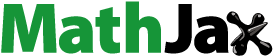
ABSTRACT
Green oceanic microalgae are efficient converters of solar energy into the biomass via the photosynthesis process, with the first step of carbon fixation in the photosynthesis being controlled by the enzyme ribulose-1, 5-bisphosphate carboxylase/oxygenase (RuBisCO), which is a large proteinaceous machine composed of large (L, 52 kDa) and small (S, 12 kDa) subunits arranged as a L8S8 hexadecamer that catalyzes the formation of 2 phosphoglyceric acid molecules from one ribulose 1,5-bisphosphate (RuBP) molecule and one of carbon dioxide (CO2) and that is considered as the most abundant protein on Earth. The catalytic efficiency of this protein is controlled by the RuBisCO activase (RCA) that interacts with RuBisCO and promotes the CO2 entrance to the active site of RuBisCO by removing RuBP. One of the peculiar features of RCA is the presence of functional disordered tails that might play a role in RCA-RuBisCO interaction. Based on their ability to move, microalgae are grouped into 2 major class, motile and non-motile. Motile microalgae have an obvious advantage over their non-motile counterparts because of their ability to actively migrate within the water column to find the most optimal environmental conditions. We hypothesizes that the RCA could be functionally different in the non-motile and motile microalgae. To check this hypothesis, we conducted a comparative computational analysis of the RCAs from the representatives of the non-motile (Ostreococcus tauri) and motile (Tetraselmis sp. GSL018) green oceanic microalgae.
Introduction
Green algae photosynthesis
Green algae are eukaryotic organisms that obtain chemical energy (is stored in carbohydrate molecules) from energy of light via photosynthesis, the process described by the following general reaction:where C6H12O6 is glucose, which is subsequently transformed into other sugars, cellulose, lignin, etc. Green microalgae are typically more efficient than higher plants in transforming solar energy to biomass because of their simple cellular structure, fast growth rates, and abundance of nutrients and CO2 dissolved in water.Citation1 In eukaryotes, photosynthesis occur in the chloroplasts in the 2 set of reactions: light-dependent reaction (light reaction) that occurs specifically in the thylakoid membrane through photosystem I and photosystem II, and the Calvin cycle (dark reaction) that occur in the stroma (see ). During the light dependent reaction, adenosine triphosphate (ATP) and nicotinamide adenine dinucleotide phosphate reduced form (NADPH) are formed:
Figure 1. Oversimplified schematic representation of the photosynthesis process (light reaction and Calvin cycle) that also shows a central role of RuBisCo in carbon fixation.

Then, NADPH and ATP are released in the stroma to fuel the Calvin cycle, which consist of series of reactions to produce Glyceraldehyde 3-phosphate (G3P):
Although, Calvin cycle is also known as light independent reaction or dark reaction, this process occurs only in presence of light. The Calvin cycle consist of 3 major steps: carbon fixation, reduction, and, regeneration of ribulose-1,5-bisphosphate, a 5-carbon sugar (RuBP). The first major step of carbon fixation in the photosynthesis process occurs when the enzyme Ribulose-1, 5-bisphosphate carboxylase/oxygenase (RuBisCO) catalyzes the carboxylation of RuBP. This enzyme is one of the most abundant protein on Earth and is considered by many as one of the most essential factors for all life on earth. It is composed of the large chain (L, ∼55,000 Da) and the small chain (S, ∼13,000 Da) subunits arranged as a L8S8 heterohexadecamer (∼540,000 Da). To illustrate an intricate packing of the RuBisCO subunits, represents half of this large protein complex (PDB ID: 4MKV). RuBisCO is active when a lysine residue inside of its active site is carbamylated (a proton is lost from the amino group and the change in composition is NHCO) and bind a Mg2+ ion.Citation2
RuBisCO and RuBisCo activase (RCA)
Analysis of the concentrations of intermediates found in Calvin cycle combined with the calculations of free energy differences of reactions included in this cycle and evaluation of the control coefficients of the enzymes participating in this pathway provided strong evidence that RuBisCo might represent the main limitation on photosynthesis in C3 plants (which are the most common and the most efficient at photosynthesis in cool, wet climates) and algae at limiting CO2 concentrations and saturating irradiance.Citation3-6 However, RuBisCO is rather inefficient enzyme and is rapidly self-inactivated under the physiological conditions, because its active site can undergo spontaneous decarbamylation (loosing CO2) and strongly binds RuBP or any of the sugar inhibitor analogs such us 3-ketoarabinitol-1,5-bisphosphate (KABP), D-xylulose-1,5-bisphosphate (XuBP), D-glycero-2,3-pentodiulose-1,5-bisphosphate (PDBP), 2-carboxytetritol- 1,4-bisphosphate (CTBP), and 2-carboxy-D-arabinitol 1-phosphate (CA1P). Therefore, the rate of CO2 fixation and the rate of photosynthetic electron transport depend on the RuBisCO regulation.Citation7 As a result, RuBisCO is a highly regulated machine used to control carbon fixation reaction in response of environmental fluctuations.Citation8
RuBisCO activase (RCA) is an enzyme responsible for the RuBisCO activation by uncoupling RuBisCO and RuBP interaction or releasing the bound RuBP or its analog from RuBisCO.Citation9 It allows the rapid formation of the critical carbamate in the active site of RuBisCO by binding the activator CO2.Citation10 It is believed that the presence of RCAs defines the RuBisCO ability to function at its maximal capacity in sub-optimal CO2 concentration that would normally not permit carbamylation in vivo.Citation11 Although microalgae and cyanobacteria contain a CO2-concentrating mechanism and therefore can efficiently increase CO2 concentration,(Aizawa and Miyachi, 1986) the presence RCA in these organisms was shown to be crucial to sustain high-CO2 stress, which dramatically increased expression levels of this protein.Citation12 provides a schematic representation of the RuBisCO activation by RCA.Citation13 Only the active form of RuBisCO is capable of catalyzing CO2 fixation, and the proportion of RuBisCO that is active depends on RCA effectiveness in removing bound RuBP.Citation14
Figure 2. Crystal structure of the half of the RuBisCO hexadecamer (L4S4 complex) from Pisum sativum (PDB ID: 4MKV). To illustrate an intricate packing of the RuBisCO subunits, top (A), side (B), and bottom views (C) of this L4S4 complex are shown.

Figure 3. Schematic representation of the RuBisCO activation by RCA (modified from ref. Citation13). In this scheme, a RuBisCO active site that has spontaneously lost CO2 (was decarbamylated) binds a sugar phosphate RuBP, causing RuBisCO to undergo conformational changes leading to the inactivation of this protein. RCA interacts directly with the inactive RuBisCO, altering its conformation. ATP hydrolysis by RCA is required for these conformational changes. These conformational changes allow RuBP to dissociate and the CO2 molecule to enter the active RuBisCO site.

Mechanistically, RCA-driven release of the RuBisCO inhibition is based on the induction of ATP-dependent (RCA is a member of the AAA+ family of proteins, which are ATPases associated with diverse cellular activities) conformational changes that open closed RuBisCO sites, increase their accessibility to solvents, and facilitate dissociation of inhibitory sugar phosphates.Citation15 Transition of the RuBisCO structure from the closed to the open state involves rotation of the N-terminal domains that can proceed only in an RCA- RuBisCO super-complex comprising the RuBisCO holoenzyme with its 8 active sites encircled by 16 (or possibly 8) RCA subunits.Citation15 The torque needed to move the N-terminal domains of RuBisCO is provided by the concerted movement of the RCA subunits, the energy for which is provided by ATP hydrolysis.Citation15
The RCA activity is regulated by the ADP/ATP ratio in the stroma and by the ambient redox status.Citation8 In other words, the activity of RCA is principally dependent on the light intensity variation and the movement of protons into thylakoids that define the changes in the relative amounts of active and inactive forms of the enzyme. In addition, RCA activity can also be affected by high temperature, because it has been shown that RCA aggregates at high temperatures and can no longer activate RuBisCO diminishing carboxylation capacity.Citation16
Intrinsically disordered regions (IDRs) and RuBisCO activase (RCA)
Most of the eukaryotic proteins contain both structured and disordered regions. Intrinsically disordered regions (IDRs) are polypeptide segments that lack a defined 3D structure, existing as highly dynamic ensembles of flexible conformations,Citation17-24 and that have numerous important biological functions.Citation19,20,24-34 The high flexibility, decreased content of hydrophobic residues, and increased content of polar and charge residues (A, E, G, K, P, Q, R, and S) in the disordered regions allow them to interact with more than one partner and facilitate their multiple contacts. This ability to bind various partners makes intrinsically disorder proteins (IDPs) and IDRs important regulators capable of modulating the activity of several proteins in a coordinated way, playing an important role in various regulatory pathways.Citation23,35-38
The number of studies dedicated to the discovery and characterization of IDPs and IDRs has increased exponentially in the last years.Citation23 However, little is currently known about intrinsic disorder status of proteins in photosynthetic organism. The most recent studies of protein disorder in plants have shown that IDPs play central role in many responses such as stress (biotic and abiotic), development and metabolism regulation.Citation39-42 Yruela and Contreras-Moreira (2012) analyzed 12 plants genomes and concluded that the nuclear-encoded proteins follow the same trend that other multi-cellular eukaryotes and show a higher disorder in the internal part of nuclear-encoded proteomes rather than at their extremities, in contrast to the chloroplast- and mitochondrion- encoded proteomes.Citation43
RuBisCO activase (RCA) might be present in 2 different isoforms: α-isoform and β-isoform in photosynthetic organism,Citation44 but not all species appear to have both isoforms (e.g., Nicotiana tabacum has one RCA isoform).Citation45-47 shows low resolution 3D-structure of the Nicotiana tabacum RCA complex obtained by electron microscopy (PDB ID: 3ZW6).Citation48 The α-isoform differ from the β-isoform by the presence of a short C-terminal disordered extension that in most cases contains 2 cysteine residues, and is light dependent.Citation49 It was also pointed out that of these 2 isoforms, the longer RCA variant possesses higher ADP/ATP sensitivity,Citation50 but this high sensitivity is mostly eliminated via thioredoxin-f-induced reduction likely leading to the formation of a disulfide bridge between the C-terminal cysteine residues.Citation49 Therefore, although both RCA isoforms are capable of activating RuBisCO, only the large isoform is redox regulated.Citation49 This opens an intriguing possibility that changes in the expression pattern of RCAs (α-isoform vs. β-isoform) might represent a specific means to control and regulate the activity of activase. In other words, it is likely that the alternative splicing of RCA could serve as a means for the carbon assimilation optimization. Citation51 This hypothesis is partially supported by the results of a recent analysis of the relationships between RuBisCO activity, the expression of 2 RCA splicing variants, photoacclimation, and pre-hardening (15°C) and cold acclimation (4°C) of Lolium perenne genotypes with contrasting levels of freezing tolerance to low temperature.Citation51 This study revealed that only a highly freezing-tolerant genotype showed an induction of RuBisCO activity at both 15°C and 4°C. Curiously, noticeably enhanced RuBisCO activity after pre-hardening correlated with the increased expression of the long splicing variant (α-isoform) of RCA, whereas during cold acclimation, RuBisCO activity was increased due to the expression of both alternatively spliced variants.Citation51
Figure 4. Low resolution 3D-structure of the Nicotiana tabacum RCA complex obtained by the electron microscopy (PDB ID: 3ZW6).Citation48

Zhang et al. (2001) indicated that RCAs are functional heteromers (αnβn) and not homomers (αn or βn),Citation46 and Stotz et al. (2011) who worked with Nicotiana tabacum RCA found that the active state of this protein is a hexamer, where the helical insertion of the C-terminal is implicated in RuBisCO recognition and the Loop segments exposed toward the central pore of the hexamer required for the ATP-dependent remodeling of RuBisCO.Citation48 Based on this observations, Thieulin-Pardo et al. (2015) proposed a model for RCA in for A.thaliana.Citation13 They indicated that RCA is the α3β3 heterohexamers, and that one of its flat faces is involved in interaction with RuBisCO, whereas an exposed loop of the RuBisCO protein could fit into the central hole of RCA.Citation13
Motile microalgae have the ability to migrate vertically in the water column in order to exploit nutrients sources, to avoid predators, low and high irradiance, UV and energetic turbulence (temperature, sediments, etc.) near the surface.Citation52,53 The mobility allow them to adjust for optimal irradiance and maximize photosynthetic efficiency offering potential advantages over passive microalgae (non-motile) that are also exposed to all the aforementioned factors.Citation52 Therefore, motility in microalgae can be seen as an indirect mechanism that optimize irradiance condition for the photosynthesis. In this sense, non-motile microalgae can be more exposed to higher and lower levels of irradiance than the motile microalgae. It is likely that RuBisCo might be differently regulated in motile and non-motile algae. Although such regulation could act on many different levels (ranging from different expression of key metabolic enzymes (such as RuBisCo itself and other enzymes participating in Calvin cycle) to different regulation of transporters, etc.), we hypothesizes that the RCA, which is responsible for the RuBisCO activation through physical interaction with the disorder regions of this proteinaceous machine and whose activity is determined by the light irradiance (as well as ATP/ADP ratio and the ambient redox status), could be functionally different in the non-motile and motile microalgae. Therefore, there must be differences in the sequences, amino acid compositions, and content of functional intrinsic disorder in RCAs from these 2 microalgae.
Materials and methods
To test our hypothesis, 2 oceanic green microalgae were selected 2 species, Tetraselmis sp GSL018 and Ostreococcus tauri (O. tauri) that belong to the chlorophyta phylum. Tetraselmis sp. GSL018 is a eukaryotic unicellular flagellate microalgae that belongs to the Chlorodendraceae class, has 4 flagella, possesses phototaxis (light-dependent behavior, either toward the source of light or away from it),Citation54 and is of ecological and economic importance. On the other hand, O. tauri is the smallest unicellular non-motile eukaryotic microalgae known, and is the main component of the phytoplankton.Citation55 Aligned amino acid sequences of the corresponding RCA proteins are shown in .
Figure 5. Intrinsic disorder profiles of the RCA from O. tauri (A) and Tetraselmis sp. (B) generated by PONDR-FIT, PONDR® VLXT, PONDR® VSL2, and PONDR® VL3, IUPred_short, IUPred_long, DISOPRED3, and RONN v3.2. Bold dark pink dashed line shows the mean disorder propensity calculated by averaging disorder profiles of individual predictors. Light pink shadow around the PONDR® FIT curves shows error distribution. The amino acids in the input sequence are considered disordered when the corresponding disorder propensity curve is located above the 0.5 threshold.

Figure 6. (A) Aligned mean disorder profiles of the RCA proteins from O. tauri (solid black line) and Tetraselmis sp (dashed red line). Gaps in the corresponding curves correspond to the insertion found via ClustalW-based sequence alignment (see plot C). (B) Aligned ANCHOR profiles of the RCA proteins from O. tauri (solid black line) and Tetraselmis sp. (dashed red line). Corresponding outputs of DISOPRED3 for the position of disordered binding regions are shown at the top of the plot by light gray and pink curves for the O. tauri and Tetraselmis sp TCAs, respectively. (C) Sequence alignment of the RCAs from O. tauri (query sequence of 407 residues) and Tetraselmis sp. (subject sequence of 416 residues) by ClustalW2 (http://www.ebi.ac.uk/Tools/msa/clustalw2/). Although these 2 proteins show high overall sequence identity, the sequence similarity is low at their termini, where the IDRs are located. This is especially the case for the N-termini of these RCAs.

Intrinsic disorder propensities of target proteins were evaluated using 4 algorithms from the PONDR family, PONDR-FIT, PONDR® VLXT, PONDR® VSL2, and PONDR® VL3,Citation56-61 as well as the IUPred web server,Citation62 DISOPRED3,Citation63 and RONN v3.2.Citation64 For each protein, after obtaining an average disorder score by each predictor, all predictor-specific average scores were averaged again to generate an average per-protein intrinsic disorder score. Use of consensus for evaluation of intrinsic disorder is motivated by empirical observations that this approach usually increases the predictive performance compared to the use of a single predictor.Citation65-67
Potential disorder-based protein binding sites of query proteins (molecular recognition features, MoRFs) were identified by the ANCHOR algorithm.Citation68,69 This algorithm utilizes the pair-wise energy estimation approach originally used by IUPredCitation62,70 and acts on the hypothesis that long regions of disorder include localized potential binding sites which are not capable of folding on their own due to not being able to form enough favorable intrachain interactions, but can obtain the energy to stabilize via interaction with a globular protein partner.Citation68,69 We also used an SVM based classifier incorporated into the DISOPRED3 to identify IDRs that are predicted as protein binding sites based on their profile data and additional sequence-derived features.Citation63
Results and discussion
The molecular weights of the RCAs from O. tauri (407 amino acids) and Tetraselmis sp (416 amino acids) were 44.5 KDa and 45.3 kDa, respectively. RCAs are globular proteins that possess disordered tails.Citation13 Based on the results obtained by the sequences analysis using a wide range of disorder predictors (PONDR-FIT, PONDR® VLXT, PONDR® VSL2, and PONDR® VL3, IUPred_short, IUPred_long, DISOPRED3, and RONN v3.2) and the mean disorder propensity calculated by averaging disorder profiles of individual predictors, the 2 RCAs analyzed in our study are characterized by different disorder propensities (estimated as percentage of intrinsically disordered residues). In fact, shows that the percentage of intrinsically disordered residues in the sequence of the Tetraselmis sp RCA and its protein-average disorder scores are a bit higher than those of the O. tauri protein. This idea is further illustrated by that shows the aligned mean disorder profiles of these 2 proteins. In fact, based on the analysis of disorder profiles shown in , the Tetraselmis sp. RCA has 21.9% disordered residues and is characterized by the protein-average disorder score of 0.36±0.09, whereas the RCA from O. tauri contains 17.9% disordered residues and has the protein-average disorder score of 0.32 ± 0.08.
The intrinsic disorder profiles of RCA for O. tauri and Tetraselmis sp are shown in . Profiles clearly show that the RCA enzymes from both microalgae possess IDRs at their extremities, suggesting that the N- and C-termini of these proteins can be considered as disordered tails. Additionally, the RCA of Tetraselmis has a short disordered region at the central part of its sequence. For both RCAs, the C-terminal tails are noticeably shorter than corresponding N-tails. Since the C-terminal regions of these 2 RCAs do not have cysteine residues, these proteins cannot be considered as α-RCA isoforms, which, according Zhang and Portis classification,Citation49 should contain 2 cysteines within the C-termini. It is known that the functionality of both α- and β-isoforms of RCAs depends on the ADP/ATP ratio. However, it has been also demonstrated that α-RCAs is additionally dependent on the light because of the thioredoxin action (redox response) on its 2 cysteine residues present on its C-terminal. Curiously, not all species appear to have both isoforms, and only one RCA was found in tobacco, maize, and the fresh water microalgae Chlamydomonas.Citation45,46
represents results of the ClustalW-based sequence alignment of RCAs from O. tauri and Tetraselmis sp The sequence alignment showed a 68% identity, which indicates high structural and functional similarity of these proteins. However, despite this high overall identity levels, shows that the intrinsically disordered N- and C-termini of these proteins are characterized by low sequence similarity.
According to Thieulin-Pardo et al. (2015),Citation13 RCAs probably acquired their disorder regions during evolution to gain new regulation opportunities, such as enhanced responsiveness to the environmental factors and/or the ability to be engaged in new protein-protein interactions. In addition, a positive correlation has been found between the increase of disorder content and the increase in the biological complexity during evolution.Citation63,65,71-75 The microalgae O. tauri is the smallest and simplest photosynthetic eukaryotic organism described until now, which belongs to the earlier branch of the chlorophyte (Prasinophyceae), whereas Tetraselmis sp. belongs to the Chlorodendrophyceae branch. Therefore, it is possible that this differences in the total content of predicted intrinsic disorder in the RCAs of these microalgae and low sequence similarity of their N- and C-tails can be due to the evolutionary diversification of these proteins, leading to the development of different regulatory responses or different modes of protein-protein interactions.
To check this hypothesis, we evaluated the presence of disorder-based binding sites in the RCA proteins from 2 microalgae. Results of this analysis are shown in where the outputs of ANCHOR algorithm and functional annotation of DISOPRED3 are compared. According to ANCHOR, the O. tauri RCA has an N-terminally located 12 residue-long disorder-based binding site (residues 12-23) and a short binding site within the C-terminal tail (residues 385-386). The RCA from Tetraselmis sp has a short N-terminal binding site (residues 1-4) followed by 16 residue-long binding site (residues 18-33) and another short disorder-based binding site located at the 205-207 region (see ). DISOPRED3, which generates precise assignments of disordered protein binding regions,Citation63 indicated that both, the RCAs have 5 disordered binding regions, residues 1-23, 34-38, 45-58, 392-401, and 403-407 in the O. tauri protein and residues 3-5, 21-23, 245-250, 401-404, and 407-409 in the Tetraselmis sp. RCA.
Providing annotations of IDRs, secondary structure elements, and binding sites, DISOPRED3 gives a possibility to compare distribution of these features within the query proteins. shows the differences between the DISOPRED3 profiles of the RCA from both microalgae. Particularly, show that although the N- and C-tails of both RCAs contain disorder-based protein binding sites and although the overall level of predicted disorder is somewhat higher for the Tetraselmis RCA, the amount of binding-related disorder is higher for the O. tauri protein than for the RCA from Tetraselmis sp Comparing ANCHOR and DISOPRED data for these 2 proteins it is clear that in addition to the N- and C-terminal disorder-based binding sites the Tetraselmis protein has extra binding regions located within its central part. These differences in distribution of the disorder-based binding sites probably indicate that the ways in which the protein-protein interactions occur between the RCAs and their partners are different in different microalgae.
According to Parry et al.Citation8 the C-terminus of RCA is important for the RuBisCO recognition and for the ATP hydrolysis, whereas the N-terminus of this protein is necessary for RuBisCO activation.Citation8 However, the functional role and potential targets of the short disordered binding region at the central part of the Tetraselmis RCA are unknown as of yet. The presence of a remarkable sequence diversity in functional N- and C-terminal regions of these RCAs provides a support to the RuBisCO activation is carried out in different ways by these microalgae because the RCA binding disorder residues that interact with RuBisCO are not the same. Also, since C-tails of analyzed RCAs from both algae do not have characteristic cysteine residues, it is likely that these activases are not redox-sensitive.
Tetraselmis sp. has positive phototaxis (movement toward the light source) at lower light intensity and negative phototaxis (movement away from light source) at higher light intensity.Citation54 This kind of response is observed in Tetraselmis because it has a photoreceptor apparatus (stigma) that perceive a modulated light signal from the environment, which controls flagellar beat frequency and direction until the cell is aligned with the incoming light rays.Citation76 Tetraselmis motility and photosynthesis rate had shown to be conditioned by the quality and quantity of radiation (light intensity), so for this microalgae both process photosynthesis and motility are tightly coupled.Citation54 In other words, being a representative of the motile microalgae, Tetraselmis sp can actively search for the most optimal conditions of its growth, such as sufficient nutrients sources, optimal irradiance, temperature, etc. On the other hand, O. tauri is a passive (non-motile) microalgae and therefore has to use motility- and migration-unrelated means to adjust to changes in its environment. Therefore, it is expected that these 2 microalgae would utilize different approaches to maximize their photosynthetic efficiency. It is likely that one of the means to control the photosynthetic efficiency in motile Tetraselmis sp. is linked to its ability to actively migrate to the most optimal and “comfortable” environment. This, actually, decreases evolutionary pressure on the optimization of the RCA-driven regulation of RuBisCo activity (even a relatively lousy farmer can have great harvest if the soil is rich). On the contrary, the non-motile microalgae O. tauri likely to experience strong evolutionary pressure to optimize its RCA-RuBisCO system for maximal efficiency under the uncontrolled and likely non-optimized irradiance condition for the photosynthesis (if soil is poor, the ability to get a good harvest depends on specific skills of a farmer). In line with this hypothesis, we found several small, but important differences between the intrinsic disorder predisposition and presence and distribution disordered binding regions in RCAs from O. tauri and Tetraselmis sp Collectively, the differences in the total percentages of intrinsic disorder, in the presence of disordered biding and non-binding regions, and in the amino acid compositions of the IDRs of these 2 proteins, although been small, suggest that RCA, this important enzyme responsible for the RuBisCO activation in both microalgae, probably utilizes different modes of interaction with RuBisCO. This disorder-based functional difference is most likely due to the differences in the degree of variability in the irradiance conditions to which these microalgae are exposed as a consequence of their motility behavior.
Disclosure of potential conflicts of interest
No potential conflicts of interest were disclosed.
References
- Parlevliet D, Moheimani NR. Efficient conversion of solar energy to biomass and electricity. Aquat Biosyst 2014; 10:4; PMID:24976951; http://dx.doi.org/10.1186/2046-9063-10-4
- Andersson I, Backlund A. Structure and function of Rubisco. Plant Physiol Biochem 2008; 46:275-91; PMID:18294858; http://dx.doi.org/10.1016/j.plaphy.2008.01.001
- Bassham JA, Krause GH. Free Energy Changes and Metabolic Regulation in Steady-State Photosynthetic Carbon Reduction. Biochimica Et Biophysica Acta 1969; 189:207-&; PMID:5350447; http://dx.doi.org/10.1016/0005-2728(69)90048-6
- Dietz KJ, Heber U. Rate-Limiting Factors in Leaf Photosynthesis .1. Carbon Fluxes in the Calvin Cycle. Biochimica Et Biophysica Acta 1984; 767:432-43; http://dx.doi.org/10.1016/0005-2728(84)90041-0
- Woodrow I, Berry J. Enzymatic regulation of photosynthetic CO2 fixation in C3 plants. Annu Rev Plant Physiol 1988; 39:533-94; http://dx.doi.org/10.1146/annurev.pp.39.060188.002533
- Stitt M, Quick WP, Schurr U, Schulze ED, Rodermel SR, Bogorad L. Decreased ribulose-1,5-bisphosphate carboxylase-oxygenase in transgenic tobacco transformed with ‘antisense’ rbcS : II. Flux-control coefficients for photosynthesis in varying light, CO2, and air humidity. Planta 1991; 183:555-66; PMID:24193849
- Perchorowicz JT, Raynes DA, Jensen RG. Light limitation of photosynthesis and activation of ribulose bisphosphate carboxylase in wheat seedlings. Proc Natl Acad Sci U S A 1981; 78:2985-9; PMID:16593018; http://dx.doi.org/10.1073/pnas.78.5.2985
- Parry MA, Keys AJ, Madgwick PJ, Carmo-Silva AE, Andralojc PJ. Rubisco regulation: a role for inhibitors. J Exp Bot 2008; 59:1569-80; PMID:18436543; http://dx.doi.org/10.1093/jxb/ern084
- Salvucci ME, Portis AR, Jr., Ogren WL. A soluble chloroplast protein catalyzes ribulosebisphosphate carboxylase/oxygenase activation in vivo. Photosynth Res 1985; 7:193-201; PMID:24443088; http://dx.doi.org/10.1007/BF00037012
- Portis AR, Jr., Parry MA. Discoveries in Rubisco (Ribulose 1,5-bisphosphate carboxylase/oxygenase): a historical perspective. Photosynth Res 2007; 94:121-43; PMID:17665149; http://dx.doi.org/10.1007/s11120-007-9225-6
- Portis AR, Salvucci ME, Ogren WL. Activation of Ribulosebisphosphate Carboxylase/Oxygenase at Physiological CO(2) and Ribulosebisphosphate Concentrations by Rubisco Activase. Plant Physiol 1986; 82:967-71; PMID:16665175; http://dx.doi.org/10.1104/pp.82.4.967
- Beuf L, Kurano N, Miyachi S. Rubisco activase transcript (rca) abundance increases when the marine unicellular green alga Chlorococcum littorale is grown under high-CO2 stress. Plant Mol Biol 1999; 41:627-35; PMID:10645722; http://dx.doi.org/10.1023/A:1006371905597
- Thieulin-Pardo G, Avilan L, Kojadinovic M, Gontero B. Fairy “tails:” flexibility and function of intrinsically disordered extensions in the photosynthetic world. Front Mol Biosci 2015; 2:23; PMID:26042223; http://dx.doi.org/10.3389/fmolb.2015.00023
- Portis AR. The Regulation of Rubisco by Rubisco Activase. J Experimental Botany 1995; 46:1285-91; http://dx.doi.org/10.1093/jxb/46.special_issue.1285
- Portis AR, Jr. Rubisco activase - Rubisco's catalytic chaperone. Photosynth Res 2003; 75:11-27; PMID:16245090; http://dx.doi.org/10.1023/A:1022458108678
- Salvucci ME, Osteryoung KW, Crafts-Brandner SJ, Vierling E. Exceptional sensitivity of Rubisco activase to thermal denaturation in vitro and in vivo. Plant Physiol 2001; 127:1053-64; PMID:11706186; http://dx.doi.org/10.1104/pp.010357
- Dunker AK, Garner E, Guilliot S, Romero P, Albrecht K, Hart J, Obradovic Z, Kissinger C, Villafranca JE. Protein disorder and the evolution of molecular recognition: theory, predictions and observations. Pac Symp Biocomput 1998:473-84; PMID:9697205
- Wright PE, Dyson HJ. Intrinsically unstructured proteins: re-assessing the protein structure-function paradigm. J Mol Biol 1999; 293:321-31; PMID:10550212; http://dx.doi.org/10.1006/jmbi.1999.3110
- Uversky VN, Gillespie JR, Fink AL. Why are “natively unfolded“ proteins unstructured under physiologic conditions? Proteins 2000; 41:415-27; PMID:11025552; http://dx.doi.org/10.1002/1097-0134(20001115)41:3%3c415::AID-PROT130%3e3.0.CO;2-7
- Dunker AK, Lawson JD, Brown CJ, Williams RM, Romero P, Oh JS, Oldfield CJ, Campen AM, Ratliff CM, Hipps KW, et al. Intrinsically disordered protein. J Mol Graph Model 2001; 19:26-59; PMID:11381529; http://dx.doi.org/10.1016/S1093-3263(00)00138-8
- Tompa P. Intrinsically unstructured proteins. Trends Biochem Sci 2002; 27:527-33; PMID:12368089; http://dx.doi.org/10.1016/S0968-0004(02)02169-2
- Daughdrill GW, Pielak GJ, Uversky VN, Cortese MS, Dunker AK. Natively disordered proteins. In: Buchner J, Kiefhaber T, eds. Handbook of Protein Folding. Weinheim, Germany: Wiley-VCH, Verlag GmbH & Co. KGaA, 2005:271-353
- Uversky VN, Dunker AK. Understanding protein non-folding. Biochim Biophys Acta 2010; 1804:1231-64; PMID:20117254; http://dx.doi.org/10.1016/j.bbapap.2010.01.017
- Uversky VN, Dunker AK. The case for intrinsically disordered proteins playing contributory roles in molecular recognition without a stable 3D structure. F1000 biology reports 2013; 5:1; PMID:23361308; http://dx.doi.org/10.3410/B5-1
- Habchi J, Tompa P, Longhi S, Uversky VN. Introducing protein intrinsic disorder. Chem Rev 2014; 114:6561-88; PMID:24739139; http://dx.doi.org/10.1021/cr400514h
- van der Lee R, Buljan M, Lang B, Weatheritt RJ, Daughdrill GW, Dunker AK, Fuxreiter M, Gough J, Gsponer J, Jones DT, et al. Classification of intrinsically disordered regions and proteins. Chemical reviews 2014; 114:6589-631; PMID:24773235; http://dx.doi.org/10.1021/cr400525m
- Dunker AK, Brown CJ, Lawson JD, Iakoucheva LM, Obradovic Z. Intrinsic disorder and protein function. Biochemistry 2002; 41:6573-82; PMID:12022860; http://dx.doi.org/10.1021/bi012159+
- Dunker AK, Brown CJ, Obradovic Z. Identification and functions of usefully disordered proteins. Adv Protein Chem 2002; 62:25-49; PMID:12418100; http://dx.doi.org/10.1016/S0065-3233(02)62004-2
- Uversky VN. Natively unfolded proteins: a point where biology waits for physics. Protein Sci 2002; 11:739-56; PMID:11910019; http://dx.doi.org/10.1110/ps.4210102
- Uversky VN. What does it mean to be natively unfolded? Eur J Biochem 2002; 269:2-12; PMID:11784292; http://dx.doi.org/10.1046/j.0014-2956.2001.02649.x
- Uversky VN. Protein folding revisited. A polypeptide chain at the folding-misfolding-nonfolding cross-roads: which way to go? Cell Mol Life Sci 2003; 60:1852-71; PMID:14523548; http://dx.doi.org/10.1007/s00018-003-3096-6
- Uversky VN. A decade and a half of protein intrinsic disorder: biology still waits for physics. Protein Sci 2013; 22:693-724; PMID:23553817; http://dx.doi.org/10.1002/pro.2261
- Uversky VN. Functional roles of transiently and intrinsically disordered regions within proteins. FEBS J 2015; 282:1182-9; PMID:25631540; http://dx.doi.org/10.1111/febs.13202
- van der Lee R, Buljan M, Lang B, Weatheritt RJ, Daughdrill GW, Dunker AK, Fuxreiter M, Gough J, Gsponer J, Jones DT, et al. Classification of Intrinsically Disordered Regions and Proteins. Chem Rev 2014; 114:6589-631; PMID:24773235; http://dx.doi.org/10.1021/cr400525m
- Uversky VN. Intrinsic disorder-based protein interactions and their modulators. Curr Pharm Des 2013; 19:4191-213; PMID:23170892; http://dx.doi.org/10.2174/1381612811319230005
- Oldfield CJ, Meng J, Yang JY, Yang MQ, Uversky VN, Dunker AK. Flexible nets: disorder and induced fit in the associations of p53 and 14-3-3 with their partners. BMC Genomics 2008; 9 Suppl 1:S1; http://dx.doi.org/10.1186/1471-2164-9-S1-S1
- Dunker AK, Cortese MS, Romero P, Iakoucheva LM, Uversky VN. Flexible nets. The roles of intrinsic disorder in protein interaction networks. FEBS J 2005; 272:5129-48; PMID:16218947; http://dx.doi.org/10.1111/j.1742-4658.2005.04948.x
- Uversky VN, Oldfield CJ, Dunker AK. Showing your ID: intrinsic disorder as an ID for recognition, regulation and cell signaling. J Mol Recognit 2005; 18:343-84; PMID:16094605; http://dx.doi.org/10.1002/jmr.747
- Kragelund BB, Jensen MK, Skriver K. Order by disorder in plant signaling. Trends Plant Sci 2012; 17:625-32; PMID:22819467; http://dx.doi.org/10.1016/j.tplants.2012.06.010
- Sun XL, Rikkerink EHA, Jones WT, Uversky VN. Multifarious Roles of Intrinsic Disorder in Proteins Illustrate Its Broad Impact on Plant Biology. Plant Cell 2013; 25:38-55; PMID:23362206; http://dx.doi.org/10.1105/tpc.112.106062
- Marin M, Ott T. Intrinsic disorder in plant proteins and phytopathogenic bacterial effectors. Chem Rev 2014; 114:6912-32; PMID:24697726; http://dx.doi.org/10.1021/cr400488d
- Yruela I, Contreras-Moreira B. Genetic recombination is associated with intrinsic disorder in plant proteomes. BMC Genomics 2013; 14:772; PMID:24206529; http://dx.doi.org/10.1186/1471-2164-14-772
- Yruela I, Contreras-Moreira B. Protein disorder in plants: a view from the chloroplast. BMC Plant Biol 2012; 12:165; PMID:22970728; http://dx.doi.org/10.1186/1471-2229-12-165
- Gontero B, Salvucci ME. Regulation of photosynthetic carbon metabolism in aquatic and terrestrial organisms by Rubisco activase, redox-modulation and CP12. Aquatic Botany 2014; 118:14-23; http://dx.doi.org/10.1016/j.aquabot.2014.05.011
- Salvucci ME, Werneke JM, Ogren WL, Portis AR. Purification and species distribution of rubisco activase. Plant Physiol 1987; 84:930-6; PMID:16665546; http://dx.doi.org/10.1104/pp.84.3.930
- Zhang N, Schurmann P, Portis AR, Jr. Characterization of the regulatory function of the 46-kDa isoform of Rubisco activase from Arabidopsis. Photosynth Res 2001; 68:29-37; PMID:16228326; http://dx.doi.org/10.1023/A:1011845506196
- Rokka A, Zhang L, Aro EM. Rubisco activase: an enzyme with a temperature-dependent dual function? Plant J 2001; 25:463-71; PMID:11260502; http://dx.doi.org/10.1046/j.1365-313x.2001.00981.x
- Stotz M, Mueller-Cajar O, Ciniawsky S, Wendler P, Hartl FU, Bracher A, Hayer-Hartl M. Structure of green-type Rubisco activase from tobacco. Nat Struct Mol Biol 2011; 18:1366-U78; PMID:22056769; http://dx.doi.org/10.1038/nsmb.2171
- Zhang N, Portis AR, Jr. Mechanism of light regulation of Rubisco: a specific role for the larger Rubisco activase isoform involving reductive activation by thioredoxin-f. Proc Natl Acad Sci U S A 1999; 96:9438-43; PMID:10430961; http://dx.doi.org/10.1073/pnas.96.16.9438
- Shen JB, Orozco EM, Jr., Ogren WL. Expression of the two isoforms of spinach ribulose 1,5-bisphosphate carboxylase activase and essentiality of the conserved lysine in the consensus nucleotide-binding domain. J Biol Chem 1991; 266:8963-8; PMID:1827441
- Jurczyk B, Pociecha E, Grzesiak M, Kalita K, Rapacz M. Enhanced expression of Rubisco activase splicing variants differentially affects Rubisco activity during low temperature treatment in Lolium perenne. J Plant Physiol 2016; 198:49-55; PMID:27152456; http://dx.doi.org/10.1016/j.jplph.2016.03.021
- Raven JA. The flagellate condition. In: Leadbeater BSC, Green JC, eds. Flagellates: Unity, Diversity and Evolution. London and New York: Taylor & Francis, 2003:27-48
- Ralston DK, McGillicuddy DJ, Townsend DW. Asynchronous vertical migration and bimodal distribution of motile phytoplankton. J Plankton Res 2007; 29:803-21; http://dx.doi.org/10.1093/plankt/fbm061
- Ma ZL, Helbling EW, Li W, Villafane VE, Gao KS. Motility and photosynthetic responses of the green microalga Tetraselmis subcordiformis to visible and UV light levels. J Appl Phycol 2012; 24:1613-21; http://dx.doi.org/10.1007/s10811-012-9822-4
- Chretiennotdinet MJ, Courties C, Vaquer A, Neveux J, Claustre H, Lautier J, Machado MC. A New Marine Picoeucaryote - Ostreococcus Tauri Gen Et Sp-Nov (Chlorophyta, Prasinophyceae). Phycologia 1995; 34:285-92; http://dx.doi.org/10.2216/i0031-8884-34-4-285.1
- Romero P, Obradovic Z, Li X, Garner EC, Brown CJ, Dunker AK. Sequence complexity of disordered protein. Proteins 2001; 42:38-48; PMID:11093259; http://dx.doi.org/10.1002/1097-0134(20010101)42:1%3c38::AID-PROT50%3e3.0.CO;2-3
- Li X, Romero P, Rani M, Dunker AK, Obradovic Z. Predicting Protein Disorder for N-, C-, and Internal Regions. Genome Inform Ser Workshop Genome Inform 1999; 10:30-40; PMID:11072340
- Xue B, Dunbrack RL, Williams RW, Dunker AK, Uversky VN. PONDR-FIT: a meta-predictor of intrinsically disordered amino acids. Biochim Biophys Acta 2010; 1804:996-1010; PMID:20100603; http://dx.doi.org/10.1016/j.bbapap.2010.01.011
- Peng K, Vucetic S, Radivojac P, Brown CJ, Dunker AK, Obradovic Z. Optimizing long intrinsic disorder predictors with protein evolutionary information. J Bioinform Comput Biol 2005; 3:35-60; PMID:15751111; http://dx.doi.org/10.1142/S0219720005000886
- Obradovic Z, Peng K, Vucetic S, Radivojac P, Dunker AK. Exploiting heterogeneous sequence properties improves prediction of protein disorder. Proteins 2005; 61 Suppl 7:176-82; http://dx.doi.org/10.1002/prot.20735
- Peng K, Radivojac P, Vucetic S, Dunker AK, Obradovic Z. Length-dependent prediction of protein intrinsic disorder. BMC Bioinformatics 2006; 7:208; PMID:16618368; http://dx.doi.org/10.1186/1471-2105-7-208
- Dosztanyi Z, Csizmok V, Tompa P, Simon I. IUPred: web server for the prediction of intrinsically unstructured regions of proteins based on estimated energy content. Bioinformatics 2005; 21:3433-4; PMID:15955779; http://dx.doi.org/10.1093/bioinformatics/bti541
- Ward JJ, Sodhi JS, McGuffin LJ, Buxton BF, Jones DT. Prediction and functional analysis of native disorder in proteins from the three kingdoms of life. J Mol Biol 2004; 337:635-45; PMID:15019783; http://dx.doi.org/10.1016/j.jmb.2004.02.002
- Yang ZR, Thomson R, McNeil P, Esnouf RM. RONN: the bio-basis function neural network technique applied to the detection of natively disordered regions in proteins. Bioinformatics 2005; 21:3369-76; PMID:15947016; http://dx.doi.org/10.1093/bioinformatics/bti534
- Walsh I, Giollo M, Di Domenico T, Ferrari C, Zimmermann O, Tosatto SC. Comprehensive large-scale assessment of intrinsic protein disorder. Bioinformatics 2015; 31:201-8; PMID:25246432; http://dx.doi.org/10.1093/bioinformatics/btu625
- Fan X, Kurgan L. Accurate prediction of disorder in protein chains with a comprehensive and empirically designed consensus. J Biomol Struct Dyn 2014; 32:448-64; PMID:23534882; http://dx.doi.org/10.1080/07391102.2013.775969
- Peng Z, Kurgan L. On the complementarity of the consensus-based disorder prediction. Pac Symp Biocomput 2012:176-87; PMID:22174273
- Meszaros B, Simon I, Dosztanyi Z. Prediction of protein binding regions in disordered proteins. PLoS Comput Biol 2009; 5:e1000376; PMID:19412530
- Dosztanyi Z, Meszaros B, Simon I. ANCHOR: web server for predicting protein binding regions in disordered proteins. Bioinformatics 2009; 25:2745-6; PMID:19717576; http://dx.doi.org/10.1093/bioinformatics/btp518
- Dosztanyi Z, Csizmok V, Tompa P, Simon I. The pairwise energy content estimated from amino acid composition discriminates between folded and intrinsically unstructured proteins. J Mol Biol 2005; 347:827-39; PMID:15769473; http://dx.doi.org/10.1016/j.jmb.2005.01.071
- Dunker AK, Obradovic Z, Romero P, Garner EC, Brown CJ. Intrinsic protein disorder in complete genomes. Genome Inform Ser Workshop Genome Inform 2000; 11:161-71; PMID:11700597
- Schad E, Tompa P, Hegyi H. The relationship between proteome size, structural disorder and organism complexity. Genome Biol 2011; 12:R120; PMID:22182830; http://dx.doi.org/10.1186/gb-2011-12-12-r120
- Xue B, Dunker AK, Uversky VN. Orderly order in protein intrinsic disorder distribution: disorder in 3500 proteomes from viruses and the three domains of life. J Biomol Struct Dyn 2012; 30:137-49; PMID:22702725; http://dx.doi.org/10.1080/07391102.2012.675145
- Peng Z, Yan J, Fan X, Mizianty MJ, Xue B, Wang K, Hu G, Uversky VN, Kurgan L. Exceptionally abundant exceptions: comprehensive characterization of intrinsic disorder in all domains of life. Cell Mol Life Sci 2015; 72:137-51; PMID:24939692; http://dx.doi.org/10.1007/s00018-014-1661-9
- Necci M, Piovesan D, Tosatto SC. Large-scale analysis of intrinsic disorder flavors and associated functions in the protein sequence universe. Protein Sci 2016; PMID:27636733; http://dx.doi.org/10.1002/pro.3041
- Häder DP, Hemmersbach R, Lebert M. Gravity and the Behavior of Unicellular Organisms. New York: Cambrige University Press, 2005