ABSTRACT
Nicotinamide Adenine Dinucleotide (NAD) is an endogenous substance in redox reactions and regulates various functions in metabolism. NAD and its precursors are known for their anti-ageing and anti-obesity properties and are mainly active in the liver and muscle. Boosting NAD+ through supplementation with the precursors, such as nicotinamide mononucleotide (NMN) or nicotinamide riboside (NR), enhances insulin sensitivity and circadian rhythm in the liver, and improves mitochondrial function in the muscle. Recent evidence has revealed that the adipose tissue could be another direct target of NAD supplementation by attenuating inflammation and fat accumulation. Moreover, murine studies with genetically modified models demonstrated that nicotinamide phosphoribosyltransferase (NAMPT), a NAD regulatory enzyme that synthesizes NMN, played a critical role in lipogenesis and lipolysis in an adipocyte-specific manner. The tissue-specific effects of NAD+ metabolic pathways indicate a potential of the NAD precursors to control metabolic stress particularly via focusing on adipose tissue. Therefore, this narrative review raises an importance of NAD metabolism in white adipose tissue (WAT) through a variety of studies using different mouse models.
1. Introduction
NAD+, the oxidized form of nicotinamide adenine dinucleotide (NAD), is a well-known coenzyme that possesses anti-ageing properties [Citation1]. Decreasing NAD+ levels induces ageing-like effects, including cell senescence, DNA damage, defective mitochondrial function, and abnormal epigenetic pattern [Citation1]. Cluster of differentiation 38 (CD38), an enzyme that breaks down NAD+, is a key regulator of NAD+ metabolism [Citation2] and has been known to increase with ageing [Citation3]. Additionally, other enzymes that use NAD as a substrate have also been reported to possess anti-ageing properties. Particularly, sirtuin 1 (SIRT1) is a NAD+-dependent deacetylase that produces nicotinamide (NAM) and acetyl group, altering activity of several transcription factors to regulate biological functions [Citation4]. It protects against DNA damage during ageing [Citation5], and SIRT1 overexpression has been shown to prevent age-induced transcriptional changes [Citation6]. Moreover, previous studies have demonstrated that calorie restriction (CR) NAD+-dependent regulates life span through SIRTs/Sir2 [Citation7,Citation8], which supports the hypothesis that boosting NAD+ levels would induce anti-ageing effects.
NAD+ plays a crucial role as a cofactor and therefore early studies reported the existence of a ‘conferment’ agent that was critical for the alcoholic fermentation of sugar [Citation9]. Later studies revealed that NAD+ is metabolically reactive, thus establishing a crucial basis for future research on NAD metabolism [Citation10]. This was followed by the discovery of nicotinic acid (NA), a NAD precursor that prevents pellagra, a disease caused by vitamin B3 (i.e., niacin) deficiency [Citation11]. Since these early findings, NAD+ has been reported to be not only a vital redox cofactor but also an important signalling molecule that regulates inflammation, mitochondrial function, and circadian rhythm [Citation12]. For example, NAD+ compartmentalized in adipocytes regulates adipocyte differentiation and gene expression, in addition to controlling glucose metabolism [Citation13].
Recent studies have demonstrated that boosting NAD+ and its precursors such as nicotinamide mononucleotide (NMN), NAM, or nicotinamide riboside (NR) prevented age-induced metabolic stress [Citation14–17]. Aging impairs the NAD+ salvage pathway in the liver, which is associated with liver dysfunction [Citation18]. In fat tissue, visfatin/NAMPT (nicotinamide phosphoribosyltransferase), an adipocytokine involved in the first limiting step in the NAD+ salvage pathway, was reported to induce similar effects to those of insulin [Citation19], thus preventing metabolic disorders such as insulin resistance and inflammation in adipocytes [Citation20–23]. However, NAD metabolism in adipose tissue has remained relatively less explored and several conflicting results have been reported. Therefore, this narrative review discusses the adipocyte-specific functions of NAD+ precursors and NAMPT, as well as the discrepancies among their reported functions.
2. Main pathways of NAD+ biosynthesis in mammals
There are several NAD+ synthesis pathways, and two of the most representative biosynthesis and consumption pathways are illustrated in . One of the NAD+ synthesis pathways is the de novo biosynthesis from tryptophan. When tryptophan from food enters the body, it is converted to α-amino-β-carboxymuconate-ε-semialdehyde (ACMS), which is then converted to NAM in the liver and transported to other tissues [Citation24]. This tryptophan catabolism process is also commonly referred to as the kynurenine pathway. In the absence of niacin supplementation, dietary tryptophan can thus be converted to NA [Citation25] and alterations in this tryptophan-nicotinamide pathway can lead to adverse physiological changes (i.e., diseases) [Citation24], suggesting that NAD+ de novo biosynthesis supplies a portion of NAD+ to compensate for ageing-related NAD+ decline.
Figure 1. Main pathways of NAD+ synthesis and consumption in mammals. NAD+ is either synthesized from tryptophan (de novo biosynthesis) or from NMN by NMNAT. NMN can be provided by NR and NAM. The synthesized NAD+ is broken down by NAD+-dependent enzymes such as SIRT1, PARP1, and CD38, and therefore the recycled NAM can be re-synthesized to NAD+ via the NAD+ salvage pathway. NAD±consuming enzymes have several functions. For example, SIRT1 controls circadian rhythm, whereas PARP1 and CD38 regulate DNA repair and mitochondrial function, respectively. Trp, tryptophan; NAD+, a reduced form of nicotinamide adenine dinucleotide; NMNAT, nicotinamide mononucleotide adenylyltransferase; NMN, nicotinamide mononucleotide; NR, nicotinamide riboside; NRK, nicotinamide riboside kinase; NAMPT, nicotinamide phosphoribosyltransferase; NAM, nicotinamide; SIRT1, sirtuin 1; PARP1, poly (ADP-ribose) polymerase 1; CD38, cluster of differentiation 38.
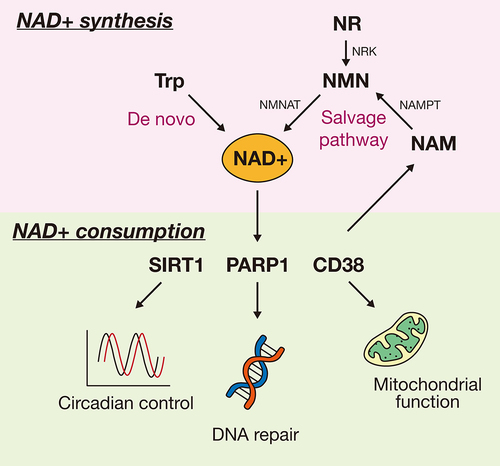
The other pathway is the NAD+ salvage pathway, which consists of the recycling of NAM through enzymes that consume NAD+, such as SIRTs, poly (ADP-ribose) polymerase (PARP), and CD38. In this pathway, NAMPT catalyzes NAM into NMN. When NAD+ precursors such as NR or NMN enter the cell, NR is converted to NMN with ATP, after which NAD+ is synthesized from NMN through the activity of nicotinamide mononucleotide adenylyltransferase (NMNAT). NAD+-dependent enzymes including SIRT1 and PARP1 have been known to possess health-promoting effects. For example, SIRT1 regulates circadian rhythm by recruiting the circadian complex [Citation26] and improves life span by stimulating cell survival in mammalian cells [Citation27]. Additionally, SIRT1 reportedly prevents obesity and age-induced physiological disorders such as cellular senescence and inflammation [Citation4]. Other NAD+-dependents enzymes have different functions. For instance, PARP1 mediates DNA repair and chromatin remodelling in response to DNA damage [Citation28], whereas CD38 is involved in the degradation of NAD precursors generated due to age-related mitochondrial dysfunction via SIRT3 [Citation3]. Several enzymes that use NAD+ as a substrate and are known to prevent metabolic decline have also been associated with cell survival. Therefore, the evidence suggests that the NAD+ salvage pathway is essential to sustain cellular activity. When both NAD+ synthesis pathways function properly, most NAD+ is exclusively generated via the salvage pathway rather than the de novo synthesis pathway [Citation29], and many studies on the importance of NAMPT have been recently conducted.
3. Metabolic effects of boosting NAD+ levels in liver and muscle tissues
The liver is an organ that controls the balance of uptake and storage of glucose by gluconeogenesis and glycogenesis to maintain blood glucose level in the body and plays a crucial role in detoxifying toxins. In mammalian models of high-fat diet (HFD)-induced diabetes, decreased NAD+ levels and expression of NAMPT in the liver can be reversed via NMN supplementation [Citation14]. illustrates the effects of boosting NAD+ levels through the salvage pathway in the liver, skeletal muscle, and white adipose tissue (WAT). NAD+ in the liver restores hepatic gene expression changes caused by ageing through 6-month NMN supplementation [Citation15] and improves metabolic dysfunction such as hepatic insulin resistance in obesity models even after 1-week administration [Citation14]. 4-month supplementation of NR also enhances the remodelling of the hepatic circadian transcriptome via the binding of chromatin to basic helix-loop-helix ARNT like 1 (BMAL1) [Citation30], which is one of the main transcription factors that regulate circadian rhythm. Additionally, enhancing NAD+ level recovers abnormal rhythmic transcriptome patterns in the liver in a SIRT1-dependent manner, thus regulating the acetylation of period circadian regulator 2 (PER2) [Citation30], which is associated with circadian rhythm negative feedback. These observations highlight the importance of the NAMPT/NAD/SIRT1 axis as a therapeutic target for chronic diseases caused by obesity and ageing [Citation31]. Alcohol-induced hepatic steatosis, which is caused by an excessive intake of alcohol and also contributes to obesity, can be treated by decreasing triglyceride accumulation in a SIRT1-dependent manner [Citation32]. Additionally, previous studies have demonstrated that the anti-obesity and anti-ageing effects of NAD+ in the maternal liver are transgenerational and can thus be passed down to the offspring through epigenetic mechanisms [Citation33,Citation34].
Figure 2. Effects of boosting NAD+ through the NAD+ salvage pathway by SIRT1 in the liver, skeletal muscle, and white adipose tissue. NMN is synthesized from NAM and NR by NAMPT and NRK, respectively. The synthesized NAD+ from NMN is used as a SIRT1 substrate, which leads to the recycling of NAD+ via the salvage pathway. In this process, NAD+ can exert different effects depending on the tissue. NAMPT, nicotinamide phosphoribosyltransferase; NAM, nicotinamide; NMN, nicotinamide mononucleotide; NR, nicotinamide riboside; NRK, nicotinamide riboside kinase; NMNAT, nicotinamide mononucleotide adenylyltransferase; NAD+, reduced form of nicotinamide adenine dinucleotide; SIRT1, sirtuin 1.
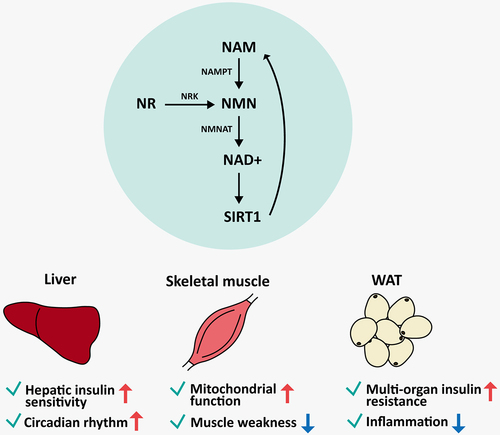
Skeletal muscle makes up most of the total muscle mass in the body and accounts for approximately 40% of the total body mass [Citation35]. Moreover, muscle excitation and contraction are responsible for all body movements. One of the most common ageing-related muscle disorders is sarcopenia, a condition characterized by a decline in muscle volume, strength, and function [Citation35]. Ageing causes the NAD+ levels in the muscles to decrease, thus affecting other metabolic functions. Similarly, obesity diminishes muscle mass and power, thus impairing exercise capacity and lipid oxidation [Citation36]. In humans, supplementation with NAD+ precursors enhances NAD+ metabolism in the muscle [Citation17] and improves muscular insulin signalling [Citation16], albeit without significant changes in muscle metabolism [Citation17]. However, another study demonstrated that the physiological alterations caused by ageing and HFD-induced metabolic stress could be alleviated via NAD+ supplementation in a mouse model [Citation15,Citation37]. Additionally, muscle-specific Nampt knockout mice exhibited mitochondrial dysfunction and muscle weakness that is reversible with NR supplementation [Citation38]. Therefore, the differences in the effects of NAD+ in muscle and liver tissues suggest that the mechanisms of NAD+ metabolism vary in a tissue-specific manner.
In addition to the liver and muscle, other tissues have also been reported to benefit from NAD+ supplementation. In the brain, NMN treatment improves neurological function and protects against brain damage including forebrain ischaemia and hippocampus injury [Citation39]. In the heart, boosting NAD+ prevents NAD+ depletion during ischaemia and protects against reperfusion injury through SIRT1-mediated forkhead box protein O1 (FOXO1) deacetylation [Citation40]. Moreover, enhancing NAD+ levels can promote reproductive health in a sex-dependent manner. In females, both NMN and NR treatment restores ovary quality [Citation41,Citation42] thus improving fertility indicators such as litter size, live birth, and ovulation rate. In contrast, increased NAD+ levels in the testis have been linked to reductions in sperm count and vitality and increased sperm oxidative DNA damage [Citation43]. However, given that these results varied depending on the method of NAD+ supplementation [Citation43], no clear relationship has been identified between male fertility and NAD+. Moreover, NAD+ boosters also possess renoprotective effects, anti-inflammatory effects in lymphoid tissue, and increase insulin sensitivity in the pancreas [Citation12]. Most studies in WAT have focused on genetic models rather than investigating systemic effects of NAD+, and therefore these effects and current research discrepancies will be discussed in the next section.
4. Metabolic effects of boosting NAD+ in white adipose tissue
Adipose tissue stores fat to produce energy but is also an endocrine organ that receives signals and secretes endocrine factors to regulate many important metabolic functions [Citation44]. Adipose tissue mainly consists of brown and white adipose tissue (BAT and WAT), which have different functions and structures. BAT consists of multilocular fat cells that contain a large number of mitochondria. Additionally, uncoupling protein 1 (UCP1) is mostly expressed in BAT and is involved in the release of energy as a heat [Citation45]. In contrast, WAT cells contain a single lipid droplet and few mitochondria [Citation45] and mainly function as a fuel storage [Citation46]. WAT also regulates immune response, inflammation, and glucose metabolism via the endocrine system. Particularly, WAT controls leptin, which in turn modulates many other functions including appetite and energy expenditure [Citation46]. Among the many proteins secreted by WAT, visfatin is an adipocytokine that is known to improve insulin resistance and activates insulin receptors, thereby exerting anti-diabetic effects [Citation19]. Interestingly, visfatin is in fact extracellular NAMPT originating from WAT, suggesting that the NAD regulator in WAT is a promising therapeutic target for the treatment of metabolic disorders.
Studies on NAD+ precursor supplementation have mainly focussed on its effects in the liver [Citation14,Citation15,Citation30,Citation33,Citation34,Citation47] and the muscle [Citation15–17,Citation37], whereas very few studies have explored its role in WAT. However, recent studies have provided novel and promising insights into the effects of boosting NAD+ on WAT (), as well as the adipose-specific effects of NAMPT (). NMN and NR supplementation has been shown to reduce body weight and enhance insulin sensitivity in aged chow-fed wild-type mice and die-induced obese mice, respectively [Citation15,Citation47]. NAM supplementation reduced fat accumulation in diet-induced obese mice [Citation48]. Additionally, both NMN and NR supplementation prevented inflammation even with different treatment durations [Citation15,Citation47]. NAM administration increased mitochondrial biogenesis and glutathione synthesis in WAT [Citation48]. Similarly, another study reported that NMN treatment in HFD-induced type 2 diabetes mouse model promoted the recovery of glutathione S-transferase alpha 2 (Gsta2) gene expression in the liver [Citation14]. Given that various effects were observed depending on the types of tissue, additional research is needed to elucidate the molecular mechanisms underlying the health-promoting effects of NAD+, as well as their tissue-specific variations.
Table 1. Effects of NAD+ precursors in white adipose tissue1.
Table 2. Evidence of NAMPT function based on NAMPT genetically modified mouse models.
Tissue-specific functions of NAMPT using genetically modified mouse models have been investigated (). Due to the importance of NAMPT in the NAD+ salvage pathway, several studies have investigated the functions of NAMPT in a variety of tissues such as the liver, muscle, and fat [Citation20–23,Citation38,Citation49–52] using genetically modified mouse models with ubiquitous and tissue-specific deletion. Dall and colleagues reported that the liver-specific Nampt knockout model exhibited only minor metabolic changes despite a 50% decrease in liver NAD+ levels [Citation50]. In contrast, Nampt muscle-specific knockout mice had increased body weight with reduced muscle mass and impaired muscle function such as myonecrosis [Citation38]. Ubiquitous homozygous knockout was lethal in mice [Citation49], suggesting that NAMPT plays a vital role in systemic functions and may even participate in early development. Another mouse model with ubiquitous deletion generated by mating Nampt exon 2-floxed mice and inducible ubiquitin C-Cre mice resulted in death within 5–10 days upon tamoxifen treatment. Before dying, the mice exhibited rapid weight loss, which was accompanied by high serum triglyceride levels and attenuation of metabolic processes including glucose and fatty acid metabolism in the liver [Citation49]. Moreover, visceral fat was dramatically depleted [Citation49], indicating a potential role of NAMPT in adipose tissue homoeostasis.
Similarly, adipocyte-specific Nampt knockout mice, generated by mating exon 3-floxed mice and adiponectin-Cre mice, exhibited reduced fat mass [Citation23]. The results were consistent with the results from in vitro experiments with 3T3-L1 cell lines investigating the function and mechanism of NAMPT in adipocytes [Citation13,Citation20,Citation53–55]. In the process of adipocyte differentiation, genes associated with NAD+ synthesis via the salvage pathway were significantly up-regulated [Citation54]. Treatment of the NAMPT inhibitor FK866 significantly blocked adipocyte differentiation [Citation54] and lipid synthesis [Citation53] in vitro, confirming the crucial role of NAMPT in these processes. In terms of circadian rhythm, adipocyte-specific Nampt deletion targeting exon 3 showed disrupted circadian transcriptome in WAT and BAT [Citation52]. Especially in BAT, disrupted rhythm of metabolites in energy metabolism occurred [Citation52], indicating that fat tissue controls peripheral clock leading to regulate metabolic biorhythms in NAMPT-dependent manner. However, despite under the same mouse model, food intake was differently reported [Citation23,Citation52] which might be due to other factors such as age of mice.
Another adipocyte-specific Nampt knockout model obtained by mating exon 5–6-floxed mice and adiponectin-Cre mice showed inconsistent results [Citation20–22,Citation51]. The mouse model did not show changes in fat mass compared to the wild-type [Citation20,Citation51], although systemic insulin resistance was detected [Citation20,Citation22]. Despite a lack of significant effects on fat mass, the model exhibited impaired lipolysis and elevated inflammation levels in WAT [Citation20,Citation22], which was consistent with previous reports showing the anti-inflammatory effects of NMN supplementation in wild-type mice [Citation15,Citation47]. The inconsistent results regarding the effects of NAMPT on fat mass might be attributed to differences in the sex and age of the experimental organisms and/or the knockout strategy employed to obtain the models. Sex-dependent metabolic differences have previously been explored in mouse studies [Citation56] and the results have demonstrated that males became leaner in response to food restriction, whereas females exhibited more fat accumulation to protect themselves from energy imbalances. The increased energy storage capacity of females is a consequence of menstrual periods, which was linked to differences in sex hormones [Citation57]. These differences might contribute to sex-specific differences in transgenerational inheritance of metabolic stress, as reported in previous studies [Citation58,Citation59].
Additionally, the effects of NMN on adipogenic differentiation remain unclear [Citation53–55]. NMN supplementation significantly recovered adipogenesis, which was hindered by NAMPT inhibition via FK866 treatment [Citation53,Citation54]. However, NMN did not seem to affect adipogenesis when NAMPT was not inhibited [Citation55]. These results suggest that the effects of NMN treatment vary depending on the availability of NAMPT in adipocytes. Therefore, additional studies are needed to understand the roles of NAD+ precursors and NAMPT in the regulation of adipocyte development and metabolism.
5. Conclusion
NAD metabolism is a central pathway involved in energy and redox homoeostasis, which in turn determines the risk of chronic disease and ageing. Moreover, current studies have suggested that the roles and effects of NAD are tissue-specific. Enhancing NAD+ improves hepatic insulin sensitivity and circadian rhythm in the liver, while also promoting mitochondrial function in the muscle. Although few studies have assessed the effects of NAD+ metabolism on WAT, current evidence suggests that supplementation with NAD+ precursors reduce fat accumulation and inflammation in fat tissue. Nevertheless, research on the direct effects of NAD+ metabolism on adipocytes using the adipocyte-specific Nampt deletion mouse model or in vitro cell models has rendered inconclusive results, which might be attributed to sex or age differences, and/or basal levels of cellular NAD+ availability, respectively. Further studies are thus needed to fully understand the effects of NAD+ supplementation and the distinct functions of NAMPT in adipocytes.
Abbreviations
ACMS | = | α-amino-β-carboxymuconate-ε-semialdehyde; |
BAT | = | brown adipose tissue; |
BMAL1 | = | basic helix-loop-helix ARNT like 1; |
CD38 | = | cluster of differentiation 38; |
CR | = | calorie restriction; |
FOXO1 | = | forkhead box protein O1; |
GSTA2 | = | glutathione S-transferase alpha 2; |
HFD | = | high-fat diet; NA, nicotinic acid; |
NAD | = | nicotinamide adenine dinucleotide; |
NAM | = | nicotinamide; |
NAMPT | = | nicotinamide phosphoribosyltransferase; |
NMN | = | nicotinamide mononucleotide; |
NMNAT | = | nicotinamide mononucleotide adenylyltransferase; |
NR | = | nicotinamide riboside; |
PARP | = | poly (ADP-ribose) polymerase; |
PER2 | = | period circadian regulator 2; |
SIRT | = | sirtuin; |
UCP1 | = | uncoupling protein 1; |
WAT | = | white adipose tissue. |
Author contributions
Park YJ has made contributed to the conceptualization, study design, and funding acquisition. Under Park JY’s supervision, Kwon SY performed data collection and prepared a manuscript. The final manuscript underwent review and editing by all authors.
Disclosure statement
There are no relevant financial or non-financial competing interests to report.
Data availability statement
The authors confirm that the data supporting the findings of this study are available within the article [and/or] its supplementary materials.
Additional information
Funding
References
- Aman Y, Qiu Y, Tao J, et al. Therapeutic potential of boosting NAD+ in aging and age-related diseases. Transl Med Aging. 2018;2:30–9. doi: 10.1016/j.tma.2018.08.003
- Schultz MB, Sinclair DA. Why NAD+ declines during aging: it’s destroyed. Cell Metab. 2016;23(6):965–966. doi: 10.1016/j.cmet.2016.05.022
- Camacho-Pereira J, Tarragó MG, Chini CCS, et al. CD38 dictates age-related NAD decline and mitochondrial dysfunction through an SIRT3-dependent mechanism. Cell Metab. 2016;23(6):1127–1139. doi: 10.1016/j.cmet.2016.05.006
- Rahman S, Islam R. Mammalian Sirt1: insights on its biological functions. Cell Commun Signal. 2011;9(1):11. doi: 10.1186/1478-811X-9-11
- Booth Lauren N, Brunet A. The Aging Epigenome. Mol Cell. 2016;62(5):728–744. doi: 10.1016/j.molcel.2016.05.013
- Oberdoerffer P, Michan S, McVay M, et al. SIRT1 redistribution on chromatin promotes genomic stability but alters gene expression during aging. Cell. 2008;135(5):907–18. doi: 10.1016/j.cell.2008.10.025
- Rogina B, Helfand SL. Sir2 mediates longevity in the fly through a pathway related to calorie restriction. Proc Natl Acad Sci U S A. 2004;101(45):15998–16003. doi: 10.1073/pnas.0404184101
- Lu S-P, Lin S-J. Regulation of yeast sirtuins by NAD+ metabolism and calorie restriction. Biochim Biophys Acta Proteins Proteom. 2010;1804(8):1567–1575. doi: 10.1016/j.bbapap.2009.09.030
- Harden A, Young WJ. The alcoholic ferment of yeast-juice. Part II.–the conferment of yeast-juice. Proc R Soc Lond B Biol Sci. 1906;78:369–375.
- Warburg O, Christian W. Pyridin, der wasserstoffübertragende Bestandteil von Gärungsfermenten. Helv Chim Acta. 1936;19(1):E79–E88. doi: 10.1002/hlca.193601901199
- Lanska DJ. The discovery of niacin, biotin, and pantothenic acid. Ann Nutr Metab. 2012;61(3):246–53. doi: 10.1159/000343115
- Rajman L, Chwalek K, Sinclair DA. Therapeutic potential of NAD-Boosting molecules: the in vivo evidence. Cell Metab. 2018;27(3):529–547. doi: 10.1016/j.cmet.2018.02.011
- Ryu KW, Nandu T, Kim J, et al. Metabolic regulation of transcription through compartmentalized NAD(+) biosynthesis. Science. 2018;360(6389). doi: 10.1126/science.aan5780
- Yoshino J, Mills KF, Yoon MJ, et al. Nicotinamide mononucleotide, a key NAD(+) intermediate, treats the pathophysiology of diet- and age-induced diabetes in mice. Cell Metab. 2011;14(4):528–536. doi: 10.1016/j.cmet.2011.08.014
- Mills KF, Yoshida S, Stein LR, et al. Long-term administration of nicotinamide mononucleotide mitigates age-associated physiological decline in mice. Cell Metab. 2016;24(6):795–806. doi: 10.1016/j.cmet.2016.09.013
- Yoshino M, Yoshino J, Kayser BD, et al. Nicotinamide mononucleotide increases muscle insulin sensitivity in prediabetic women. Science. 2021;372(6547):1224–1229. doi: 10.1126/science.abe9985
- Elhassan YS, Kluckova K, Fletcher RS, et al. Nicotinamide riboside augments the aged human skeletal muscle NAD(+) metabolome and induces transcriptomic and anti-inflammatory signatures. Cell Rep. 2019;28(7):1717–1728.e6. doi: 10.1016/j.celrep.2019.07.043
- Zhou CC, Yang X, Hua X, et al. Hepatic NAD(+) deficiency as a therapeutic target for non-alcoholic fatty liver disease in ageing. Br J Pharmacol. 2016;173(15):2352–2368. doi: 10.1111/bph.13513
- Adeghate E. Visfatin: structure, function and relation to diabetes mellitus and other dysfunctions. Curr Med Chem. 2008;15(18):1851–62. doi: 10.2174/092986708785133004
- Stromsdorfer KL, Yamaguchi S, Yoon MJ, et al. NAMPT-Mediated NAD(+) biosynthesis in adipocytes regulates adipose tissue function and multi-organ insulin sensitivity in mice. Cell Rep. 2016;16(7):1851–1860. doi: 10.1016/j.celrep.2016.07.027
- Yamaguchi S, Franczyk MP, Chondronikola M, et al. Adipose tissue NAD(+) biosynthesis is required for regulating adaptive thermogenesis and whole-body energy homeostasis in mice. Proc Natl Acad Sci U S A. 2019;116(47):23822–23828. doi: 10.1073/pnas.1909917116
- Franczyk MP, Qi N, Stromsdorfer KL, et al. Importance of adipose tissue NAD+ biology in regulating metabolic flexibility. Endocrinology. 2021;162(3). doi: 10.1210/endocr/bqab006
- Nielsen KN, Peics J, Ma T, et al. NAMPT-mediated NAD+ biosynthesis is indispensable for adipose tissue plasticity and development of obesity. Mol Metab. 2018;11:178–188. doi: 10.1016/j.molmet.2018.02.014
- Fukuwatari T, Shibata K. Nutritional aspect of tryptophan metabolism. Int J Tryptophan Res. 2013;6(Suppl 1):3–8. doi: 10.4137/IJTR.S11588
- Fukuwatari T, Wada H, Shibata K. Age-related alterations of B-Group vitamin contents in urine, blood and liver from rats. J Nutr Sci Vitaminol. 2008;54(5):357–362. doi: 10.3177/jnsv.54.357
- Ramsey KM, Yoshino J, Brace CS, et al. Circadian clock feedback cycle through NAMPT-mediated NAD+ biosynthesis. Science. 2009;324(5927):651–4. doi: 10.1126/science.1171641
- Chen D, Bruno J, Easlon E, et al. Tissue-specific regulation of SIRT1 by calorie restriction. Genes Dev. 2008;22(13):1753–1757. doi: 10.1101/gad.1650608
- Ray Chaudhuri A, Nussenzweig A. The multifaceted roles of PARP1 in DNA repair and chromatin remodelling. Nat Rev Mol Cell Biol. 2017;18(10):610–621. doi: 10.1038/nrm.2017.53
- Sporty J, Lin SJ, Kato M, et al. Quantitation of NAD+ biosynthesis from the salvage pathway in Saccharomyces cerevisiae. Yeast. 2009;26(7):363–9. doi: 10.1002/yea.1671
- Levine DC, Hong H, Weidemann BJ, et al. NAD(+) controls circadian reprogramming through PER2 nuclear translocation to counter aging. Mol Cell. 2020;78(5):835–849.e7. doi: 10.1016/j.molcel.2020.04.010
- Imai S, Yoshino J. The importance of NAMPT/NAD/SIRT1 in the systemic regulation of metabolism and ageing. Diab Obes Metab. 2013;15(S3):26–33. doi: 10.1111/dom.12171
- Xiong X, Yu J, Fan R, et al. NAMPT overexpression alleviates alcohol-induced hepatic steatosis in mice. PloS One. 2019;14(2):e0212523. doi: 10.1371/journal.pone.0212523
- Uddin GM, Youngson NA, Chowdhury SS, et al. Administration of Nicotinamide Mononucleotide (NMN) reduces metabolic impairment in male mouse offspring from obese mothers. Cells. 2020;9(4):791. doi: 10.3390/cells9040791
- Uddin GM, Youngson NA, Doyle BM, et al. Nicotinamide mononucleotide (NMN) supplementation ameliorates the impact of maternal obesity in mice: comparison with exercise. Sci Rep. 2017;7(1):15063. doi: 10.1038/s41598-017-14866-z
- Frontera WR, Ochala J. Skeletal muscle: a brief review of structure and function. Calcif Tissue Int. 2015;96(3):183–195. doi: 10.1007/s00223-014-9915-y
- Tallis J, James RS, Seebacher F. The effects of obesity on skeletal muscle contractile function. J Exp Biol. 2018;221(Pt 13). doi: 10.1242/jeb.163840
- Cantó C, Houtkooper RH, Pirinen E, et al. The NAD(+) precursor nicotinamide riboside enhances oxidative metabolism and protects against high-fat diet-induced obesity. Cell Metab. 2012;15(6):838–847. doi: 10.1016/j.cmet.2012.04.022
- Frederick DW, Loro E, Liu L, et al. Loss of NAD homeostasis leads to progressive and reversible degeneration of skeletal muscle. Cell Metab. 2016;24(2):269–82. doi: 10.1016/j.cmet.2016.07.005
- Park JH, Long A, Owens K, et al. Nicotinamide mononucleotide inhibits post-ischemic NAD+ degradation and dramatically ameliorates brain damage following global cerebral ischemia. Neurobiol Dis. 2016;95:102–110. doi: 10.1016/j.nbd.2016.07.018
- Yamamoto T, Byun J, Zhai P, et al. Nicotinamide mononucleotide, an intermediate of NAD+ synthesis, protects the heart from ischemia and reperfusion. PloS One. 2014;9(6):e98972. doi: 10.1371/journal.pone.0098972
- Bertoldo MJ, Listijono DR, W-HJ H, et al. NAD+ repletion rescues female fertility during reproductive aging. Cell Rep. 2020;30(6):1670–1681.e7. doi: 10.1016/j.celrep.2020.01.058
- Yang Q, Cong L, Wang Y, et al. Increasing ovarian NAD+ levels improve mitochondrial functions and reverse ovarian aging. Free Radic Biol Med. 2020;156:1–10. doi: 10.1016/j.freeradbiomed.2020.05.003
- Youngson NA, Uddin GM, Das A, et al. Impacts of obesity, maternal obesity and nicotinamide mononucleotide supplementation on sperm quality in mice. Reprod. 2019;158(2):169–179. doi: 10.1530/REP-18-0574
- Kershaw EE, Flier JS. Adipose tissue as an endocrine organ. J Clin Endocrinol Metab. 2004;89(6):2548–2556. doi: 10.1210/jc.2004-0395
- Townsend K, Tseng YH. Brown adipose tissue: recent insights into development, metabolic function and therapeutic potential. Adipocyte. 2012;1(1):13–24. doi: 10.4161/adip.18951
- Trayhurn P, Beattie JH. Physiological role of adipose tissue: white adipose tissue as an endocrine and secretory organ. Proc Nutr Soc. 2001;60(3):329–339. doi: 10.1079/PNS200194
- Kim MB, Pham TX, vanLuling M, et al. Nicotinamide riboside supplementation exerts an anti-obesity effect and prevents inflammation and fibrosis in white adipose tissue of female diet-induced obesity mice. J Nutr Biochem. 2022;107:109058. doi: 10.1016/j.jnutbio.2022.109058
- Luo C, Yang C, Wang X, et al. Nicotinamide reprograms adipose cellular metabolism and increases mitochondrial biogenesis to ameliorate obesity. J Nutr Biochem. 2022;107:109056. doi: 10.1016/j.jnutbio.2022.109056
- Zhang LQ, Van Haandel L, Xiong M, et al. Metabolic and molecular insights into an essential role of nicotinamide phosphoribosyltransferase. Cell Death Dis. 2017;8(3):e2705. doi: 10.1038/cddis.2017.132
- Dall M, Trammell SAJ, Asping M, et al. Mitochondrial function in liver cells is resistant to perturbations in NAD+ salvage capacity. J Biol Chem. 2019;294(36):13304–13326. doi: 10.1074/jbc.RA118.006756
- Yoon M J, Yoshida M, Johnson S, et al. SIRT1-mediated eNAMPT secretion from adipose tissue regulates hypothalamic NAD+ and function in mice. Cell Metab. 2015;21(5):706–717. doi: 10.1016/j.cmet.2015.04.002
- Basse AL, Nielsen KN, Karavaeva I, et al. NAMPT-dependent NAD(+) biosynthesis controls circadian metabolism in a tissue-specific manner. Proc Natl Acad Sci U S A. 2023;120(14):e2220102120. doi: 10.1073/pnas.2220102120
- Mitani T, Watanabe S, Wada K, et al. Intracellular cAMP contents regulate NAMPT expression via induction of C/EBPβ in adipocytes. Biochem Biophys Res Commun. 2020;522(3):770–775. doi: 10.1016/j.bbrc.2019.11.165
- Okabe K, Nawaz A, Nishida Y, et al. NAD+ metabolism regulates preadipocyte differentiation by enhancing α-ketoglutarate-mediated histone H3K9 demethylation at the PPARγ promoter. Front Cell Dev Biol. 2020;8:8. doi: 10.3389/fcell.2020.586179
- Majeed Y, Halabi N, Madani AY, et al. SIRT1 promotes lipid metabolism and mitochondrial biogenesis in adipocytes and coordinates adipogenesis by targeting key enzymatic pathways. Sci Rep. 2021;11(1):8177. doi: 10.1038/s41598-021-87759-x
- de Souza GO, Wasinski F, Donato J. Characterization of the metabolic differences between male and female C57BL/6 mice. Life Sci. 2022;301:120636. doi: 10.1016/j.lfs.2022.120636
- Varlamov O, Bethea CL, Roberts CT. Sex-specific differences in lipid and glucose metabolism. Front Endocrinol. 2014;5:241. doi: 10.3389/fendo.2014.00241
- Park JH, Yoo Y, Cho M, et al. Diet-induced obesity leads to metabolic dysregulation in offspring via endoplasmic reticulum stress in a sex-specific manner. Int J Obes. 2018;42(2):244–251. doi: 10.1038/ijo.2017.203
- Ng SF, Lin RC, Laybutt DR, et al. Chronic high-fat diet in fathers programs β-cell dysfunction in female rat offspring. Nature. 2010;467(7318):963–966. doi: 10.1038/nature09491