ABSTRACT
Breakdown of blood-brain barrier (BBB) represents a key pathology in hyperglycemia-mediated cerebrovascular damage after an ischemic stroke. As changes in the level and nature of vasoactive agents released by endothelial cells (ECs) may contribute to BBB dysfunction, this study first explored the specific impact of hyperglycemia on EC characteristics and secretome. It then assessed whether secretome obtained from ECs subjected to normoglycaemia or hyperglycemia might regulate pericytic cytokine profile differently. Using a triple cell culture model of human BBB, composed of brain microvascular EC (BMEC), astrocytes and pericytes, this study showed that exposure to hyperglycemia (25 mM D-glucose) for 72 h impaired the BBB integrity and function as evidenced by decreases in transendothelial electrical resistance and increases in paracellular flux of sodium fluorescein. Dissolution of zonula occludens-1, a tight junction protein, and appearance of stress fibers appeared to play a key role in this pathology. Despite elevations in angiogenin, endothelin-1, interleukin-8 and basic fibroblast growth factor levels and a decrease in placental growth factor levels in BMEC subjected to hyperglycemia vs normoglycaemia (5.5 mM D-glucose), tubulogenic capacity of BMECs remained similar in both settings. Similarly, pericytes subjected to secretome obtained from hyperglycemic BMEC released higher quantities of macrophage migration inhibitory factor and serpin and lower quantities of monocyte chemoattractant protein-1, intercellular adhesion molecule, interleukin-6 and interleukin-8. Taken together these findings indicate the complexity of the mechanisms leading to BBB disruption in hyperglycemic settings and emphasize the importance of endothelial cell-pericyte axis in the development of novel therapeutic strategies.
Introduction
Stroke continues to be one of the leading causes of mortality and morbidity worldwide.Citation1 Each year, >15 million people suffer a first stroke, with two-thirds of them experiencing death or long-term disability.Citation2 Brain edema stemming from the breakdown of blood-brain barrier (BBB) accounts for the majority of deaths during the first week after an ischemic stroke. The BBB controls the selective passage of circulating elements and toxic metabolites between the blood and the brain tissue and is formed by brain microvascular endothelial cells (BMEC), capillary basement membrane, astrocyte endfeet and pericytes. Tight junctions, a multi-protein complex, composed of occludin, claudins and zonula occludes, account for much of the restraining role of the BBB.Citation3,Citation4 As cerebral edema, stemming from BBB breakdown, is more prevalent and severe in stroke patients with diabetes, hyperglycemia (HG) is likely to play a critical role in this defect.Citation5 Although various mechanisms, notably inductions of polyol pathway, oxidative stress and protein kinase C-β/RhoA/Rho-kinase/MLC2 pathways have been implicated in cerebrovascular pathologies, the role of other mechanisms by which HG may compromise cerebral vasculature remain unexplored.Citation6–8
Endothelial cells (ECs) cover the entire inner surface of all blood vessels and constitute the main cellular component of the BBB. They modulate cerebral perfusion in response to neuronal demand and regulate coagulation, immune responses, vascular tone and permeability by releasing a wide range of vasoactive substances such as nitric oxide, prostacyclins and endothelin-1 (ET-1).Citation9–11 Given the diversity of these functions, it is of paramount importance to maintain EC integrity and function at all times to maintain vascular homeostasis. Pericytes that are embedded in capillary basement membrane also contribute to vascular stability and immune modulation and help regulate BBB function and cerebral blood flow.Citation12–14 Due to their reparative and stabilizing roles following ischemic injury, pericytes may also serve as important therapeutic targets for stroke. Indeed, pericyte deficiency in vivo is closely associated with elevations in tight junction dysfunction and endothelial transcytosis.Citation15 HG is known to be a direct cause of pericyte apoptosis and loss.Citation16
Following a cerebral ischemic event, various inflammatory cells, such as leukocytes and microglia, are activated and accumulate within the brain tissue, eventually leading to inflammatory injury. Management of post-stroke inflammatory responses is of pivotal importance. Inflammation can promote tissue repair and axonal regeneration, especially through upregulation of IL-1 and IL-4.Citation17 However, it can also exacerbate tissue damage by promoting secondary pathologies like oxidative stress and excitotoxicity.Citation18,Citation19 While microglia, astrocytes, and leukocytes are known initiators of cerebral inflammation, pericytes can also contribute to this process.Citation20
Given that ECs and pericytes jointly support cerebrovascular recovery through angiogenesis, vasculogenesis and the modulation of immune responses and hyperglycemia is known to adversely affect these processes,Citation21 this study explores how HG affects EC and BBB characteristics in hyperglycemic settings. It also investigates whether changes induced in EC secretory profile by HG may influence pericyte cytokine profile and thus exacerbate BBB damage.
Materials and methods
Cell culture
Human brain microvascular endothelial cells (HBMEC, Neuromics, USA), pericytes (HP, Neuromics, USA and astrocytes (HA, Neuromics, USA) grown up to passage 8 in respective media and supplements were used in relevant experiments. HBMEC were grown to full confluence before exposure to normoglycaemia (NG; 5.5 mM D-glucose), hyperglycemia (HG, 25 mM D-glucose) or D-mannitol (osmotic control, 5.5 mM D-glucose +19.5 mM D-mannitol) for 72 h. Cells were visualized and photographed by light microscopy (Leica DFC3000 G, Wetzlar, Germany).
Establishment of in vitro model of human BBB
In this study, a triple cell culture model of human BBB, composed of BMEC, astrocytes and pericytes was employed. Due to well-established interactions amongst all cell layers, this model is preferred to other contact and non-contact cell culture models.Citation22 To set up the model, ~7.5 × 104 astrocytes were seeded on the outer surface of transwell inserts as described before.Citation22 On the following day, the inserts were inverted the correct way before seeding ~ 4 × 104 HBMECs onto the inner surface. Both cell layers were then cultured to full confluence in their respective media before transferring the filters to fresh 12-well plates containing confluent pericytes.
Analyses of BBB integrity and function
The BBB integrity and function were studied successively by measurements of trans-endothelial electrical resistance (TEER) and the paracellular flux of low and high molecular weight permeability markers, sodium fluorescein (NaF, 376 Da) or Evan’s blue-labeled albumin (EBA, 67kDa). The TEER was measured using an EVOM resistance meter and STX electrodes (World Precision Instruments, Hertfordshire, UK). After TEER assessments, the inserts were rinsed with Hank’s Balanced Salt Solution (HBSS) and transferred to fresh 12-well plates containing 2 ml HBSS. To evaluate paracellular flux, 500 μl of NaF (50 μg/ml NaF) or EBA (165 μg/ml) was introduced into the luminal chamber of each insert and incubated for 1 hour. 400 μl of solutions from both the luminal and abluminal chambers were then collected and analyzed in triplicate using a FLUOstar Omega microplate reader (BMG Labtech Ltd, UK). The flux across the barrier was calculated as before.Citation22
Immunocytochemistry
To investigate possible changes in subcellular localization of tight junction protein zonula occludens-1 (ZO-1) and in actin cytoskeleton organization, HBMEC were grown to about 80–90% confluence on glass coverslips before fixation (4% paraformaldehyde for 15 min) and permeabilisation (0.1% Triton X-100 for 15 min). To study ZO-1 localization, the cells were successively incubated with a ZO-1 primary antibody (1:200, ThermoFisher, UK) and a fluorescently labeled secondary antibody (1:250, Abcam, UK). To study cytoskeletal organization, the cells were stained with rhodamine phalloidin for 30 minutes. The coverslips were then mounted onto glass slides using a mounting medium (Vector Laboratories, Peterborough, UK). The cells were visualized by a fluorescence microscopy (pE-300white, Andover, Hampshire).
Tubulogenic assay
The angiogenic potential of HBMEC subjected to NG, HG or D-mannitol was evaluated over a period of three days by tubulogenesis assay. To this end, HBMEC were carefully seeded onto a layer of reduced Matrigel spread in a 96-well plate. The formation of tubular structures was visualized using a microscope (Leica DFC3000 G, Wetzlar, Germany) that captured detailed images from various fields in each well. These images were then analyzed using the ImageJ software, equipped with the Angiogenesis Analyzer plugin. The analysis involved calculating both the total number and the cumulative length of the tubular networks formed which included the sum of all segments and branches within the observed area.
Analyses of secretome
To obtain the secretome from HBMEC subjected to NG or HG, the respective culture media were collected and condensed by filter centrifugation as per the manufacturer’s instructions (Amicon Ultra-15 Centrifugal Filter Units, Merck Group, Darmstadt, Germany). In some experiments, pericytes were treated with the condensed secretomes for 24 hours prior to evaluation of pericyte cytokine expression using proteome profiler kit.
Protein profiler assays
To analyze the contents of endothelial cell secretome and cytokine profile of pericytes subjected to HBMEC secretome, a proteome profiler human angiogenesis array kit (R&D systems, Minnesota, United States) and a human cytokine array kit (R&D systems, Minnesota, United States) were used as per the manufacturers’ instructions. While the former concomitantly assesses 55 proteins and the latter assesses 103 cytokines.
Statistical analysis
The data are presented as mean ± SEM from at least three independent experiments. Statistical analyses involved unpaired Student’s t-test for two-group comparisons and one-way or two-way ANOVA for multiple group comparisons, followed by Tukey’s test for post-hoc analysis. GraphPad Prism 9 was used for all statistical analyses. p < 0.05 was considered as statistically significant.
Results
Impact of hyperglycaemia on blood-brain barrier integrity and function
Scrutiny of the effects of HG on BBB integrity over three days led to marked disruptions in integrity at 48 and 72 h as ascertained by decreases in TEER compared to the values obtained from BBB subjected to NG or D-mannitol (). Studies assessing the effect of HG on BBB function showed significant increases in paracellular flux of a low molecular weight (NaF, 376 Da) but not a high molecular weight permeability marker EBA (67 kDa) compared to NG and D-mannitol groups ().
Figure 1. Exposure of an in vitro model of human blood-brain barrier, consisting of brain microvascular endothelial cells, astrocytes and pericytes, to hyperglycemia (HG) for 24–72 h led to significant impairments in barrier integrity (a) compared to those exposed to normoglycaemia (NG) and D-mannitol. Although no differences were observed in paracellular flux of high molecular weight marker EBA across different experimental groups (b), the flux of low molecular weight marker sodium fluorescein (NaF) was higher (c) in HG group compared to both NG and D-mannitol groups. Data are expressed as mean±SEM from six independent experiments. ***p < 0.001 compared to HG group.
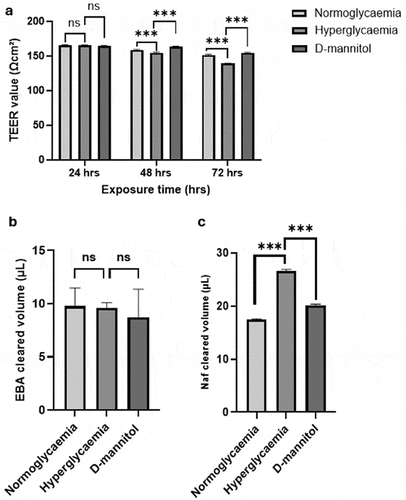
Effect of hyperglycaemia on subcellular localisation of zonula occludens-1 and actin filaments
To investigate whether disruptions in tight junctional complex formation might account for the HG-mediated increases in BBB permeability, the subcellular localization of ZO-1, a key tight junction protein, was investigated in HBMECs subjected to HG, NG or D-mannitol. The ECs cultured under NG and D-mannitol conditions showed typical plasma membrane staining of ZO-1. The uniform ZO-1 localization in these conditions suggests that neither of these conditions disrupt the normal tight junctional organization. In contrast, HBMECs cultured under HG conditions displayed a punctate and discontinuous staining on plasma membrane with visible gaps between cells ().
Figure 2. Treatment of human brain microvascular endothelial cells with high glucose (HG) leads to disappearance of tight junction protein, zonula occludens-1 from plasma membrane (a) and increases actin stress fiber formation in a time-dependent manner (b-c) compared to cells subjected to normoglycaemia (NG) or equimolar concentrations of D-mannitol. Scale bars: 100 μm for ZO-1 and 20 μm for actin microfilament staining. All images were captured using 20× magnification. ****p < 0.001 compared to HG group.
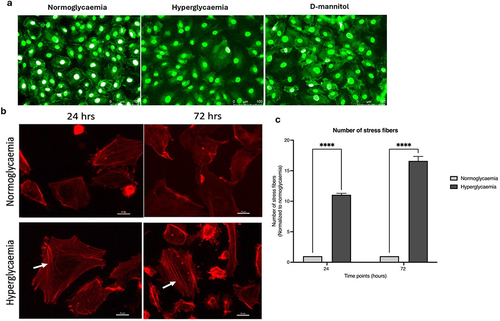
Similarly, to investigate the effects of high levels of glucose on actin cytoskeletal organization, HBMECs were subjected to HG for 24 and 72 h which led to time-dependent significant increases in actin stress fiber formation (). These changes were previously shown to be independent of increased osmolality.Citation6
Impact of hyperglycaemia on tubulogenesis
Exposure of HBMECs to NG, HG or D-mannitol for 72 h did not produce significant differences in tubule formation (), number of nodes (), number of junctions (), mesh area (), length of tubes formed () and total segment length ().
Figure 3. Human brain microvascular endothelial cells subjected to hyperglycemia (HG), normoglycaemia (NG) or D-mannitol did not show significant difference in their angiogenic capacity as evidenced by similarities in morphology (a), the number of junctions (b), number of branching points (c), mesh area (d), total length (e) and total segment length (f). Data are expressed as mean±SEM from six independent experiments. The tubules were visualized using 10× magnification.
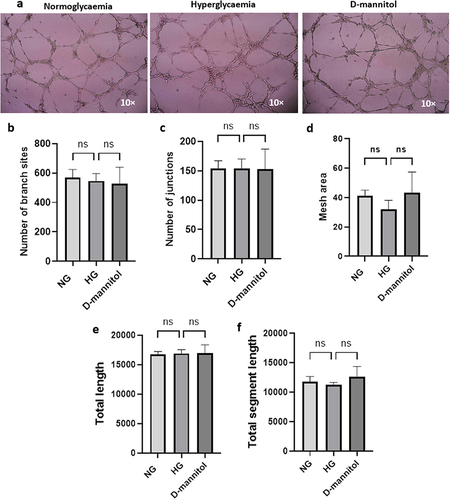
Impact of hyperglycaemia on angiogenic components
Comparative analyses of BMEC conditioned media obtained from cells subjected to NG or HG revealed significant increases in angiogenin, ET-1, basic fibroblast growth factor (bFGF) and interleukin-8 (IL-8) levels and a significant decrease in placental growth factor (PIGF) in the HG group. The levels of angiopoietin-2 (Ang-2) and vascular endothelial growth factor (VEGF) were similar in both groups (). These results indicate that HG regulates the expression or availability of different angiogenic factors in a different manner.
Figure 4. Analysis of angiogenic factors in human brain microvascular endothelial cells subjected to hyperglycemia (HG) or normoglycaemia (NG) revealed significant alterations in the levels of angiogenin, endothelin-1, basic fibroblast growth factor (bFGF), interleukin-8 (IL-8) and placental GF (PlGF) but not angiopoietin-2 (ang-2) and vascular endothelial GF (VEGF). Data are expressed as mean±SEM from three independent experiments. **p < 0.01 compared to HG group. ***p < 0.001 compared to HG group.
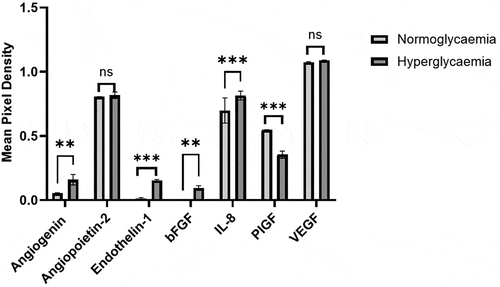
Impact of endothelial cell secretome on pericytic cytokine expression
Pericytes incubated with the secretome of HBMEC subjected to HG showed significant decreases in monocyte chemoattractant protein-1 (MCP-1), intercellular adhesion molecule-1 (ICAM-1) and IL-8 and an increase in macrophage migration inhibitory factor (MIF) levels compared to their counterparts incubated with the secretome of HBMEC subjected to NG. The levels of IL-6, plasminogen activator inhibitor-1 (PAI-1) and growth-regulated oncogene-β (GRO-β) remained unchanged in both groups (). These findings reflect the complex alterations in cytokine expression in pericytes under hyperglycemic influence.
Figure 5. Analysis of cytokine profile in pericytes subjected to secretome obtained from human brain microvascular endothelial cells treated with hyperglycemia (HG) or normoglycaemia (NG) revealed significant alterations in the levels of monocyte chemoattractant protein-1 (MCP-1), intercellular adhesion molecule-1 (ICAM-1), interleukin-8 (IL8) and macrophage migration inhibitory factor (MIF) but not growth regulated oncogene-beta (GRO), IL6 and serpin. Data are expressed as mean±SEM from three independent experiments. ***p < 0.001 compared to HG group.
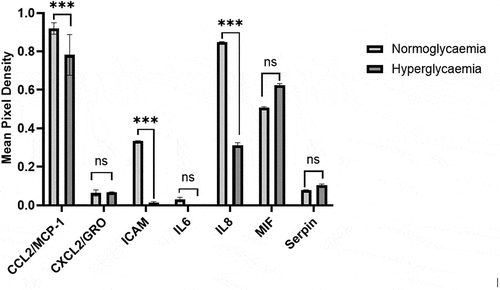
Discussion
The burgeoning epidemic of diabetes poses a significant threat to global health. It is expected that the number of individuals affected by diabetes will be well above 600 million by 2040.Citation23 Particularly alarming is the high incidence of diabetes among stroke patients.Citation24 Given the worse stroke outcome in diabetics, a critical examination of the relationship between diabetes and stroke is imperative. As the majority of stroke patients die from the brain edema within the first week of stroke, it is particularly important to explore the impact of HG on the function and integrity of the BBB and identify mechanisms involved in HG-mediated breakdown of the BBB. In this regard, this study paid a particular attention to tight junctional distribution of ZO-1, BMEC tubulogenic capacity and the changes in EC secretome that are likely to affect cerebrovasculature directly or through their impact on other BBB-forming cells, notably pericytes. Hence, the study specifically explored whether, and how, EC secretome may alter cytokine profile of pericytes.
Our initial studies aiming to consolidate the deleterious effect of HG on BBB has shown that HG compromises the BBB integrity after 2 days of incubation which worsened on day 3. This HG-mediated disruption of the BBB is likely to have profound implications for patients with diabetes who manifest chronic HG.Citation25 As disruption of BBB can heighten the risk of hemorrhagic transformation after thrombolytic therapy and exacerbate cerebral injury and inflammation, the maintenance of BBB integrity is regarded as a key step in stroke management.Citation26 Observation of specific increases in paracellular flux of NaF (367 Da) alone in the present study suggests that HG elicits relatively small openings between neighboring endothelial cells which hinder the passage of high molecular weight molecules as evidenced by EBA (67 kDa) studies. A size-dependent flux in response to HG has also been reported in co-cultures established with hCMEC/D3, an immortalized BME cell line and astrocytes where low (4–10 kDa) but not high molecular weight dextran (70kDa) traveled across the barrier.Citation27
To elucidate whether structural impairments in tight junctions may in part account for diminished TEER, this study investigated the subcellular localization of ZO-1 and revealed a discontinuity in its plasma membrane localization. ZO-1 is an essential component of the tight junction complex, playing a pivotal role in maintaining the integrity of the BBB by forming connections between transmembrane proteins and the actin cytoskeleton.Citation28 The prominent role of ZO-1 in restricting molecular exchange between blood and the brain parenchyma confirms its status as a critical marker of BBB function. It is noteworthy here that changes in subcellular localization of other tight junction proteins, notably claudins and occludin as well as vascular endothelial (VE)-cadherin, an endothelial specific adhesion molecule located at junctions between endothelial cells may also contribute to the HG-induced barrier disruption. Despite a marginal transient increase in VE-cadherin expression in hCMEC/D3 after a short exposure (~3 h) to hyperglycemia,Citation27 longer exposures to HG leads to internalization of VE-cadherin and promotes BBB dysfunction.Citation29 Morphological changes stemming from microfilament reorganization may also contribute to HG-induced BBB dysfunction. In this study, HBMEC grown under NG displayed a cortical actin filament distribution, whereas those subjected to HG had an elongated and cuboidal appearance and developed stress fibers traversing the cells. The cortical actin bands participate in the formation and stabilization of intercellular junctions and therefore play a critical role in control of the paracellular flux. In contrast, stress fibers generate a tensile centripetal force by which pull junctional proteins inward, breakup junctions and thus form inter-endothelial cellular gaps.Citation30,Citation31
Angiogenesis, the formation of blood vessels from preexisting ones, is a process induced by various growth factors, integrins, and enzymes. Under normal circumstances, angiogenesis is required to supply tissues with sufficient quantities of oxygen and nutrients. Stimulation of EC detachment from basement membrane initiates the process which is then spurred on by cell proliferation and migration.Citation32,Citation33 Using the tube formation assay, exploiting ECs’ natural propensity to form vessel-like structures on reduced matrigel,Citation34 no significant changes in tubulogenic capacity were observed in BMEC exposed to HG, NG or D-mannitol. This was evidenced by the similarities in indicators of vascular branching (the number of nodes and junctions) and network complexity (the lengths of the tubular structures). Taken together these findings corroborate the notion that EC metabolism rather than HG per se determines the extent of EC responsiveness to growth factors and angiogenic phenotype.Citation35 Differences in the intensity and longevity of stress as well as in EC characteristics (immortalized vs primary cell lines) may distinctly affect EC metabolism and account for some of the differences previously reported in angiogenic response (enhanced or impaired) to HG.Citation36 This also sheds some light on unaltered tubulogenic activity observed in this study despite increases in various promoters of angiogenesis, namely bFGF, ET-1, angiogenin, angiopoietin-2 (Ang-2) and IL-8.
The collection of molecules secreted by cells into the culture media, aka the secretome, influences the cellular microenvironment and cell-to-cell communication.Citation11 Changes in the composition of secretome can provide insights into cellular responses to different stimuli. Considering the complexity of the EC secretome and its responsiveness to both endogenous and external factors, the present study probed the impact of HG and NG on BMEC secretome. Given the exaggerated release of reactive oxygen species and proinflammatory cytokines in hyperglycemic settings, this was of particular importance.Citation37
Elevations in angiogenin and Ang-2 in hyperglycemic secretome suggest that BMECs attempt to maintain vascular equilibrium despite metabolic challenges. Angiogenin is a secreted ribonuclease that promotes new blood vessel formation while Ang-2 plays a crucial role in EC physiology, angiogenic function and vascular stability. Similarly, increases observed in bFGF, that regulate morphogenesis, cell growth and tissue repair, may also be an adaptive response to hyperglycemic stress. By synthesizing and storing bFGF intracellularly and incorporating it into the extracellular matrix, ECs ensure as to the contribution of bFGF to angiogenic processes as/when required. However, increased availability of bFGF is also coupled to the vascular complications in type 2 diabetic rats, indicating the complexity of mechanisms involved in vascular homeostasis in hyperglycemic settings.Citation38 The concomitant increases observed in IL-8 secretion in BMEC subjected to HG in this study corroborates this notion. Besides being a potent promoter of angiogenesis, IL-8 is also an inducer of vasocontractile, proinflammatory, chemotactic and matrix-degradative responses.Citation39 Neutralisation of nitric oxide, the most prominent endogenous vasodilator, represents the main cause of ET-1-mediated vascular contractility.Citation40 Albeit needs confirming, increases in protein kinase C activity and ET-1 mRNA may somewhat explain HG-mediated ET-1 overexpression in BMECs.Citation41
Contrary to the abovementioned agents, no difference was observed in VEGF expression between the secretomes of BMEC subjected to NG or HG. Although a correlation between VEGF overexpression and elevated TEER stemming from upregulation of ZO-1 transcript and protein has been reported,Citation42 VEGF overexpression is also associated with BBB leakage, cerebral hemorrhage and increased infarction volume. Discontinuous plasma membrane localization of ZO-1 in the current study somewhat suggests inability of VEGF to modulate ZO-1 availability in hyperglycemic settings. Decreases in the levels of PlGF, a member of the VEGF subfamily that contributes to angiogenesis and vasculogenesis especially during embryonic development, may add some weight to this notion.Citation43 As alluded above, the impact of glucose on VEGF expression is far from being universal. For instance, human plasma VEGF levels were reported to increase and decrease in response to hyperglycemia and hypoglycemia, respectively. This may constitute a neuroprotective mechanism and maintain a constant rate of glucose influx to the brain.Citation44 However, both hyperglycemia and hypoglycemia have been shown to induce VEGF expression at 24 h in hCMEC/D3.Citation27 It is of note that despite retaining structural and functional aspects of primary human BMEC over some passages, these immortalized cells do not express specific tight junction molecules and transporters to the same extent as primary cells.Citation45,Citation46 Furthermore, while primary cells in culture conditions change their phenotype and go into senescence after a certain number of passages, immortalized cells can, in theory, proliferate indefinitely.Citation47 These functional differences may somewhat explain some of the differences seen in primary and immortalized human BMECs.
As indicated above, endothelium-derived agents influence vasculature directly and through their effect on the surrounding cells. Amongst all other cells present in microcirculation, pericytes are of particular importance. Because, they contribute to the maintenance of the BBB, regulate cerebral blood flow and control immune cell entry to the central nervous system.Citation12 Pericytes wrap around the ECs and communicate with them through this physical contact as well as via paracrine signaling. Bearing this in mind, the present study examined the specific impact of EC secretome obtained from cells exposed to NG or HG on pericytic cytokine profile which are implicated in various neurovascular disorders.Citation48 This revealed significant decreases in MCP-1, ICAM, IL-8 and elevations in MIF in pericytes exposed to secretome obtained from hyperglycemic BMEC. Taken together these changes imply that hyperglycemic conditions can shift the balance toward impaired fibrinolysis, potentially aggravating tissue damage, neuroinflammation and the risk of thrombus formation after a stroke.Citation49
Intriguingly, the expression of MCP-1, a chemokine that plays a key role in monocyte recruitment, was found to be lower in pericytes cultured in hyperglycemic EC secretome. This may attenuate recruitment of monocytes to the site of vascular damage and somewhat explain the perturbed immune responses and vascular functions reported in diabetics.Citation50 Concurrent decreases induced in pericyte IL-8 and ICAM expressions by hyperglycemic EC secretome may further add to impairments in host defense and provide a mechanistic understanding for the prolonged infections or suboptimal tissue repair in patients with diabetes.Citation51,Citation52 Although the reason for the difference observed in pericyte and EC IL-8 expression is currently unknown, this may simply arise from few counter-regulatory mechanisms exist between the two cell types. The increase in the levels of MIF and serpin E1 in pericytes treated with secretome of BMEC exposed to HG support the hypothesis that the interactions amongst a large number of vasoactive elements capable of affecting vascular integrity and function as well as angiogenesis and inflammation ultimately determine the integrity and function of BBB in physiological and pathological settings.Citation48,Citation53,Citation54
There are some limitations to the study. Firstly, this is an in vitro study using an experimental model of human BBB and employing a single concentration of high glucose for 72 h. These naturally cannot replicate the in vivo conditions where the BBB is also affected by a variety of physiological and circulatory elements such as shear stress, hormones, minerals, vitamins, growth factors, cytokines, etc and the level of blood glucose may vary dramatically. Besides, the experimental model lacking neurons cannot fully replicate the complexity of the neurovascular unit in vivo. As we had previously studied the impact of glycemic control on the BBB,Citation7,Citation55 this study did not focus on this aspect. However, a significant proportion of patients with diabetes would be on some sort of glycemic treatment. It is of note that use of three-dimensional dynamic models, enabling better intercellular interactions that more closely resemble in vivo behavior, may address some of the issues associated with static models of BBB. Ultimately, well-thought in vivo studies considering the abovementioned limitations in their design are needed to establish translational relevance of the findings generated in laboratory studies.
In conclusion, the present study provides some evidence as to how HG impacts the communication between BMEC and pericytes at a secretory level and provides some mechanistic understanding for the HG-mediated BBB damage, paying a particular attention to tight junctional formation and EC tubulogenic capacity.
Disclosure statement
No potential conflict of interest was reported by the authors.
Data availability statement
The datasets used and analyzed during the current study are available from the corresponding author on reasonable request.
Additional information
Funding
References
- Huang Y, Wang Z, Huang Z-X, Liu Z. Biomarkers and the outcomes of ischemic stroke. Front Mol Neurosci. 2023;16:1171101. doi:10.3389/fnmol.2023.1171101.
- Donkor ES. Stroke in the 21st Century: a snapshot of the burden, epidemiology, and quality of life. Stroke Res Treat. 2018;3238165. doi:10.1155/2018/3238165.
- Bayraktutan U. Endothelial progenitor cells: potential novel therapeutics for ischaemic stroke pharmacol res. Pharmacol Res. 2019;144:181–12. doi:10.1016/j.phrs.2019.04.017.
- Allen CL, Bayraktutan U. Risk factors for ischaemic stroke. Intl J Stroke. 2008;3(2):105–116. doi:10.1111/j.1747-4949.2008.00187.x.
- Hisham NF, Bayraktutan U. Epidemiology, pathophysiology and treatment of hypertension in ischaemic stroke patients. J Stroke Cerebrovasc Dis. 2013;22(7):e4–14. doi:10.1016/j.jstrokecerebrovasdis.2012.05.001.
- Srivastava K, Shao B, Bayraktutan U. PKC- β exacerbates in vitro brain barrier damage in hyperglycemic settings via regulation of RhoA/Rho-kinase/MLC2 pathway. J Cereb Blood Flow Metabol. 2013;33(12):1928–1936. doi:10.1038/jcbfm.2013.151.
- Allen CL, Bayraktutan U. Antioxidants attenuate hyperglycaemia-mediated brain endothelial cell dysfunction and blood-brain barrier hyperpermeability. Diabet Obes Metabol. 2009;11(5):480–490. doi:10.1111/j.1463-1326.2008.00987.x.
- Shao B, Bayraktutan U. Hyperglycaemia promotes cerebral barrier dysfunction through activation of protein kinase C-β. Diabet Obes Metab. 2013;15(11):993–999. doi:10.1111/dom.12120.
- Bayraktutan U. Free radicals, diabetes and endothelial dysfunction. Diabet Obes Metabol. 2002;4(4):224–238. doi:10.1046/j.1463-1326.2002.00184.x.
- Bayraktutan U. Reactive oxygen species, nitric oxide and hypertensive endothelial dysfunction. Curr Hypertens Rev. 2005;1(3):201–215. doi:10.2174/157340205774574568.
- Alwjwaj M, Kadir RRA, Bayraktutan U. The secretome of endothelial progenitor cells: a potential therapeutic strategy for ischemic stroke. Neural Regen Res. 2021;16(8):1483–1489. doi:10.4103/1673-5374.303012.
- Attwell D, Mishra A, Hall CN, O’Farrell FM, Dalkara T. What is a pericyte? J Cereb Blood Flow Metab. 2016;36(2):451–455. doi:10.1177/0271678X15610340.
- Bennett HC, Kim Y. Pericytes across the lifetime in the central nervous system. Front Cell Neurosci. 2021;15:627291. doi:10.3389/fncel.2021.627291.
- Thalgott J, Dos-Santos-Luis D, Lebrin F. Pericytes as targets in hereditary hemorrhagic telangiectasia. Front Genet. 2015;6. doi:10.3389/fgene.2015.00037.
- Sweeney MD, Ayyadurai S, Zlokovic BV. Pericytes of the neurovascular unit: key functions and signaling pathways. Nat Neurosci. 2016;19(6):771–783. doi:10.1038/nn.4288.
- Gan J, Huang M, Lan G, Liu L, Xu F. High glucose induces the loss of retinal pericytes partly via NLRP3-caspase-1-GSDMD-Mediated pyroptosis. Biomed Res Int. 2020;23:4510628. doi:10.1155/2020/4510628.
- Fregnan F, Muratori L, Simões AR, Giacobini-Robecchi MG, Raimondo S. Role of inflammatory cytokines in peripheral nerve injury. Neural Regen Res. 2012;15:2259–2266. doi:10.3969/j.issn.1673-5374.2012.29.003.
- Liu R, Pan M-X, Tang J-C, Zhang Y, Liao H, Zhuang Y, Zhao D, Wan Q. Role of neuroinflammation in ischemic stroke. Neuroimmunol Neuroinflammation. 2017;4:158. doi:10.20517/2347-8659.2017.09.
- Matsumoto J, Takata F, Machida T, Takahashi H, Soejima Y, Funakoshi M, Futagami K, Yamauchi A, Dohgu S, Kataoka Y. Tumor necrosis factor-α-stimulated brain pericytes possess a unique cytokine and chemokine release profile and enhance microglial activation. Neurosci Lett. 2014;578:133–138. doi:10.1016/j.neulet.2014.06.052.
- Jansson D, Rustenhoven J, Feng S, Hurley D, Oldfield RL, Bergin PS, Mee EW, Faull RL, Dragunow M. A role for human brain pericytes in neuroinflammation. J Neuroinflammation. 2014;11(1):104. doi:10.1186/1742-2094-11-104.
- Bergers G, Song S. The role of pericytes in blood-vessel formation and maintenance. Neuro Oncol. 2005;7(4):452–464. doi:10.1215/S1152851705000232.
- Abdulkadir RR, Alwjwaj M, Bayraktutan U. Establishment of an in vitro model of human blood-brain barrier to study the impact of ischeamic injury. In: The blood-brain barrier: methods and protocols 1st. Vol. 357. Berlin: Springer; 2022. doi:10.1007/978-1-0716-2289-6-8.
- Ogurtsova K, da Rocha Fernandes J, Huang Y, Linnenkamp U, Guariguata L, Cho N, Makaroff L. IDF diabetes atlas: global estimates for the prevalence of diabetes for 2015 and 2040. Diabetes Res Clin Pract. 2017;128:40–50. doi:10.1016/j.diabres.2017.03.024.
- Lau LH, Lew J, Borschmann K, Thijs V, Ekinci EI. Prevalence of diabetes and its effects on stroke outcomes: a meta-analysis and literature review. J Diabetes Invest. 2019;10(3):780–792. doi:10.1111/jdi.12932.
- Prasad S, Sajja RK, Naik P, Cucullo L. Diabetes mellitus and blood-brain barrier dysfunction: an overview. J Pharmacovigil. 2014;2(2):125. doi:10.4172/2329-6887.1000125.
- Li X, Cai Y, Zhang Z, Zhou J. Glial and vascular cell regulation of the blood-brain barrier in diabetes. Diabetes Metab J. 2022;46(2):222–238. doi:10.4093/dmj.2021.0146.
- Sajja RK, Prasad S, Cucullo L. Impact of altered glycaemia on blood-brain barrier endothelium: an in vitro study using the hCMEC/D3 cell line. Fluid Barriers CNS. 2014;11(1):8. doi:10.1186/2045-8118-11-8.
- Fanning AS, Jameson BJ, Jesaitis LA, Anderson JM. The tight junction protein ZO-1 establishes a link between the transmembrane protein occludin and the actin cytoskeleton. J Biol Chem. 1998;273(45):29745–29753. doi:10.1074/jbc.273.45.29745.
- Li W, Chen Z, Chin I, Chen Z, Dai H. The role of VE-cadherin in blood-brain barrier integrity under central nervous system pathological conditions. Curr Neuropharmacol. 2018;16(9):1375–1384. doi:10.2174/1570159X16666180222164809.
- Allen C, Srivastava K, Bayraktutan U. Small GTPase RhoA and its effector rho kinase mediate oxygen glucose deprivation-evoked in vitro cerebral barrier dysfunction. Stroke. 2010;41(9):2056–2063. doi:10.1161/STROKEAHA.109.574939.
- Kadir RRA, Alwjwaj M, McCarthy Z, Bayraktutan U. Therapeutic hypothermia augments the restorative effects of PKC-β and Nox2 inhibition on an in vitro model of human blood–brain barrier. Metab Brain Dis. 2021;36(7):1817–1832. doi:10.1007/s11011-021-00810-8.
- Bouïs D, Kusumanto Y, Meijer C, Mulder NH, Hospers GAP. A review on pro- and anti-angiogenic factors as targets of clinical intervention. Pharmacol Res. 2006;53(2):89–103. doi:10.1016/j.phrs.2005.10.006.
- Kubota Y, Kleinman HK, Martin GR, Lawley TJ. Role of laminin and basement membrane in the morphological differentiation of human endothelial cells into capillary-like structures. J Cell Biol. 1988;107(4):1589–1598. doi:10.1083/jcb.107.4.1589.
- Arnaoutova I, Kleinman H. In vitro angiogenesis: endothelial cell tube formation on gelled basement membrane extract. Nat Protoc. 2010;5(4):628–635. doi:10.1038/nprot.2010.6.
- Goveia J, Stapor P, Carmeliet P. Principles of targeting endothelial cell metabolism to treat angiogenesis and endothelial cell dysfunction in disease. EMBO Mol Med. 2014;6(9):1105–1120. doi:10.15252/emmm.201404156.
- Eelen G, de Zeeuw P, Treps L, Harjes U, Wong BW, Carmeliet P. Endothelial cell metabolism. Physiol Rev. 2018;98(1):3–58. doi:10.1152/physrev.00001.2017.
- Qazi BS, Tang K, Qazi A. Recent advances in underlying pathologies provide insight into interleukin-8 expression-mediated inflammation and angiogenesis. Int J Inflam. 2011;2011:908468. doi:10.4061/2011/908468.
- Deng J, Liu Y, Liu Y, Li W, Nie X. The multiple roles of fibroblast growth factor in diabetic nephropathy. J Inflamm Res. 2021;14:5273–5290. doi:10.2147/JIR.S334996.
- Apostolakis S, Vogiatzi K, Amanatidou V, Spandidos DA. Interleukin-8 and ardiovascular disease. Cardiovasc Res. 2009;84(3):353–360. doi:10.1093/cvr/cvp241.
- Nishiyama SK, Zhao J, Wray DW, Richardson RS. Vascular function and endothelin-1: tipping the balance between vasodilation and vasoconstriction. J Appl Physiol. 2017;122:354–360. doi:10.1152/japplphysiol.00772.2016.
- Park JY, Takahara N, Gabriele A, Chou E, Naruse K, Suzuma K, Yamauchi T, Ha SW, Meier M, Rhodes CJ. et al. Induction of endothelin-1 expression by glucose: an effect of protein kinase C activation. Diabetes. 2000;49:1239–1248. doi:10.2337/diabetes.49.7.1239.
- Ghassemifar R, Lai CM, Rakoczy PE. VEGF differentially regulates transcription and translation of ZO-1alpha+ and ZO-1alpha- and mediates trans-epithelial resistance in cultured endothelial and epithelial cells. Cell Tissue Res. 2006;323(1):117–125. doi:10.1007/s00441-005-0046-7.
- Cerdeira AS, Karumanchi SA. Angiogenic factors in preeclampsia and related disorders. Cold Spring Harb Perspect Med. 2012;2(11):a006585. doi:10.1101/cshperspect.a006585.
- Oltmanns KM, Melchert UH, Scholand-Engler HG, Schultes B, Schweiger U, Peters A. Divergent effects of hyper- and hypoglycemia on circulating vascular endothelial growth factor in humans. Metabolism. 2008;57:90–94. doi:10.1016/j.metabol.2007.07.022.
- Weksler BB, Subileau EA, Perriere N, Charneau P, Holloway K, Leveque M, Tricoire-Leignel H, Nicotra A, Bourdoulous S, Turowski P. et al. Blood–brain barrier-specific properties of a human adult brain endothelial cell line. Faseb J. 2005;19:1872–1874. doi:10.1096/fj.04-3458fje.
- Tai LM, Reddy PS, Lopez-Ramirez MA, Davies HA, Male DK, Loughlin AJ, Romero IA. Polarized P-glycoprotein expression by the immortalised human brain endothelial cell line, hCMEC/D3, restricts apical-tobasolateral permeability to rhodamine 123. Brain Res. 2009;1292:14–24. doi:10.1016/j.brainres.2009.07.039.
- Ya J, Kadir RRA, Bayraktutan U. Delay of endothelial cell senescence protects cerebral barrier against age-related dysfunction: role of senolytics and senomorphics. Tissue Barriers. 2023;11(3):2103353. doi:10.1080/21688370.2022.2103353.
- Ramesh G, MacLean AG, Philipp MT. Cytokines and chemokines at the crossroads of neuroinflammation, neurodegeneration, and neuropathic pain. Mediators Inflamm. 2013;2013:480739. doi:10.1155/2013/480739.
- Girbl T, Lenn T, Perez L, Rolas L, Barkaway A, Thiriot A, Del Fresno C, Lynam E, Hub E, Thelen M. et al. Distinct compartmentalization of the Chemokines CXCL1 and CXCL2 and the atypical receptor ACKR1 determine discrete stages of neutrophil diapedesis. Immunity. 2018;49(6):1062–1076.e6. doi:10.1016/j.immuni.2018.09.018.
- Amor S, Puentes F, Baker D, van der Valk P. Inflammation in neurodegenerative diseases. Immunology. 2010 Feb;129(2):154–169. doi:10.1111/j.1365-2567.2009.03225.x.
- Ley K, Laudanna C, Cybulsky MI, Nourshargh S. Getting to the site of inflammation: the leukocyte adhesion cascade updated. Nat Rev Immunol. 2007;7(9):678–689. doi:10.1038/nri2156.
- Stark K, Eckart A, Haidari S, Tirniceriu A, Lorenz M, von Brühl ML, Gärtner F, Khandoga AG, Legate KR, Pless R. et al. Capillary and arteriolar pericytes attract innate leukocytes exiting through venules and ‘instruct’ them with pattern-recognition and motility programs. Nat Immunol. 2013;14(1):41–51. doi:10.1038/ni.2477.
- Binder BR, Mihaly J, Prager GW. uPAR-uPA-PAI-1 interactions and signaling: a vascular biologist’s view. Thromb Haemost. 2007;97(3):336–342. doi:10.1160/TH06-11-0669.
- Basu A, Menicucci G, Maestas J, Das A, McGuire P. Plasminogen activator inhibitor-1 (PAI-1) facilitates retinal angiogenesis in a model of oxygen-induced retinopathy. Invest Ophthalmol Vis Sci. 2009;50(10):4974–4981. doi:10.1167/iovs.09-3619.
- Weidig P, McMaster D, Bayraktutan U. High glucose mediates pro-oxidant and antioxidant enzyme activities in coronary endothelial cells. Diabet Obes Metabol. 2004;6(6):432–441. doi:10.1111/j.1462-8902.2004.00364.x.