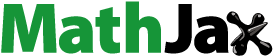
Abstract
Reactors produce noise that negatively impacts the environment during their operation. This study focused on vibration and noise reduction in shunt oil reactors through an enhanced design, aiming to mitigate these environmental consequences. Additionally, the precision of measuring vibroacoustic characteristics was enhanced by employing the finite element method. This study utilized the 110–750 kV reactor, POM-60000/525-У1. To minimize noise and vibrations from the active part to the tank, the rubber gaskets’ thicknesses, dimensions, and placements were carefully chosen, considering the magnetic system’s design specifics and pressing forces within the reactor frame. The reactor’s design underwent improvements through modeling and optimization. In the revised design, the number of radiators in the cooling system on both sides and the stiffeners on the reactor tank’s walls were increased, while the reactor’s overall dimensions were preserved. The isosurfaces derived from vibroacoustic testing on the reactor tank model facilitated the processing and visualization of results, thereby validating the model’s accuracy and effectiveness. The average background noise levels of the enhanced reactor were significantly lowered, with the noise difference between the reactor and its surroundings being nearly double that of its existing counterpart. Since noise from wall vibrations and the cooling system impacts reactor lifecycles, reducing these vibrations is crucial for extending the lifespan of the improved reactor.
REVIEWING EDITOR:
1. Introduction
In power grids, shunt oil reactors play a crucial role in enhancing the capacities of ultra-high-voltage lines, mitigating reactive power in voltage lines, and stabilizing both reactive power and voltage levels (Belyaev et al., Citation2005; Meiying, Citation2019; Yinbiao et al., Citation2018). Reactive power flow within electrical networks is a dynamic process, oscillating between consumption and generation. An excess of reactive power can lead to heightened active losses and an increase in grid frequency (Bengtsson et al., Citation2020; Sun et al., Citation2017). Load variations alter the system’s characteristics, modifying the flow of reactive current. This flow induces a voltage drop, lowering the system voltage below its no-load value, and results in additional network losses. To address fluctuations in reactive power demand, stemming from changes in power consumption or generation, systems often undergo adjustments or compensations. These measures ensure that operations continue within defined and acceptable parameters (Aali, Citation2017).
In networks characterized by predictable and stable power consumption and generation, capacitor banks or fixed-power shunt reactors are employed to manage reactive power. This compensation occurs under specific operating conditions to maintain system efficiency. Introducing mechanical switching to these units can further enhance their operational flexibility. Shunt reactors play a pivotal role in stabilizing voltage levels, ensuring fluctuations remain within a 2% threshold. Additionally, they contribute to a reduction in power losses, typically between 15 and 20%, thereby bolstering system stability and overall capacity (Tong et al., Citation2017).
During operation, transformer-reactor equipment generates noise, which in turn has adverse effects on the environment. The noise in reactors originates from the vibration of the active components and the cooling system’s fans. Concurrently, the equipment’s vibration, the primary source of this noise, compromises its mechanical integrity, shortening its lifespan and elevating the risk of internal damage. Notably, DC control windings, employed to maintain a constant magnetic bias flux, significantly contribute to vibration and noise by altering the core’s magnetic saturation level (Shuai et al., Citation2015; Zhang et al., Citation2019).
Magnetostriction in siliceous steel, which causes deformation of the crystal lattice in magnetic materials during magnetization, along with electromagnetic forces between the discs in the active zone and dynamic forces within the windings, are primary factors contributing to reactor vibration (Chen et al., Citation2019; Gao et al., Citation2009; Yuan et al., Citation2022). Consequently, there is an increasing focus on processes aimed at minimizing the environmental impact of power equipment utilized in the distribution and transmission of electrical energy. The continuous vibration of the reactor core primarily accelerates the wear of insulating components, necessitating frequent maintenance and replacement of these parts in the unit (Isaac et al., Citation2021; Wrona et al., Citation2022). Presently, transformers within the 110–750 kV range emit noise levels from 79 dB(A) to just above 90 dB(A). However, health standards dictate that sustained noise exposure should not exceed 74 dB(A) during the day and 59 dB(A) at night to avoid auditory harm (Beltle & Tenbohlen, Citation2016; Mazur et al., Citation2021; Murat et al., Citation2020). Recent advancements have seen the finite element method applied in computer simulations to design and analyze shunt oil reactors (Tu et al., Citation2021). This study aims to diminish noise and vibration in reactors of the 110–750 kV class through design enhancements of the POM-60000/525-У1 shunt oil reactor, thereby improving its noise and vibration characteristics and extending its operational lifespan.
2. Materials and methods
This study examined the POM-60000/525-У1 reactor, known for its improved vibration and noise characteristics, with the main technical data presented in . At the design stage, we used TDS, a 3D design, computer modeling, and visualization program developed by the Soft Team Group.Footnote1 In the TDS environment, the reactor’s geometric model featured a spatial shell with stiffeners (). The geometric model of the tank is depicted in .
Table 1. Main technical data related to POM-60000/525-У1.
displays the dimensional parameters obtained in TDS and the three-dimensional (3D) model of the improved РОМ-60000/525 design. Using the finite element method in TDS, we simulated and optimized the tank design to lower the displacement amplitudes of its walls, consequently reducing reactor vibrations. Vibration analysis in TDS calculates the tank walls’ bending amplitudes at various frequencies due to a harmonic load. The tank’s geometric model included a spatial shell with stiffeners, and a 3D acoustic environment model was built inside, transmitting sound pressure to the tank walls through this oil. The tank’s geometric model included a spatial shell with stiffeners, and a 3D acoustic environment model was built inside, transmitting sound pressure to the tank walls through the oil. The model focused solely on sound pressure and its transmission through the oil to the tank walls.
The target sound power level was set at 96 dB(A), with a 19.1 dB transition adjustment from the corrected to the uncorrected A-scale. To simulate the active part’s vibration load, acoustic pressure was applied across the tank’s inner surface. The calculated sound pressure and power are listed in .
Table 2. Design sound pressure and power.
The design of the POM-60000/525 tank was improved as follows:
The number of ‘M’-type radiators in the cooling system of the reactor was increased from six to seven on both sides.
The number of vertical and horizontal stiffeners on the wall of the reactor tank was increased to 15 and 7, respectively.
Laser-treated M090-27Pb HIB laser-grade electric steel with low vibroacoustic characteristics was used for manufacturing the magnetic circuit in the active part of the reactor. Laser treatment of the metal surface in the final stage of production can reduce specific magnetic losses by 8–10%. In transformer cores made of anisotropic electrical steel, eddy current losses account for up to 70% of the total remagnetization losses. Eddy current losses can be reduced via several methods, such as obtaining a material with adjustable grain size, artificially narrowing 180° domains by mechanically scratching the surface of finished sheets, and surface treatment with pulsed or continuous CO2 laser radiations. Surface treatment of electrical steel using continuous CO2 laser radiations does not break the insulation coating or generate additional mechanical stresses (Maharjan et al., Citation2019). The selected 0.27-mm-thick cold rolled grain-oriented steel possessed the following guaranteed magnetic properties: maximum specific loss at 1.7 T and 50 Hz was 0.90 W/kg and the polarization at H = 8004/M was J = 1.88 T. The design characteristics and dimensions of the reactor are listed in .
Table 3. Design characteristics and dimensions of the POM-60000/525-U1 reactor.
The saturation curve of the material is shown in . As shown in , the dependence of the peak values of flow coupling on the peak values of current is almost linear. Experimentally, the noise and vibration levels transmitted from the active part to the reactor tank were found to be reduced by 2–3 dB when the magnetic circuit was manufactured using M090-27Pb HIB laser electrical steel with rubber gaskets () and transition disks with diameters of 800 and 1205 mm in the reactor core and the central core of the magnetic circuit.
Figure 4. Installation of rubber gaskets on the 3D model of the reactor: (a) 1—between the packages of the magnetic system and (b) 2—between the beam and the yoke on the assembled reactor.

The reactor design contained a central ferromagnetic rod with nonmagnetic gaps evenly distributed along the length of the rod, which reduced the main losses owing to the reduction in the length of the magnetic field line in the non-magnetic medium. The use of adapter discs in these gaps can reduce noise levels.
According to thermal calculations, the maximum temperature of the surface of the magnetic system should be no more than 75 °C above the ambient temperature. Nitrile rubber has excellent oil resistance at high temperatures up to 300 °C and has gasoline resistance. The dimensions and position of the rubber gaskets were selected based on the design features of the reactor frame and the pressing forces of the magnetic system of this frame.
Simulations revealed that dividing the active area of steel into small areas, which increases the damping effect, can reduce noise and vibrations via the installed rubber dampers made of nitrile butadiene rubber gaskets. When nitrile rubber is used, each section of the magnetic system is grounded according to the scheme shown in .
The following areas were included in the present study:
Comparative analysis of the vibration and noise characteristics of the new shunt oil reactor with an improved design and that of the operating analog, РОМБСМ-60000/500У.
Verification of the adequacy of the model developed using the TDS program based on the finite element method for calculating vibration displacements of reactor tank walls.
3. Results and discussion
3.1. Conducting noise tests
РОМ-60000/525-У1 has a cooling system of the ‘M (ONAN)’ type consisting of two groups of seven radiators each. shows the points at which sound levels are measured in the reactor with the cooling system.
Figure 6. Points at which the sound levels of the reactor are measured: A—high-voltage lead and X1, X2—grounded side.

The test reactor was installed on a stand without rollers, the nominal excitation voltage was 303.11 kV, the height of the reactor tank was 3190 mm, the total area of the test room was 891 m2, the measuring line perimeter related to the measurement distance L = 2.0 m was 34 m. Sound levels were measured using a handheld analyzer (model B&K 2250 L-100) ().
The measurement lines were placed 0.3 m from the radiating surface of the reactor at 1/3 and 2/3 of the reactor height. A microphone was installed at each measurement point in the direction of the test objects. Before the tests, the noise meter was acoustically calibrated, and the extraneous noise levels were measured. and summarize the measured sound data.
Table 4. Measured extraneous noise levels.
Table 5. Measured sound levels.
The measurement results were processed, and the corrected sound power level was calculated according to IEC 60076-6-2014. The constant sound reflection coefficient K (dBA) of the room in which the tests were performed was calculated as follows:
(1)
(1)
where S is the area of the measuring surface. Additionally, А is the equivalent area over which sound is absorbed in the room and is approximately calculated using the following formula:
(2)
(2)
where а is the average sound absorption coefficient equal to 0.15 (IEC 60076-10-1:2016) and
is the total area of the test room (m2).
Furthermore, S is the area of the surface over which the radiation of the reactor was measured.
(3)
(3)
where H is the height of the tank and L is the length of the measuring line perimeter.
Calculated constant K, which considers the influence of the reflected sound, was
The calculated average sound level of the reactor with the cooling system was
(4)
(4)
Corrected sound power level LpA was
(5)
(5)
Therefore, the corrected sound power level of the POM-60000/525-У1 kV reactor was 82.2 dBA with a permissible value of 100 dBA based on IEC 60076-6-2014. compares the calculated noise characteristics of the РОМ-60000/525-У1 reactor and those of its analog, РОМБСМ-60000/500-У1, obtained from acceptance tests https://drive.google.com/drive/folders/1pONbTSHgscVpXBTDl5BFjm8VyrWV6Vwr?usp=sharing
Table 6. Comparison between the calculated noise characteristics of РОМ-60000/525-У1 and those of РОМБСМ-60000/500-У1.
The analysis of the collected data shows a marked decrease in the average background noise levels for the improved reactor, РОМ-60000/525-У1, with the noise disparity between the reactor and its ambient environment being roughly double that of its predecessor, РОМБСМ-60000/500-У1. The wear on the insulating components within the reactor’s magnetic system is primarily due to noise and vibration from the magnetic system’s operation, a phenomenon largely attributed to magnetostriction. Specifically, the insulation between the magnetic system and the pressing yoke beams, as well as the insulation between the magnetic system and the fixing brackets, tends to degrade first. Moreover, the end beams and foot, along with the cardboard cooling rails situated between the electrical steel package layers in the magnetic system, are among the first components to show signs of wear. Consequently, these parts often require replacement during comprehensive maintenance activities. Given that reactor noise—which significantly influences its lifespan—is linked to the vibrations of the reactor walls and the cooling system, it is anticipated that the lifespan of the enhanced reactor, РOM-60000/525-У1, will surpass that of its predecessor.
3.2. Conducting vibration tests
Vibration displacements for both the tank wall and the cooling system were assessed on a fully assembled РOM-60000/525 shunt reactor (). This reactor was securely positioned on a stand devoid of rollers, with an applied excitation voltage of 303.11 kV. The testing protocol adhered to the standards outlined in IEC 60068-2-80:2005. For these measurements, VIBROTEST MG-4 measuring instrument was employed. The specific locations where these vibration displacements were recorded are depicted in . The measured vibration displacements at the designated points are detailed in and . The peak vibration displacement observed on the tank wall reached 21.9 µm, remaining within the acceptable limit of 30 µm as stipulated by IEC 60076-6-2014. In the case of the cooling system, the maximum displacement recorded was 12.15 µm, well below the allowable threshold of 60 µm. Consequently, the РОМ-60000/525-У1 reactor successfully met the vibroacoustic testing standards and complied with the criteria set forth in IEC 60068-2-80:2005.
Figure 8. Process of measuring vibration displacements of the tank wall and the cooling system of РOM-60000/525-У1.

Figure 9. Points at which vibration displacements are measured on the tank wall (1–12) and the reactor cooling system (1–14).

Table 7. Measured vibration displacements of the tank wall.
Table 8. Measured vibration displacements on the cooling system.
3.3. Calculated and modeled vibration displacements of the tank walls using the finite element method
Using the finite element method within the TDS program facilitated the estimation and representation of reactor tank wall displacements through isosurfaces. Should the outcomes from the model align with those from vibroacoustic testing, it would confirm the model’s accuracy.
The vibration analysis encompassed the bending amplitudes of the tank walls across various frequencies under harmonic loading. In constructing the geometrical model, intricate details, such as the active part’s design—including the yoke, windings, disks, and studs—along with the positioning of the support points at the tank’s base, were meticulously incorporated. The computational framework within the TDS program employed specific modules: SHELL181 for shell structures, SOLID185 for solid elements, and PIPE59 for pipe elements. The inclusion of contact elements, namely TARGE170 and CONTA174, facilitated the simulation of interactions within the model.
Inside the tank, a volumetric model of the acoustic medium, i.e. oil, through which the sound pressure was transmitted to the tank walls was constructed. This model was divided into two volumes. The first was directly in contact with the tank wall, and the second, the internal volume, was in contact with the active part. This calculation scheme considered only the sound pressure and the transmission of this pressure through the oil to the tank walls. The calculated sound power level was 96 dB(A). The transition correction from the corrected-A scale to the uncorrected scale was 19.1 dB. The acoustic pressure applied to the inner surface of the tank was modeled as the vibration load generated by the active part. The calculated load was 364 Pa. The frequency interval was assumed to be 50–150 Hz. The relative damping coefficient was assumed to be 0.02 (2%). The model was fixed at the locations where the bottom beams rested on the wheels.
The calculation results were presented in the form of isosurfaces on the geometric model using a given scale, depicting the mutual correspondence between color and numerical data. shows the calculated total vibration displacements of the entire tank at 100 Hz according to the IEC 60068-2-80:2005 test method.
Figure 10. Vibration displacements (mm) of the tank walls at 100 Hz. (a) Calculation results and (b) 3D model of the tank.

The analysis revealed that the tank walls experienced their maximum displacement at the base, measuring 0.0162 mm, or 16.2 µm, as depicted in . Displacement measurements for the tank’s four sides were conducted at 100 Hz along each coordinate axis. For every side of the tank, displacement curves were plotted at points corresponding to the X and Z coordinate axis directions, within a frequency range of 50–150 Hz. Specifically, illustrates the HV (‘−Z’) side of the РОМ-60000/525-У1 reactor tank, where the displacement peaked at 0.01052 mm or 10.52 µm.
Figure 11. Vibration displacements of the tank at a frequency of 100 Hz (mm) on the HV (‘−Z’) side. (a) Calculation results and (b) location of selected points 10–15.

show the displacement plots (mm) in the frequency range of 50–150 Hz obtained from points 10–12 and 13–15, respectively, along the z-axis. The maximum displacement of the tank walls at a frequency of 100 Hz was observed at the center of the longitudinal walls (points 10 and 14 in , respectively). This displacement was 0.0105 mm = 10.5 µm, and the maximum double value of displacement was 21 µm. The maximum average value of the vibration displacements of the tank walls was measured using a hand-held device (VIBROTEST MG-4) and was found to be 21.9 μm.
Figure 12. Displacement plots (mm) in the frequency range of 50–150 Hz were obtained from (a) points 10–12 and (b) 13–15 along the z-axis.

Thus, the model created with the TDS program, which was employed for computing the vibration displacements of the reactor tank walls using the finite element method, proved to be adequate. The discrepancy in the measured maximum displacement was found to be 4.3%.
4. Conclusion
Using the finite element method in the TDS environment, the РOM-60000/525 reactor was modeled and optimized to lower tank wall displacement amplitudes and reactor vibration levels. This led to the following reactor design improvements. The number of ‘M’-type radiators in the cooling system was increased from six to seven on both sides. Additionally, the number of vertical and horizontal stiffeners on the reactor tank wall was increased to 15 and 7, respectively.
To further reduce vibration, the reactor’s magnetic circuit utilized laser-treated M090-27Pb HIB laser-grade electric steel, and nitrile butadiene rubber was chosen for the damping gaskets. Furthermore, in the improved РOM-60000/525-У1 reactor, transition disks of 800 and 1205 mm diameters were employed in the magnetic system’s central core. The innovations halved the noise difference between the reactor and background, compared to the existing РОМБСМ-60000/500-У1 model. Since reactor noise from wall and cooling system vibrations significantly impacts its lifecycle, these improvements extended the lifespan of the РOM-60000/525-У1 reactor.
This study’s key contribution is the design enhancement of the POM-60000/525-U1 shunt oil reactor, reducing noise and vibrations for 110–750 kV reactors and extending their service life. The TDS-developed model’s adequacy for calculating reactor tank wall vibration displacements was confirmed, with a maximum displacement measurement error of 4.3% by the VIBROTEST MG-4 device.
Acknowledgments
The study was conducted in “TRENCO R&D” LLP, 010000, Astana city, Kabanbay Batyr Ave., Building 53/1, Block 53 (S4).
Disclosure statement
No potential conflict of interest was reported by the author(s).
Additional information
Funding
Notes on contributors

Tazhibayev Adіlbek
Tazhibayev Adilbek has worked as a Chief Specialist at a transformer plant for more than ten years. He is an author and a leader of a team that developed a line of 35, 110, 220, and 500 kV electrical equipment. These pieces of equipment are manufactured and operated in several countries. During development, modern calculation methods were used and new scientific results and patents were obtained to improve the design and quality of these equipment.
Utepbergenov Irbulat
Utepbergenov Irbulat Doctor of Technical Sciences, Professor, Head of Laboratory of Cyber-physical Systems and Smart technologies Almaty University of Power Engineering and Telecommunications named after Gumarbek Daukeyev. Research interests: ACS, Information technology in education, telecommunications, control and transport.
Amіtov Yernar
Amitov Yernar received his PhD from Tomsk Polytechnic University in 2018. His research interests include the study of the electrical properties of composite polymer dielectrics and the physics of dielectrics. Currently, he works as the head of the Department of “Electrical Engineering” of the Almaty University of Power Engineering and Telecommunications named after Gumarbek Daukeyev.
Ateyev Dauirbek
Ateyev Dauirbek received a bachelor’s degree in electrical power engineering from Almaty University of Energy and Communications in 2015. His research interests include relay protection and automation, power supply of industrial enterprises. Currently, he works as Deputy Chairman of the Board of Asia Trafo LLP, a plant producing transformers for voltages of 110, 220 and 500 kV with a capacity of up to 400 MVA.
Notes
References
- Aali, S. (2017). Shunt active DC filter based on intelligence controller for HVDC link. High Voltage, 2(4), 274–279. https://doi.org/10.1049/hve.2016.0026
- Beltle, M., & Tenbohlen, S. (2016). Power transformer diagnosis based on mechanical oscillations due to AC and DC currents. IEEE Transactions on Dielectrics and Electrical Insulation, 23(3), 1515–1522. https://doi.org/10.1109/TDEI.2016.005537
- Belyaev, A. N., & Smolovik, S. (2005). Steady-state and transient stability of 500kV long-distance AC transmission lines with magnetically controlled shunt reactors. IEEE Power Tech Conference.
- Bengtsson, C. T., & Kern, A. J. (2020). Shunt reactors improve voltage stability and power quality. Transformers Magazine, 7, 178–182.
- Chen, D., Hou, B., Feng, Z., & Bai, B. (2019). Study of magnetostrictive influence of electrical sheet steel under different DC biases. IEEE Transactions on Magnetics, 55(2), 1–5. https://doi.org/10.1109/TMAG.2018.2873785
- Gao, Y., Muramatsu, K., Fujiwara, K., Ishihara, Y., Fukuchi, Sh., & Takahata, T. (2009). Vibration analysis of a reactor driven by an inverter power supply considering electromagnetism and magnetostriction. IEEE Transaction on Magnetics, 45(10), 4789–4792.
- Hyperlink to supporting test documents. (n.d.). https://drive.google.com/drive/folders/1pONbTSHgscVpXBTDl5BFjm8VyrWV6Vwr?usp=sharing
- Isaac, C. W., Wrona, S., Pawelczyk, M., & Roozen, N. B. (2021). Numerical investigation of the vibro-acoustic response of functionally graded lightweight square panel at low and mid-frequency regions. Composite Structures, 259, 113460. https://doi.org/10.1016/j.compstruct.2020.113460
- Maharjan, N., Zhou, W., Zhou, Y., Guan, Y., & Wu, N. (2019). Comparative study of laser surface hardening of 50CrMo4 steel using continuous-wave laser and pulsed lasers with ms, ns, ps and fs pulse duration. Surface and Coatings Technology, 366, 311–320. https://doi.org/10.1016/j.surfcoat.2019.03.036
- Mazur, K., Rzepecki, J., Pietruszewska, A., Wrona, S., & Pawelczyk, M. (2021). Vibroacoustical performance analysis of a rigid device casing with piezoelectric shunt damping. Sensors, 21(7), 2517. https://doi.org/10.3390/s21072517
- Meiying, Z. (2019). Mechanism and problem analysis of high-voltage shunt reactor. AIP Conference Proceedings 2066 (pp. 1–5).
- Murat, A., Sokolov, S., Saukhimov, A., & Dolgopolov, A. (2020). Research and modeling of magnetically controlled shunt reactors in PSCAD/EMTDC. Proceedings of the 7th International Conference of Electrical and Electronics Engineering (ICEEE) (pp. 155–159).
- Shuai, P., & Biela, J. (2015). Impact of core shape and material on the acoustic noise emission of medium frequency, medium voltage transformers. 17th European Conference on Power Electronics and Applications (EPE'15 ECCE-Europe) (pp. 1–11).
- Sun, K., Yuan, Sh., & Qiu, Yu. (2017). Development of UHV power transmission in China. In Ultra-high voltage AC/DC power transmission, advanced topics in science and technology in China (pp. 23–37). Springer.
- Tong, B., Qingxin, Y., Rongge, Y., & Lihua, Z. (2017). Magnetically controlled saturable reactor core vibration under practical working conditions. IEEE Transactions on Magnetics, 53(6), 1–4. https://doi.org/10.1109/TMAG.2017.2664104
- Tu, P. M., Hung, B. D., Nam, P. H., Trinh, Tr. C., Minh, B. C., Bao, D. Th., & Vuong, D. Q. (2021). Finite element modeling of shunt reactors used in high voltage power systems. Engineering, Technology & Applied Science Research, 11(4), 7411–7416.
- Wrona, S., Pawelczyk, M., & Cheng, L. (2022). Sound transmission through a panel with a hybrid active and semi-active control system. Journal of Sound and Vibration, 536, 117172. https://doi.org/10.1016/j.jsv.2022.117172
- Yinbiao, S., & Weijiang, Ch. (2018). Research and application of UHV power transmission in China. High Voltage, 3(1), 1–13.
- Yuan, Y., Gao, X., Zhou, J., Liu, G., Kuang, X., Yang, L., & Liao, R. (2022). A review: Research on corrosive sulphur in electrical power equipment. High Voltage, 7(2), 209–221. https://doi.org/10.1049/hve2.12155
- Zhang, P., Li, L., Cheng, Z., Tian, C., & Han, Y. (2019). Study on vibration of iron core of transformer and reactor based on maxwell stress and anisotropic magnetostriction. IEEE Transactions on Magnetics, 55(2), 1–5. https://doi.org/10.1109/TMAG.2018.2875017