μg/mL [Citation51]. The two reports are consistent with one virtual screening data of 3000 existing drugs to target the major protease and spike protein of SARS-CoV-2 virus, which believes DAP could effectively bind to the RBD region of S-protein of SARS-CoV-2 to inhibit virus [Citation52].
However, several other studies have indicated that DAP does not reduce the infectivity of two coronaviruses, including human coronavirus 229E (HCoV-229E) and MERS coronavirus (MERS-CoV) [Citation53]. In addition, Chowdhury T et al. reported that DAP had a poor binding ability to SARS-CoV-2 S-protein through silico docking [Citation54]. In addition, DAP has been confirmed not to be suitable for the treatment of bacterial infection relative pneumonia because it is inactivated by pulmonary surfactant [Citation22,Citation55]. So far, we focus on the feasibility of DAP usage in SARS-CoV-2 infection by in silico docking and subsequent validation of PsV invasion to cells.
Molecular docking, a powerful computational tool, has been widely used to predict interactions between proteins and drugs. Therefore, we selected three common peptide antibiotics, DAP, VAN, and BLE, to dock with the spike protein of SARS-CoV-2 and its variants. BLE is a glycopeptide antibiotic with strong antitumor activity against a range of lymphomas, head and neck cancers and germ cell tumours [Citation56], which is selected to a negative control drug in molecular docking of DAP, VAN with the S-protein of SARS-CoV-2.
Both DAP and VAN exhibited certain binding abilities to SARS-CoV-2 and its variant strains, whereas BLE showed poor binding ability. Considering the increased use of antibiotics in hospitals during the COVID-19 pandemic, it is still unknown whether the binding of antibiotics to SARS-CoV-2 affects the safety of drug treatment for COVID-19 patients. Therefore, we simulated viral infection in vitro using a PsV model with an Omicron variant and treated it with antibiotics to investigate whether DAP binding to the spike protein would affect virus invasion. The results showed that PsV invasion increased after DAP treatment, whereas no such phenomenon was observed in the VAN-treated group. Therefore, we investigated possible reasons why DAP promoted PsV invasion.
It is well known that the antibacterial effect of DAP is related to Ca2+ existence, DAP inserts into bacterial cell membranes in a Ca2+-dependent manner, leading to membrane depolarization and subsequent loss of intracellular components including K+, Mg2+, and ATP [Citation57]. Interestingly, Ca2+ is also important for viral infection in cells. Ca2+ is released from the lysosomal cavity and out of the cell via calcium pumps to mediate membrane fusion, and Ca2+ interactions with fusion peptides cause spatial changes in the S-protein, ultimately promoting the entry of SARS-CoV-2 [Citation58]. Studies have shown that the membrane fusion process of SARS-CoV-2 is dependent on Ca2+ concentration, and the variant S-protein of SARS-CoV-2 may increase membrane fusion by increasing Ca2+ sensing ability and ultimately facilitating viral entry [Citation37].
To investigate whether the promotion of PsV entry into cells by DAP is related to Ca2+, we performed virus invasion experiments using a Ca2+-free medium. We found that the number of PsV-entering cells was significantly reduced after using Ca2+-free medium, indicating that the lack of Ca2+ attenuated viral invasion, which is consistent with previous reports [Citation37]. After DAP treatment using Ca2+-free medium, we found no significant difference in the number of PsV-entering cells compared with the blank control group, which indicated that the effect of DAP in promoting PsV entry disappeared in the absence of Ca2+. When DAP exerts its antibacterial effect, it binds Ca2+ at a ratio of 1:1 to form a positively charged aggregate, which approaches the surface of the cell membrane due to electronegativity and then spreads out and inserts into the cell membrane to function. This process simultaneously enriches DAP and Ca2+ on the surface of the cell membrane; therefore, DAP may enrich Ca2+ near the PsV to promote the efficiency of PsV invasion (). However, its specific mechanism remains to be studied.
In summary, our study shows that DAP can promote the entry of the PsV into cells, and this effect may be related to Ca2+ concentration. In contrast, VAN had no significant influence on PsV invasion. With the continuous recombination and sequence reassortment of SARS-CoV-2, the new Omicron subvariant, XBB, has become the main epidemic strain, and studies have shown that XBB has stronger infectivity [Citation14]. Although various guidelines have clearly recommended reducing the use of antibiotics in patients with SARS-CoV-2, the use of antibiotics is still inevitable when patients have bacterial co-infection. Our results provide a use reference for clinicians to choose VAN or other antibiotics and minimize DAP use when mixed bacterial infections occur in hospitalized or critically ill patients caused by SARS-CoV-2, especially those caused by multidrug-resistant bacteria.
Author contributions
Xu Cao: Conceptualization, Methodology, Investigation, Writing Original Draft. Lan Huang: Investigation and Writing-Review. Min Tang: Methodology, Visualization. Yue Liang and Huijin Hou: Validation. Xinpeng Liu: Investigation, Visualization. Shufang Liang: Conceptualization, Writing-review and editing, funding acquisition.
Abbreviations
DAP: daptomycin; VAN: vancomycin; BLE: bleomycin; PsV: pseudovirus; Ca2+: calcium ions; GFP: green fluorescent protein; SARS-CoV-2: severe acute respiratory syndrome coronavirus 2; ACE2: angiotensin-converting enzyme 2.
Acknowledgements
We thank Dr. Tong of the State Key Laboratory of Biotherapy, Sichuan University, for kindly providing the HEK-293T-hACE2 cell line. And we also thank the free online graphic design tool (gdp.renlab.cn) to provide for creating the schematic figures in the paper.
Disclosure statement
No potential conflict of interest was reported by the author(s).
Data Availability statement
The data presented in this study are available on request from the corresponding author.
Additional information
Funding
References
- Majumder J, Minko T. Recent developments on therapeutic and diagnostic approaches for COVID-19. Aaps J. 2021;23(1):14. doi: 10.1208/s12248-020-00532-2
- Zhu N, Zhang D, Wang W, et al. A novel coronavirus from patients with pneumonia in China, 2019. N Engl J Med. 2020;382(8):727–14. doi: 10.1056/NEJMoa2001017
- Atzrodt CL, Maknojia I, McCarthy RDP, et al. A guide to COVID-19: a global pandemic caused by the novel coronavirus SARS-CoV-2. FEBS J. 2020;287(17):3633–3650. doi: 10.1111/febs.15375
- Cai Y, Zhang J, Xiao T, et al. Distinct conformational states of SARS-CoV-2 spike protein. Science. 2020;369(6511):1586–1592. doi: 10.1126/science.abd4251
- Poltronieri P, Sun B, Mallardo M. RNA Viruses: RNA roles in pathogenesis, coreplication and viral load. Curr Genomics. 2015;16(5):327–335. doi: 10.2174/1389202916666150707160613
- Wang MY, Zhao R, Gao LJ, et al. SARS-CoV-2: structure, biology, and structure-based therapeutics development. Front Cell Infect Microbiol. 2020;10:587269. doi: 10.3389/fcimb.2020.587269
- Djomkam ALZ, Olwal CO, Sala TB, et al. Commentary: SARS-CoV-2 cell entry depends on ACE2 and TMPRSS2 and is blocked by a clinically proven protease inhibitor. Front Oncol. 2020;10:1448. doi: 10.3389/fonc.2020.01448
- Hoffmann M, Kleine-Weber H, Pöhlmann S. A multibasic cleavage site in the spike protein of SARS-CoV-2 is essential for infection of human lung cells. Mol Cell. 2020;78(4):779–784.e5. doi: 10.1016/j.molcel.2020.04.022
- Xia X. Domains and functions of spike protein in SARS-CoV-2 in the context of vaccine design. Viruses. 2021;13(1):109. doi: 10.3390/v13010109
- Lippi G, Adeli K, Plebani M. Commercial immunoassays for detection of anti-SARS-CoV-2 spike and RBD antibodies: urgent call for validation against new and highly mutated variants. Clin Chem Lab Med. 2021;60(3):338–342. doi: 10.1515/cclm-2021-1287
- Ingraham NE, Ingbar DH. The omicron variant of SARS-CoV-2: understanding the known and living with unknowns. Clin Transl Med. 2021;11(12):e685. doi: 10.1002/ctm2.685
- Callaway E. Heavily mutated omicron variant puts scientists on alert. Nature. 2021;600(7887):21. doi: 10.1038/d41586-021-03552-w
- Xia S, Wang L, Jiao F, et al. SARS-CoV-2 omicron subvariants exhibit distinct fusogenicity, but similar sensitivity, to pan-CoV fusion inhibitors. Emerg Microbes Infect. 2023;12(1):2178241. doi: 10.1080/22221751.2023.2178241
- Scarpa F, Azzena I, Locci C, et al. Molecular in-depth on the epidemiological expansion of SARS-CoV-2 XBB.1.5. Microorganisms. 2023;11(4):912. doi: 10.3390/microorganisms11040912
- Castro-Lopes A, Correia S, Leal C, et al. Increase of antimicrobial consumption in a tertiary care hospital during the first phase of the COVID-19 pandemic. Antibiotics. 2021;10(7):778. doi: 10.3390/antibiotics10070778
- Abu-Rub LI, Abdelrahman HA, Johar ARA, et al. Antibiotics prescribing in intensive care settings during the COVID-19 era: a systematic review. Antibiotics. 2021;10(8):935. doi: 10.3390/antibiotics10080935
- Lai CC, Chen SY, Ko WC, et al. Increased antimicrobial resistance during the COVID-19 pandemic. Int J Antimicrob Agents. 2021;57(4):106324. doi: 10.1016/j.ijantimicag.2021.106324
- Chedid M, Waked R, Haddad E, et al. Antibiotics in treatment of COVID-19 complications: a review of frequency, indications, and efficacy. J Infect Public Health. 2021;14(5):570–576. doi: 10.1016/j.jiph.2021.02.001
- Li J, Wang J, Yang Y, et al. Etiology and antimicrobial resistance of secondary bacterial infections in patients hospitalized with COVID-19 in Wuhan, China: a retrospective analysis. Antimicrob Resist Infect Control. 2020;9(1):153. doi: 10.1186/s13756-020-00819-1
- Langford BJ, So M, Raybardhan S, et al. Antibiotic prescribing in patients with COVID-19: rapid review and meta-analysis. Clin Microbiol Infect. 2021;27(4):520–531. doi: 10.1016/j.cmi.2020.12.018
- Grau S, Echeverria-Esnal D, Gómez-Zorrilla S, et al. Evolution of antimicrobial consumption during the first wave of COVID-19 pandemic. Antibiotics. 2021;10(2):132. doi: 10.3390/antibiotics10020132
- Heidary M, Khosravi AD, Khoshnood S, et al. Daptomycin. J Antimicrob Chemother. 2018;73(1):1–11. doi: 10.1093/jac/dkx349
- Fowler Jr VG, Boucher HW, Corey GR, et al. Daptomycin versus standard therapy for bacteremia and endocarditis caused by Staphylococcus aureus. N Engl J Med. 2006;355(7):653–665. doi: 10.1056/NEJMoa053783
- Bradley J, Glasser C, Patino H, et al. Daptomycin for complicated skin infections: a randomized trial. Pediatrics. 2017;139(3):e20162477. doi: 10.1542/peds.2016-2477
- Holland TL, Cosgrove SE, Doernberg SB, et al. Ceftobiprole for treatment of complicated staphylococcus aureus bacteremia. N Engl J Med. 2023;389(15):1390–1401. doi: 10.1056/NEJMoa2300220
- Raja A, LaBonte J, Lebbos J, et al. Daptomycin. Nat Rev Drug Discov. 2003;2(12):943–944. doi: 10.1038/nrd1258
- King A, Phillips I. The in vitro activity of daptomycin against 514 Gram-positive aerobic clinical isolates. J Antimicrob Chemother. 2001;48(2):219–223. doi: 10.1093/jac/48.2.219
- Saravolatz LD, Pawlak J, Johnson LB. In vitro activity of oritavancin against community-associated methicillin-resistant Staphylococcus aureus (CA-MRSA), vancomycin-intermediate S. aureus (VISA), vancomycin-resistant S. aureus (VRSA) and daptomycin-non-susceptible S. aureus (DNSSA). Int J Antimicrob Agents. 2010;36(1):69–72. doi: 10.1016/j.ijantimicag.2010.02.023
- Silverman JA, Mortin LI, Vanpraagh ADG, et al. Inhibition of daptomycin by pulmonary surfactant: in vitro modeling and clinical impact. J Infect Dis. 2005;191(12):2149–2152. doi: 10.1086/430352
- Zhao L, Zhong K, Zhao J, et al. SARS-CoV-2 spike protein harnesses SNX27-mediated endocytic recycling pathway. MedComm. 2021;2(4):798–809. doi: 10.1002/mco2.92
- Weng G, Gao J, Wang Z, et al. Comprehensive evaluation of fourteen docking programs on protein-peptide complexes. J Chem Theory Comput. 2020;16(6):3959–3969. doi: 10.1021/acs.jctc.9b01208
- Chen B, Deng YN, Wang X, et al. miR-26a enhances colorectal cancer cell growth by targeting RREB1 deacetylation to activate AKT-mediated glycolysis. Cancer Lett. 2021;521:1–13. doi: 10.1016/j.canlet.2021.08.017
- Yang R, Huang B, A R, et al. Development and effectiveness of pseudotyped SARS-CoV-2 system as determined by neutralizing efficiency and entry inhibition test in vitro. Biosaf Health. 2020;2(4):226–231. doi: 10.1016/j.bsheal.2020.08.004
- Capcha JMC, Lambert G, Dykxhoorn DM, et al. Generation of SARS-CoV-2 spike pseudotyped virus for viral entry and neutralization assays: a 1-week protocol. Front Cardiovasc Med. 2021;7:618651. doi: 10.3389/fcvm.2020.618651
- Chen J, Xu W, Li L, et al. Immunogenicity and protective potential of chimeric virus-like particles containing SARS-CoV-2 spike and H5N1 matrix 1 proteins. Front Cell Infect Microbiol. 2022;12:967493. doi: 10.3389/fcimb.2022.967493
- Zettl F, Meister TL, Vollmer T, et al. Rapid quantification of SARS-CoV-2-neutralizing antibodies using propagation-defective vesicular stomatitis virus pseudotypes. Vaccines (Basel). 2020;8(3):386. doi: 10.3390/vaccines8030386
- Singh P, Mukherji S, Basak S, et al. Dynamic Ca2+ sensitivity stimulates the evolved SARS-CoV-2 spike strain-mediated membrane fusion for enhanced entry. Cell Rep. 2022;39(3):110694. doi: 10.1016/j.celrep.2022.110694
- Ye Y, Xia Z, Zhang D, et al. Multifunctional pharmaceutical effects of the antibiotic daptomycin. Biomed Res Int. 2019;2019:8609218. doi: 10.1155/2019/8609218
- Kim PW, Sorbello AF, Wassel RT, et al. Eosinophilic pneumonia in patients treated with daptomycin: review of the literature and US FDA adverse event reporting system reports. Drug Saf. 2012;35(6):447–457. doi: 10.2165/11597460-000000000-00000
- Portalatin GM, Chin JA, Foster B, et al. Daptomycin-induced acute eosinophilic pneumonia. Cureus. 2021;13(2):e13509. doi: 10.7759/cureus.13509
- Uppal P, LaPlante KL, Gaitanis MM, et al. Daptomycin-induced eosinophilic pneumonia - a systematic review. Antimicrob Resist Infect Control. 2016;5(1):55. doi: 10.1186/s13756-016-0158-8
- Watts A, Gahona CCT, Raj K. Multifocal pneumonia amidst the global COVID-19 pandemic: a case of daptomycin-induced eosinophilic pneumonia. Cureus. 2021;13(6):e16002. doi: 10.7759/cureus.16002
- Baskaran V, Lawrence H, Lansbury LE, et al. Co-infection in critically ill patients with COVID-19: an observational cohort study from England. J Med Microbiol. 2021;70(4):001350. doi: 10.1099/jmm.0.001350
- Westblade LF, Simon MS, Satlin MJ. Bacterial coinfections in coronavirus disease 2019. Trends Microbiol. 2021;29(10):930–941. doi: 10.1016/j.tim.2021.03.018
- Stogios PJ, Savchenko A. Molecular mechanisms of vancomycin resistance. Protein Sci. 2020;29(3):654–669. doi: 10.1002/pro.3819
- Ma W, Zhang D, Li G, et al. Antibacterial mechanism of daptomycin antibiotic against Staphylococcus aureus based on a quantitative bacterial proteome analysis. J Proteomics. 2017;150:242–251. doi: 10.1016/j.jprot.2016.09.014
- Howden BP, Davies JK, Johnson PDR, et al. Reduced vancomycin susceptibility in Staphylococcus aureus, including vancomycin-intermediate and heterogeneous vancomycin-intermediate strains: resistance mechanisms, laboratory detection, and clinical implications. Clin Microbiol Rev. 2010;23(1):99–139. doi: 10.1128/CMR.00042-09
- Tabah A, Laupland KB. Update on staphylococcus aureus bacteraemia. Curr Opin Crit Care. 2022;28(5):495–504. doi: 10.1097/MCC.0000000000000974
- Langford BJ, So M, Simeonova M, et al. Antimicrobial resistance in patients with COVID-19: a systematic review and meta-analysis. Lancet Microbe. 2023;4(3):e179–e191. doi: 10.1016/S2666-5247(22)00355-X
- Abel R, Ramos MP, Chen Q, et al. Computational prediction of potential inhibitors of the main protease of SARS-CoV-2. Front Chem. 2020;8:590263. doi: 10.3389/fchem.2020.590263
- Shekunov EV, Zlodeeva PD, Efimova SS, et al. Cyclic lipopeptides as membrane fusion inhibitors against SARS-CoV-2: new tricks for old dogs. Antiviral Res. 2023;212:105575. doi: 10.1016/j.antiviral.2023.105575
- Maffucci I, Contini A. In silico drug repurposing for SARS-CoV-2 main proteinase and spike proteins. J Proteome Res. 2020;19(11):4637–4648. doi: 10.1021/acs.jproteome.0c00383
- Johnson BA, Hage A, Kalveram B, et al. Peptidoglycan-associated cyclic lipopeptide disrupts viral infectivity. J Virol. 2019;93(22):e01282–19. doi: 10.1128/JVI.01282-19
- Chowdhury T, Baindara P, Mandal SM. LPD-12: a promising lipopeptide to control COVID-19. Int J Antimicrob Agents. 2021;57(1):106218. doi: 10.1016/j.ijantimicag.2020.106218
- Kanafani ZA, Corey GR. Daptomycin: a rapidly bactericidal lipopeptide for the treatment of Gram-positive infections. Expert Rev Anti Infect Ther. 2007;5(2):177–184. doi: 10.1586/14787210.5.2.177
- Ayilya BL, Balde A, Ramya M, et al. Insights on the mechanism of bleomycin to induce lung injury and associated in vivo models: a review. Int Immunopharmacol. 2023;121:110493. doi: 10.1016/j.intimp.2023.110493
- Ho SW, Jung D, Calhoun JR, et al. Effect of divalent cations on the structure of the antibiotic daptomycin. Eur Biophys J. 2008;37(4):421–433. doi: 10.1007/s00249-007-0227-2
- Liu W, Li H. COVID-19: the CaMKII-like system of S protein drives membrane fusion and induces syncytial multinucleated giant cells. Immunol Res. 2021;69(6):496–519. doi: 10.1007/s12026-021-09224-1