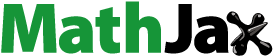
Abstract
This study investigates the chemical and physical properties of fine and coarse aggregates and their implications for concrete performance. The research employed an experimental approach to analyze various parameters, including chemical composition, chloride content, particle distribution, and slump, among others. The mixed proportions of concrete were carefully controlled to assess the influence of different aggregate compositions on concrete properties. The study utilized ASTM standards for testing procedures and calculations. Key findings of the research include the significant impact of chemical composition on concrete properties, with variations in oxides such as MgO, Al2O3, SiO2, K2O, CaO, Fe2O3 and Na2O influencing setting time and strength development. Moreover, the chloride content of fine aggregates varied across different sources, with implications for concrete durability. Particle distribution analysis revealed the importance of optimizing aggregate gradation for desired concrete properties. The study’s outcomes emphasize the importance of careful mix design and aggregate selection in optimizing concrete performance and durability. Recommendations for future research include further exploration of the mechanical behavior properties of concrete and assessment of environmental sustainability considerations in aggregate production processes. This research contributes valuable insights into optimizing concrete mix designs and enhancing the durability of concrete structures. Based on the findings, it is recommended to carefully consider aggregate characteristics and sources to achieve desired concrete properties. Overall, this study underscores the significance of aggregate selection in achieving durable and sustainable concrete structures.
Introduction
Concrete, as a major construction material, relies heavily on the quality of its components for optimal performance. Among these components, fine aggregates play a pivotal role, significantly influencing strength and stability (Khadka et al., Citation2022; Quayson & Mustapha, Citation2019). The diversity in fine aggregate types, derived from various sources and locations, introduces a dynamic element to concrete production, demanding a thorough investigation into their characteristics and the ensuing impact on compressive strength and durability. Fine aggregates, comprising particles smaller than 5 millimetres in diameter, include natural sand, crushed stone sand, and manufactured sand, among others (Wembe et al., Citation2023), serve as key ingredients in determining the properties and performance of concrete. Understanding the properties and suitability of different fine aggregate types is essential for achieving desired concrete properties and ensuring the integrity of concrete structures. Moreover, research suggests that variations in fine aggregate characteristics, such as particle shape, size distribution, and surface texture, can significantly affect concrete workability, durability, strength and resistance to environmental factors (Nedeljković et al., Citation2021; He et al., Citation2016). Thus, investigating the relationship between fine aggregate properties and concrete performance is crucial for advancing knowledge on construction materials and engineering practices.
Previous studies have outlined key determinants affecting concrete strength, including the cement-to-water ratio, cement-to-aggregate ratio, density, and aggregate size (Abdullahi, Citation2012; Chen et al., Citation2022; Li et al., Citation2020; Li et al., Citation2023; Sarireh & Al-Baijat, Citation2019; Wang & Song, Citation2021). However, the nuanced variations in fine aggregates arising from geographical and environmental factors bring an additional layer of complexity to this relationship (Ajagbe et al., Citation2018a; Ajagbe, Tijani, Arohunfegbe, et al. Citation2018). Emmanuel et al. (Citation2023) examined the durability of concrete made with fine aggregate from various sources using mathematical modelling. Their findings indicate that the geographical source of fine aggregate affects its suitability for the production of particular concrete types, whether structural lightweight concrete or high-strength concrete.
Numerous works have explored the properties of concrete prepared from fine aggregates sourced from diverse geographic locations and environmental conditions (Ajagbe et al., Citation2018c; Aves, Citation2022; Jackson & Akomah, Citation2018; Nicoara et al., Citation2020; Rahman, Citation2020). Acknowledging this, the present study aims to delve into the unique qualities of locally available fine aggregates in the Sekondi-Takoradi and Ahanta West Districts of Ghana. The research by Nilimaa (Citation2023) and Sohail et al. (Citation2020) highlights the importance of locally sourced aggregates in sustainable construction practices, emphasizing the need for thorough characterization and testing. Therefore, understanding the characteristics and performance of fine aggregates sourced from specific regions is crucial for optimizing concrete mix designs and enhancing construction practices (Nedeljković et al., Citation2021; Yaragal et al., Citation2019).
Memon et al. (Citation2023), Aves (Citation2022), Emmanuel et al. (Citation2023), and Jackson and Akomah (Citation2018) contributed valuable insights into the intricate relationship between fine aggregates and concrete properties. Notably, the study by Ozyildirim et al. (Citation2020) delves into the effect of fine aggregate characteristics on concrete strength and durability, while Wang et al. (Citation2021) explore the influence of fine aggregate gradation on concrete workability and performance. Additionally, Iron et al. (Citation2024) examined the role of fine aggregate shape on concrete mechanical properties, shedding light on the importance of particle geometry in concrete mixture design.
Several other studies have focused on the aggregate – aggregate–concrete interactions (Memon et al., Citation2023; Aves, Citation2022; Devadass, Citation2019; Iron et al., Citation2024). Additionally, studies by Medina et al. (Citation2017) and Prokopski et al. (Citation2020) have demonstrated the potential of granite quarry dust as a supplementary material in concrete production, indicating its positive effects on strength and durability properties. Recent investigations by Ukpata et al. (Citation2024) and Ashraf and Noor (Citation2011) have underscored the significance of considering aggregate properties, such as gradation and shape, in optimizing concrete mix designs for enhanced performance and longevity.
These diverse studies collectively contribute to our understanding of the complex interplay between fine aggregates and concrete properties, emphasizing the need for comprehensive investigation and analysis in this field. While previous studies have extensively examined the individual characteristics of fine aggregates and their influence on concrete properties, there remains a lack of comprehensive analysis that considers the combined effects of various characteristics of fine aggregates and their blends with granite quarry dust on performance characteristics of concrete. This paper provides insights into the synergistic effects of the characteristics of different aggregate types on concrete behavior by focusing on locally available fine aggregates and the incorporation of 50% granite quarry dust replacement. Through an in-depth examination of the characteristics of these fine aggregates, our primary objective is to elucidate their specific influence on the compressive strength of concrete.
Materials and methods
Materials
Fine aggregates used in this work were sourced from three locations in the Sekondi-Takoradi and Ahanta West Districts in the Western Region of Ghana. Six fine aggregate samples were collected, following ASTM C702/C702M-20 Standard Test Method for Reducing Samples of Aggregate to Testing Size. Three of the samples were sourced from Asakae (4°55'60" N and 1°48'0" W), Kejebil (4.9248° N, 1.8440° W), and Apowa (4.8963° N, 1.8172° W), in accordance with ASTM D75/D75M-19 Standard Practice for Sampling Aggregates. Refer to for the geographical location on the map, as outlined in ASTM D420-98(2013) Standard Guide for Site Characterization for Engineering Design and Construction Purposes. The fourth, fifth, and sixth samples were obtained by replacing 50% of the sampled fine aggregates from Asakae, Apowa, and Kejebil with granite quarry dust, in compliance with ASTM C33/C33M-18 Standard Specification for Concrete Aggregates.
Figure 1. The locations of the areas where fine aggregate samples were collected are denoted by red spots.

The granite quarry dust was sourced from Justmoh Quarry, located at Essipon (4.9706° N, 1.6919° W), near the Western Regional Capital of Ghana.
Cement and fine and coarse aggregates underwent testing for various properties to conduct the mixed design of the concrete. Ordinary Portland Cement Type II (CEM II) with a specific gravity of 3.15 and a 42.5 R grade was utilized, following the ASTM C-150/C-150M-21a standard. A uniform mix ratio of 1:1:2 was employed for concrete production, adhering to the ASTM C-192/C-192M-20 standard. Twelve cubes measuring 150 × 150 × 150 mm were cast for each fine aggregate, in accordance with the ASTM C-31/C-31M-19 standard. Additionally, 12 concrete cubes were produced for each sampled aggregate by replacing 50% of the fine aggregates sourced from Asakae, Kejebil, and Apowa with quarry dust, following the ASTM C-109/C-109M-20 standard. This resulted in a total of 72 concrete cube samples. All cubes were demoulded after 24 ± 3 hours and then cured for four separate periods in a curing water tank maintained at 29 ± 3 °C before testing. The curing periods were 3, 7, 14, and 28 days, in accordance with the ASTM C-192/C-192M-20 standard. shows a flowchart depicting the work process of the study.
Ordinary Portland cement (OPC)
The cement used as the binding material in this study was OPC (CEM II) produced by GHACEM, which conforms to ASTM C150/C150M-19 standards. It has a specific gravity of 3.15 and is classified as 42.5 R grade. The cement was sourced from an open store at New Amanful Junction, near Apowa in the Ahanta West Municipal Assembly, Ghana.
Aggregates
The fine aggregates, sourced from Apowa, Kejebil, Asakae, and Quarry Dust from Justmoh Quarry within the Sekondi-Takoradi Metropolis and Ahanta West Municipality, adhere to the BS 1377: PART 2 standard for grading and particle size distribution and pass through a BS 200 sieve. The coarse aggregates, sized 19 mm, conform to the BS 1377: PART 2 standard for grading and particle size distribution and are retained on the BS 200 sieve. Prior to utilization in concrete production, it was ensured that the aggregates were moisture-free.
Water
Portable drinking water produced by Ghana Water Company, conforming to ASTM C1152 standards, was utilized for this investigation.
Mixture proportion of concrete
The mix designs for the produced concrete are listed in , following ASTM C876-15 and ASTM C136 standards. A mixing ratio of 1:1:2 and a water/cement ratio of 0.5 were utilized, as indicated in . The mixing composition was computed using the Absolute Volume Method, expressed as:
(1)
(1)
where
is the weight of water,
is the weight of cement,
is the weight of coarse aggregates,
is the specific gravity of fine aggregates, and
is the specific gravity of the coarse aggregates.
Table 1. Mix design of concrete.
Measurements of 22.5 kg of fine aggregates, 22.5 kg of cement, and 45 kg of coarse aggregates with a 19 mm size were taken using a 30 kg electronic scale calibrated by the Ghana Standards Authority (GSA). The fine aggregates and cement were thoroughly mixed in a large, flat pan to achieve a uniform blend. Subsequently, the coarse aggregates and 11,250 ml of distilled water were added, and all components were mixed thoroughly to ensure complete blending.
Experiments
In order to accomplish the goal of this investigation, a series of tests were conducted on aggregates and concrete cubes after they had been cured. The following tests were conducted in accordance with their respective standards:
Grading/Particle size distribution (ASTM C136)
Organic impurity test (ASTM C40)
Chloride content test (ASTM C1218)
Bulk density test (ASTM C29)
Specific gravity test (ASTM C128)
Hydrometer test (ASTM C128)
Los Angeles abrasion (ASTM C131)
Aggregate crushing value (ACV) (BS 812)
Aggregate impact value (AIV) (BS 812)
Flakiness and elongation (ASTM D4791)
Coarse aggregate soundness test (ASTM C88)
Slump test (ASTM C143)
Compressive strength test (ASTM C39)
Grading/particle size distribution
Using the ASTM C136 and ASTM C117 standards, the particle size distributions were determined for all sampled fine and coarse aggregates. The samples were collected in a pan, weighed on a 3 kg G&G electronic scale, and soaked for 5 hours. Subsequently, the soaked samples were washed through a BS200 75 µm sieve under running distilled water to remove all the silt, following the procedures outlined in ASTM C117. The washed samples were then dried in an ECDO-3 drying oven at a temperature of 105 °C for 24 hours, adhering to ASTM C136 guidelines. After drying, the samples were air-cooled and rewashed, as per ASTM C117 specifications, and the mass was measured. Subsequently, the samples were dried and sieved through BS300/BS 200 sieves, with the mass retained in the sieves weighed and recorded, in accordance with ASTM C136 standards.
Organic impurity
The determination of organic impurities in the fine aggregates was conducted in accordance with ASTM C40 standard. Distilled water (97 g) was measured using a G&G electronic scale. Sodium Hydroxide pellets (3 g) were dissolved in the distilled water to prepare a 100 g solution. The fine aggregates sample was transferred into a measuring cylinder (EX 20 °C MC 250 ml ± 2 ml) and filled up to the 130 ml mark. The prepared Sodium Hydroxide solution was then added to the same measuring cylinder up to the 200 ml mark, and the mixture was left undisturbed for 24 hours. The degree of impurity was determined based on the colour intensity of the solution, where a darker colour indicated higher impurity levels.
Chloride content test
The chloride content of all the fine aggregates was determined following the ASTM C1152 standard. Then, 50 g of the sieved sample passing through the 2 mm sieve was soaked with distilled water in a 1:2 portion and left to settle for an hour. Cloudy water formed above the settled aggregates. 100 ml of the cloudy water was transferred into a beaker. The drop of 0.05 N Potassium Chloride solution was added to the cloudy water until it turned yellow. 0.05 N Silver Nitrate (AgNO3) was transferred into a burette, and its initial level was recorded. It was then titrated into the beaker containing the yellow solution until a brick-red colour was observed. The final level of AgNO3 was recorded, and the volume dispensed was calculated by simply subtracting the final volume from the initial volume. This volume of AgNO3 dispensed was used to determine the Chloride content of the fine aggregates following the calculation specified in Equation (2):
(2)
(2)
where 35.5 u is the atomic weight for chlorine,
for the normality of AgNO3,
is the volume (in ml) of the AgNO3 solution used for the titration of the test solution,
is the volume (in ml) of the sample solution of the concrete powder, and
is the mass of the sample.
Specific gravity tests
The specific gravity test was conducted for all fine and coarse aggregate samples in accordance with ASTM D854 standards. The temperature of the distilled water was measured using an AR320 infrared thermometer. For each sample, 50 g was taken using a pycnometer. The following measurements were recorded:
The weight of the pycnometer only, denoted by
The combined weight of the pycnometer and the samples, denoted by
The combined weight of the pycnometer, distilled water, and sample, denoted by
The weight of the pycnometer and water only, denoted by
Specific gravity was calculated using the formula:
(3)
(3)
Hydrometer
A hydrometer test, conducted in accordance with ASTM D422 standards, was performed on the sample to determine the size of the soil particles. Dry samples were sieved through a 2.0 mm sieve. 50 g of the samples were weighed using an electronic scale. Sodium Hexametaphosphate (125 ml) was added to the sample and stirred thoroughly. The solution was left overnight to allow the soil particles to disintegrate. The next day, the solution was transferred into a 1000 ml volumetric flask. The flask was filled up to 1000 ml with distilled water and shaken thoroughly. A washed bottle was then used to wash all the soil content sticking to the wall of the flask above the 1000 ml mark. A hydrometer bulb was dropped into the flask immediately after shaking, and the flask was placed down. Readings were taken according to the timings specified in the test sheet (30 s, 1 min, 2 min, 4 min, 8 min, 15 min, 30 min, 1 hour, 2 hours, 4 hours, 6 hours, 12 hours, and 24 hours). The sample was then washed under clear running water, oven-dried at 105 °C for 24 h, and graded.
Los Angeles abrasion
The Los Angeles abrasion test was conducted in accordance with ASTM C131 standard procedures. Initially, two 2500 g samples of coarse aggregate were prepared: the first sample, passing through a 12.5 mm BS 300-2069 perforated plate sieve but retained on a 9.5 mm sieve, and the second sample, passing through a 10 mm sieve but retained on a 9.5 mm sieve. Each sample was weighed on a 30 kg G&G electronic scale. Subsequently, the two sieved samples, totaling 5000 g, were introduced into a Los Angeles abrasion test machine as shown in . Eleven steel balls with a diameter of 48 mm, each weighing 0.390 kg, were then gently dropped into the machine containing the prepared samples. The machine was activated by pressing the power button, initiating a counterclockwise rotation for 500 cycles before automatically stopping. Following the completion of the test, the crushed aggregates were sieved using a 1.18 mm sieve. The aggregates retained on the 1.18 mm sieve were weighed using a 30 kg electronic scale, and any differences in weight were recorded.
Aggregate Crush Value (ACV) Test
The Aggregate Crushing Value (ACV) Test was conducted in accordance with BS 812. The weight of the empty cylinder in the base plate was measured and recorded as W. Coarse aggregates were then poured into the cylinder positioned on the base plate in two layers, and each layer was tamped 25 times with a small metal tamping rod. The weight of the aggregates was measured using a 3 kg G&G electronic scale and recorded as W1. The aggregates were gently poured into the base plate, and the load was released by raising the handle and releasing the locker pin for load transfer onto the aggregates in the base plate. The load was released directly onto the aggregates 15 times. Subsequently, the crushed aggregates were sieved through a 2.35 mm perforated base plate, and the retained sample was weighed on a 3 kg electronic scale, recorded as W2. The ACV was then calculated using the following formula:
(4)
(4)
Aggregates impact value (AIV) test
The aggregate impact value test was conducted according to the BS 812 standard to determine the resistance to sudden impact or shock. Aggregates ranging in size from 10.0 mm to 12.5 mm were utilized. Initially, the aggregates were dried by heating at 105 °C for a period of 4 hours and then cooled. Subsequently, the aggregates were sieved through the 12.5 mm and 10.0 mm IS sieves. The aggregates retained on the 10.0 mm sieve were selected for the test. A measuring cylinder was one-third filled with the aggregates, and the samples were compacted by administering 25 gentle blows using a rounded-end tamping rod. Two additional layers were added and compacted similarly to fill the measuring cylinder completely. Any surplus samples were struck off. The net weight of the aggregate, denoted as W1, was determined by bringing the impact machine to rest without wedging it. The hammer on the impact machine was raised to a height of 380 mm above the surface of the aggregates in the cup and then allowed to fall freely on the sample 15 times at intervals. The crushed aggregates were subsequently removed from the cup and sieved through a 2.36 mm sieve. The fraction that passed through the sieve was weighed and labelled as W2. Finally, the AIV was determined using the following formula:
(5)
(5)
Flakiness and elongation
The flakiness index was determined following ASTM D4791 to assess the suitability of coarse aggregate for concrete production. The dried aggregate sample underwent successive sieving through 63 and 6.3 mm sieves. The retained aggregates on each sieve (W1 and W2) were weighed, and the percentage of retained samples was calculated. The samples passing through the appropriate slot from each size range were combined and weighed. This weight was then divided by the total weight of the sample from different sizes to determine the desired flakiness index.
Additionally, a portion of the aggregate sample was sieved through 63 and 6.3 mm sieves as per ASTM D4791 standard. Aggregates retained on the 63 mm sieve and those passing through the 6.3 mm sieve were excluded. However, aggregates passing through the 63 mm sieve but retained on the 6.3 mm sieve were weighed and recorded. These aggregate samples were then passed through their corresponding length gauges or elongation gauges, using the Elongation Index Metal Gauge. Samples not passing through the length gauge were weighed and recorded. The elongation index was calculated as the percentage of total weight of samples retained on various length gauges.
Soundness
To determine the resistance of aggregates to disintegration by weathering and, in particular, freeze-thaw cycles, a soundness test was conducted following ASTM C88/C88M – 18 standards. The samples were wet sieved through an IS sieve nest ranging in size from 300 microns to 10 mm. The samples that passed through the 10 mm sieve and were retained on a 300-micron sieve were dried at 105 °C for 15 h. The samples were again sieved through a series of 10 mm, 4.75 mm, 1.18 mm, 600-micron, and 300-micron sieves. 100 g of each sieved sample was taken and placed in a separate test container. The samples were placed in a mesh basket and immersed in a solution of Sodium Sulphate for 16 hours in accordance with ASTM C88/C88M – 18 standards. The samples were removed from the solution and drained for 15 minutes. Subsequently, they were placed in an oven and dried at a temperature of 105 °C for 4 hours following the same ASTM standard. Once dried, the samples were cooled to room temperature. These samples were then sieved again on the same sieves on which they were retained before the test, as per the requirements of ASTM C88/C88M – 18.
Slump test
The workability of all the mixes was assessed using the cone method in accordance with ASTM C143/C143M – 10 standards. The procedure involved dampening the cone, placing it on a flat surface, and securely holding it in place, as depicted in . The concrete sample was then poured into the cone using a scoop, following which it was compacted in three layers. Each layer was compacted by tamping 25 times with a rod measuring 15 mm in diameter and 600 mm in length to eliminate any voids. Subsequently, the brim of the cone was levelled with a trowel to remove excess concrete. The cone was gradually removed and placed upside down near the concrete, with the tamped rod positioned over the cone. The reduction in the height of the concrete relative to that of the cone was measured using a scale ruler.
Compressive strength test
As per , six composite components were prepared and measured. Mixing was conducted thoroughly to achieve a homogeneous mixture of the components following ASTM C109 standards. Concrete cubes of dimension 150 × 150 × 150 mm were moulded using 12 cubes. The moulds were thoroughly greased to prevent adhesion of concrete as per standard procedure. Concrete was cast into the moulds in three layers, with each layer tamped 25 times using a rod of diameter 15 mm and length 600 mm to eliminate voids. A total of 144 concrete cubes were cast. Four sets of these concrete samples, each containing 30 cubes, were cured separately for 3, 7, 14, and 28 days respectively. Cubes were demoulded 24 ± 3 hours after casting and placed in a curing tank at a temperature of ±26 °C until testing. Concrete cubes were weighed, and compressive strength tests were conducted on the 3rd, 7th, 14th, and 28th days of curing using a CTM-2000 compressive test machine, calibrated according to the standards set by the GSA.
Results and discussion
Chemical properties of fine aggregates
The chemical properties of fine aggregates play a critical role in determining the strength development of concrete structures (Joel & Mbapuun, Citation2017; Khadka et al., Citation2022; Lee & Lee, Citation2019; Zhao et al., Citation2021; Chen & Liu, Citation2004). presents the chemical composition of the fine aggregates, highlighting key oxides such as MgO, Al2O3, SiO2, K2O, CaO, Fe2O3, and Na2O. These constituents are known to influence various properties of concrete, including setting time and early and final strengths. Variations in chemical composition can significantly affect the performance of concrete and must be carefully considered in mix design.
Table 2. Chemical composition of fine aggregates.
Furthermore, and provide the chloride and oxygen contents of the fine aggregates. BS and PS exhibit relatively low chloride levels compared to NS. The chloride content of aggregates is a critical consideration, especially in concrete applications where embedded metal corrosion must be minimized. The results suggest that BS and PS meet the maximum allowable limit for chloride content in concrete, as prescribed by ASTM C40 standards. However, the higher chloride content in NS warrants further investigation into its suitability for specific concrete applications, particularly those involving reinforced structures or marine environments.
Table 3. Chloride and oxygen contents of fine aggregates.
The organic impurity test revealed distinct dissolution behaviours among the fine aggregates. BS displayed rapid colour change within 2 hours, contrasting with PS and NS, which exhibited colour changes within 6 and 24 hours, respectively. Notably, the NS sample, sourced from a storm drain, showed prolonged colour change, indicative of potential organic contamination. These findings underscore the importance of assessing organic impurities in fine aggregates, as they can adversely affect concrete properties and performance.
Physical properties of coarse aggregates
The physical properties of coarse aggregates, such as bulk density, specific gravity, soundness, flakiness, elongation, 10% fineness, and Los Angeles abrasion, were evaluated to assess their suitability for concrete production. The observed percentage loss during the sodium sulphate soundness test was within the acceptable range specified by ASTM C88 and AASHTO T104 standards, indicating adequate durability of the aggregates. However, the flakiness and elongation indices of the aggregates exceeded the recommended limits set by BS-1241 and ASTM D 4791 standards, suggesting potential challenges in achieving optimal concrete mixtures with these aggregates. It is essential to address these issues through proper aggregate blending and grading to improve concrete workability and performance.
The physical properties of coarse aggregates, as outlined in , are critical for determining the durability and workability of concrete mixes. Our findings demonstrate that the samples met industry standards, with a maximum loss of 2.5% during the sodium sulphate soundness test, in line with ASTM C88 and AASHTO T104 requirements. Notably, particle shape characteristics, including flakiness and elongation, were within acceptable limits specified by BS-1241 and ASTM D 4791 standards.
Table 4. Physical properties of coarse aggregates.
Furthermore, our analysis revealed variations in bulk density and specific gravity among the coarse aggregates, influenced by their mineral compositions. Importantly, the Los Angeles abrasion test results indicated moderate abrasion resistance, consistent with basaltic rock types commonly used in construction materials (Mohajerani et al., Citation2017; ASTM C131).
Particles distribution analysis
Particle distribution analysis unveiled variations in the size distribution of fine aggregates sourced from diverse origins. While certain aggregates conformed to ASTM standards for particle size distribution, others diverged from the specified limits (Balitsaris, Citation2012; Ferreira et al., Citation2023). The presence of silt in multiple samples underscores the necessity for meticulous consideration of particle size distribution in concrete mix design. The coefficients of uniformity and curvature offer insights into the gradation characteristics of the aggregates. Specifically, aggregates from sources BS and BSQ demonstrated uniform gradation in contrast to those from NS and PS. Conversely, samples from PS and PSQ were categorized as poorly graded, highlighting the significance of optimizing aggregate gradation to attain desired concrete properties (Lindquist et al., Citation2015; Heyen et al., Citation2013; Sabih et al., Citation2016; Xiang et al., Citation2021). Analysis of particle size distribution furnishes critical information regarding the grading and uniformity of aggregates, essential for refining concrete mix designs. Our findings, as illustrated in and explicated in , underscore discrepancies in particle sizes and distribution among the fine aggregates (Cepuritis et al., Citation2017; Fomina & Polyanskiy, Citation2019; Zhou et al., Citation2014). Noteworthy deviations from ASTM standards were noted in certain samples, suggesting potential variability in concrete workability and strength.
Table 5. Physical properties of fine aggregates.
Furthermore, the coefficient of uniformity and coefficient of curvature values delineate varying degrees of uniformity across the samples. Aggregates sourced from BS and BSQ exhibited uniform grading, while those from NS and NSQ demonstrated well-graded characteristics. Conversely, samples from PS and PSQ displayed poor grading, potentially impacting concrete workability.
Slump
The slump test is a crucial procedure employed to assess the ease with which concrete paste flows and conforms to the shape of a mold. Widely utilized in construction sites worldwide, this test is instrumental in detecting variations in the uniformity of a given nominal proportion mix (ACI 116 R-90, 2000). Although not explicitly measuring concrete workability, it serves as an indicator of consistency.
Slump values obtained from concrete batches using different fine aggregates are depicted in . These values indicate a true slump for all tested fine aggregates, suggesting uniformity in the mix and confirming the concrete’s durability and workability. Notably, Black Sand (BS) exhibited a slump of 30 mm, classified as relatively low due to water absorption by the aggregate. Conversely, Pit Sand (PS), sourced from Kejebil, demonstrated the highest slump of 90 mm. PS, characterized by its angular particle shape and coarser grain size, is considered the most suitable sand type for concrete work.
The slump test serves as a pivotal tool for evaluating concrete workability and consistency. Our findings, as depicted in , showcased uniform slump values across all fine aggregates, indicative of consistent mix properties. Furthermore, variations in slump values were observed, with PS registering the highest slump of 90 mm, attributed to its coarser grain size and angular particle shape (ACI 116 R-90, 2000; Babafemi et al., Citation2018).
Density of concrete
The density results of the concrete samples are presented in . The graphs in illustrate the relationship between concrete density, curing days, and compressive strength. It was observed that the densities of all samples increased over the 28-day measurement period. Notably, the BSQ and BS samples exhibited the highest densities, measuring 2361 and 2357 kg/m3, respectively (Kosmatka et al., Citation2002; Liu et al., Citation2019). PS and PSQ, despite having lower densities, still met standard requirements and could be utilized in reinforced concrete structures (Saberian et al., Citation2019).
Table 6. The average density of concrete produced with different fine aggregates.
The density of concrete is influenced by aggregate density, air entrapped or purposefully entrained, and the amount of aggregate used (Wang et al., Citation2023). Denser concrete typically possesses greater strength and fewer voids and pores, impacting water and solubility permeability (Ozyildirim et al., Citation2020).
illustrates the relationship between density and compressive strength, affirming previous findings that uniformly distributed aggregates contribute to enhanced concrete density and compressive strength (Laldintluanga et al., Citation2020; Liu et al., Citation2018; Mandal et al., Citation2020). Furthermore, depicts the density of samples with varying compressive strengths, revealing a nonlinear increase in compressive strength with average density.
Concrete density is pivotal in determining mechanical properties and structural performance. Our analysis, as detailed in and supported by , indicated an increase in density over the curing period. Notably, BSQ and BS samples exhibited densities within the range of conventional concrete materials.
Furthermore, the relationship between concrete density and compressive strength, depicted in , revealed a positive correlation, consistent with previous studies (Laldintluanga et al., Citation2020; Liu et al., Citation2018; Mandal et al., Citation2020). Higher aggregate densities resulted in denser concrete mixes, contributing to enhanced compressive strength.
Compressive strength
The analysis of density and compressive strength reveals a positive correlation between the two parameters, indicating that the compressive strength of concrete samples increases linearly with increasing density (Othman et al., Citation2021; Zaid et al., Citation2021). This relationship is depicted in , which illustrates the trend observed in the experimental data. Additionally, presents the results of average compressive strength tests conducted on concrete specimens aged 3, 7, 14, and 28 days. Notably, concrete formulations containing 50% fine aggregates from the Asakae source, designated as Black Sand (BS), and 50% quarry dust (BSQ) demonstrated the highest compressive strength of 37 MPa among the six types of concrete cubes produced using various fine aggregate sources. The high compressive strength observed in BS and BSQ formulations can be attributed to factors such as their particle size distribution, coefficient of uniformity (Cu), coefficient of curvature (Cc), fineness modulus (FM), and density (Nair & Ozyildirim, Citation2019; Lee & Lee, Citation2019).
Contrary to previous reports (Rashid et al., Citation2018) suggesting that organic impurities may reduce compressive strength, our study observed that BS, which contained the highest organic impurity levels, exhibited superior compressive strength performance. This suggests a possible nullification of the detrimental effects of organic impurities on compressive strength in the presence of higher organic impurity concentrations. Moreover, certain studies have highlighted the potential of some organic impurities to increase concrete density, thereby enhancing compressive strength (Iffat, Citation2015; Ngugi et al., Citation2014; Skominas et al., Citation2020).
According to ACI 301-1.6.6 standards, the measured compressive strength of concrete samples should meet or exceed specified design strength requirements, with no strength test falling below the design strength by more than 3.45 MPa. Our study demonstrated that concrete samples surpassed the targeted strength of M25 after 28 days of curing, with BSQ formulations exceeding the target strength by 50%. The obtained compressive strength values fall within the range of lightweight concrete and meet the 28-day compressive strength criteria outlined in BS EN 12390-3 (2003) and ASTM C39 standards.
Compressive strength serves as a critical indicator of concrete performance and structural integrity (Chen et al., Citation2023; Iffat, Citation2015; Zhong et al., Citation2023). Our analysis, presented in and and , illustrate the progressive increase in compressive strength over the curing period. Notably, concrete mixes containing BS and BSQ fine aggregates exhibited superior compressive strengths, surpassing the requirements for M25-grade concrete. However, it is essential to acknowledge the limitations of our study, including potential variations in sample characteristics and testing conditions. Moreover, the precise impact of organic impurities on compressive strength warrants further investigation.
Table 7. Average compressive strength for all types of fine aggregates.
Our findings offer valuable insights into the chemical and physical properties of aggregates and their implications for concrete performance. By addressing concerns related to repeatability and reproducibility, this study contributes to the optimization of concrete mix designs and the development of durable infrastructure (Chen et al., Citation2023).
Conclusion
Findings
This comprehensive study delved into the chemical and physical properties of fine and coarse aggregates and their implications for concrete performance. Through meticulous analysis, several key findings emerged. Firstly, the chemical composition of fine aggregates, including oxides such as MgO, Al2O3, SiO2, K2O, CaO, Fe2O3, and Na2O, was found to significantly influence concrete properties such as setting time and strength development. Variations in chemical composition underscore the importance of careful mix design to optimize concrete performance. Additionally, the chloride content of fine aggregates varied across different sources, with implications for concrete durability, particularly in environments prone to metal corrosion. Organic impurity tests revealed distinct dissolution behaviours, highlighting the potential impact on concrete properties and performance.
Physical properties of coarse aggregates, including bulk density, specific gravity, and particle distribution, were also scrutinized. While the sodium sulphate soundness test indicated adequate durability, challenges in achieving optimal concrete mixtures were identified due to deviations from recommended limits in flakiness and elongation indices. Furthermore, particle distribution analysis underscored the importance of meticulous consideration of aggregate gradation to attain desired concrete properties.
The slump test results indicated uniformity in concrete mix properties across various fine aggregates, with variations in slump values attributed to differences in aggregate characteristics. Moreover, the density of concrete samples increased over the curing period, with certain formulations exhibiting superior compressive strength performance, surpassing design requirements.
Research limitations
Despite the comprehensive nature of this study, several limitations should be acknowledged. Variations in sample characteristics and testing conditions may have influenced the results, necessitating caution in generalizing findings. Additionally, the precise impact of organic impurities on concrete properties warrants further investigation, as does the optimization of aggregate blending and grading to address challenges identified in this study.
Recommendations for future research
Future research endeavours should focus on addressing the limitations of this study by exploring the mechanical behaviour properties of concrete in greater depth. Investigating the long-term durability and performance of concrete structures incorporating different aggregate compositions would provide valuable insights into their suitability for diverse applications. Moreover, assessing the environmental sustainability of concrete mixtures considering aggregate sources and production processes is imperative for advancing sustainable construction practices.
Implications and future directions
The findings of this study have significant implications for concrete mix design and the development of durable infrastructure. By optimizing aggregate compositions and addressing challenges identified in this research, concrete producers and engineers can enhance the performance and longevity of concrete structures. Furthermore, future research endeavours should explore additional mechanical behaviour properties of concrete to further expand the relevance and applicability of this study in the construction industry.
Author contributions
Bernard Kwame Mussey conceived the research objectives, with Lucas Nana Wiredu Damoah coordinating research efforts and overseeing experimental design. Richard Nii Ayitey Akoto and Yaw Delali Bensah provided insights into aggregate properties. Data analysis and interpretation were primarily led by Bernard Kwame Mussey and Lucas Nana Wiredu Damoah, with contributions from Richard Nii Ayitey Akoto and Yaw Delali Bensah. Manuscript drafting was a collaborative effort, with Bernard Kwame Mussey and Lucas Nana Wiredu Damoah taking the lead and Richard Nii Ayitey Akoto and Yaw Delali Bensah contributing significantly. All authors critically revised the manuscript, addressing reviewer comments collectively. All authors approved the final manuscript version for publication and agree to be accountable for its integrity and accuracy.
Acknowledgment
No funding was received.
Disclosure statement
No potential conflict of interest was reported by the author(s).
Data availability statement
The data and materials supporting the results or analyses presented in this article are available upon reasonable request. Requests for access to the data should be directed to the corresponding author.
Additional information
Notes on contributors
Bernard Kwame Mussey
Barnard Kwame Mussey: PhD candidate at Department of Materials Science and Engineering, University of Ghana, Affiliated to Department of Mechanical Engineering, Jubilee Technical Training Centre, Takoradi Technical University (TTU), Takoradi, Ghana. Research Interests include Cements and Concretes, Pozzolans and Rebar Corrosion inhibitors.
Lucas Nana Wiredu Damoah
Lucas Nana Wiredu Damoah: PhD, Department of Materials Science and Engineering, University of Ghana, Legon Accra, Ghana. Research Interests include Cements and Concretes, Corrosion Prevention, Failure Analysis, sustainable materials technologies and Refining of Metals.
Richard Nii Ayitey Akoto
Richard Nii Ayitey Akoto: PhD, Department Petroleum Studies, University of Professional Studies, Accra, Ghana. Research Interests include Concretes, Rock Mechanics, Fluid Flow in Porous Media and Nanotechnology.
Yaw Delali Bensah
Yaw Delali Bensah: PhD, Department of Materials Science and Engineering, University of Ghana, Legon Accra, Ghana. Research Interests include Concretes, Corrosion prevention, recycling, biomass and entropy generation.
References
- Abdullahi, M. (2012). Effect of aggregate type on compressive strength of concrete. International Journal of Civil and Structural Engineering, 2(3), 791–800. https://doi.org/10.6088/ijcser.00202030008
- Ajagbe, W. O., Tijani, M. A., & Agbede, O. A. (2018a). Compressive strength of concrete made from aggregates of different sources. Journal of Research Information in Civil Engineering, 15(1), 1963–1974. https://www.researchgate.net/publication/323148998.
- Ajagbe, W. O., Tijani, M. A., & Agbede, O. A. (2018c). Compressive strength of concrete made from aggregates of different sources. USEP Journal of Research Information in Civil Engineering, 15(1), 1963–1974.
- Ajagbe, W. O., Tijani, M. A., Arohunfegbe, I. S., & Akinleye, M. T. (2018). Assessment of fine aggregates from different sources in Ibadan and environs for concrete production. Nigerian Journal of Technological Development, 15(1), 7. https://doi.org/10.4314/njtd.v15i1.2
- Ashraf, W. B., & Noor, M. A. (2011). Performance-evaluation of concrete properties for different combined aggregate gradation approaches. Procedia Engineering, 14, 2627–2634. https://doi.org/10.1016/j.proeng.2011.07.330
- Aves, F. A. (2022). Impact of fine and coarse aggregates from distinctive sources on the compressive strength of concrete. International Research Journal of Advanced Engineering and Science, 7(2), 239–242.
- Babafemi, A. J., Šavija, B., Paul, S. C., & Anggraini, V. (2018). Engineering properties of concrete with waste recycled plastic: A review. Sustainability, 10(11), 3875. https://doi.org/10.3390/su10113875
- Balitsaris, M. (2012). Deviations in standard aggregate gradation and its affects on the properties of Portland cement concrete. All Theses, 1502. https://tigerprints.clemson.edu/all_theses/1502.
- Cepuritis, R., Garboczi, E. J., Ferraris, C. F., Jacobsen, S., & Sørensen, B. E. (2017). Measurement of particle size distribution and specific surface area for crushed concrete aggregate fines. Advanced Powder Technology, 28(3), 706–720. https://doi.org/10.1016/j.apt.2016.11.018
- Chen, B., & Liu, J. (2004). Effect of aggregate on the fracture behavior of high strength concrete. Construction and Building Materials, 18(8), 585–590. https://doi.org/10.1016/j.conbuildmat.2004.04.013
- Chen, B., Wang, L., Feng, Z., Liu, Y., Wu, X., Qin, Y., & Xia, L. (2023). Optimization of high-performance concrete mix ratio design using machine learning. Engineering Applications of Artificial Intelligence, 122, 106047. https://doi.org/10.1016/j.engappai.2023.106047
- Chen, J., Du, W., Zhao, G., Shi, M., & Xue, B. (2022). Effect of aggregate size and water/cement on compressive strength and physiological performance of planting concrete. Materials, 15(19), 6685. https://doi.org/10.3390/ma15196685
- Devadass, T. (2019). Experimental study on replacement of fine aggregate in concrete with dissimilar curing conditions. Case Studies in Construction Materials, 11, e00245. https://doi.org/10.1016/j.cscm.2019.e00245
- Emmanuel, O. I., Sunday, O. C., & Kenneth, M. C. (2023). Modeling of strength of concrete produced with fine aggregates from different sources. International Journal for Research in Applied Science and Engineering Technology, 11(12), 1425–1435. https://doi.org/10.22214/ijraset.2023.57598
- Ferreira, R. L. S., Medeiros, M., Pereira, J. E. S., Henriques, G. F., Tavares, J. C., Marvila, M. T., & de Azevedo, A. R. G. (2023). Effects of particle size distribution of standard sands on the physical-mechanical properties of mortars. Materials, 16(2), 844. https://doi.org/10.3390/ma16020844
- Fomina, N., & Polyanskiy, M. (2019). Grain size distribution of aggregates of crushed concrete. E3S Web of Conferences, 97, 02018. https://doi.org/10.1051/e3sconf/20199702018
- He, H., Courard, L., Pirard, E., & Michel, F. (2016). Shape analysis of fine aggregates used for concrete. Image Analysis & Stereology, 35(3), 159–166. https://doi.org/10.5566/ias.1400
- Heyen, W., Halsey, L., Rea, R., & Syslo, M. (2013). Optimized aggregates gradations for Portland cement concrete mix designs evaluation. Nebraska Department of Transportation Research Reports, 113, 1–39. http://digitalcommons.unl.edu/ndor/113
- Iffat, S. (2015). Relation between density and compressive strength of hardened concrete. Challenge Journal of Concrete Research Letters, 6(4), 182–189.
- Iron, U. H., & Ernest, A, Civil Engineering Department, University of Uyo, Nigeria. (2024). Comparative study of the crushing strengths of recycled coarse aggregates concretes and natural aggregates concretes. International Journal of Multidisciplinary Research and Analysis, 07(03), 960–968. https://doi.org/10.47191/ijmra/v7-i03-15
- Jackson, E. N., & Akomah, B. B. (2018). Analysis of the compressive strength of concrete with quarry dust, sand and mixture of them as fine aggregates. International Journal of Architecture, Engineering and Construction, 7(4), 41–45. https://doi.org/10.7492/IJAEC.2018.022
- Joel, M., & Mbapuun, I. D. (2017). Comparative analysis of the properties of concrete produced with Portland limestone cement (plc) Grade 32.5n and 42.5r for use in rigid pavement work. Global Journal of Engineering Research, 15(1), 17. https://doi.org/10.4314/gjer.v15i1.3
- Khadka, S., & Mishra, A. K, Post-Doctoral Research Scholar, Srinivas University, India and Associate Professor, Madan Bhandari Memorial Academy Nepal, Urlabari 3, Morang, Nepal. (2022). Effect of fine aggregate sources on compressive strength of cement concrete. Journal of Advanced Research in Construction and Urban Architecture, 7(1&2), 9–17. https://doi.org/10.24321/2456.9925.202201
- Kosmatka, S. H., Kerkhoff, B., & Panarese, W. C. (2002). Design and control of concrete mixtures (14th ed.). Portland Cement Association.
- Laldintluanga, H., Ramhmachhuani, R., & Thlengliani, R, Civil Engineering Department, Mizoram University, Tanhril, Aizawl–796004 (India). (2020). Evaluation of sand quality and its effect on mortar and cement concrete. Science & Technology Journal, 8(2), 62–68. https://doi.org/10.22232/stj.2020.08.02.10
- Lee, J., & Lee, T. (2019). Influences of chemical composition and fineness on the development of concrete strength by curing conditions. Materials, 12(24), 4061. https://doi.org/10.3390/MA12244061
- Li, S., Yang, J., & Zhang, P. (2020). Water-cement-density ratio law for the 28-day compressive strength prediction of cement-based materials. Advances in Materials Science and Engineering, 2020, 1–8. https://doi.org/10.1155/2020/7302173
- Li, X., Zhang, Y., Yang, T., Liao, H., Yu, L., Liu, Y., Wang, G., Zhao, Y., & Qiao, H. (2023). Study on the influence of specimen size and aggregate size on the compressive strength of rock-filled concrete. Applied Sciences, 13(10), 6246. https://doi.org/10.3390/app13106246
- Lindquist, W., Darwin, D., Browning, J., McLeod, H. A. K., Yuan, J., & Reynolds, D. (2015). Implementation of concrete aggregate optimisation. Construction and Building Materials, 74, 49–56. https://doi.org/10.1016/j.conbuildmat.2014.10.027
- Liu, X., Qu, S., & Huang, J. (2019). Relationship between physical properties and particle-size distribution of geomaterials. Construction and Building Materials, 222, 312–318. https://doi.org/10.1016/j.conbuildmat.2019.06.127
- Liu, Y., Sidhu, K. S., Chen, Z., & Yang, E. H. (2018). Alkali-treated incineration bottom ash as supplementary cementitious materials. Construction and Building Materials, 179(August), 371–378. https://doi.org/10.1016/j.conbuildmat.2018.05.231
- Mandal, S., Singh, J. K., Lee, D. E., & Park, T. (2020). Ammonium phosphate as an inhibitor to mitigate the corrosion of steel rebar in chloride-contaminated concrete pore solution. Molecules, 25(17), 3785. https://doi.org/10.3390/molecules25173785
- Medina, G., Sáez del Bosque, I. F., Frías, M., Sánchez de Rojas, M. I., & Medina, C. (2017). Granite quarry waste as a future eco-efficient supplementary cementitious material (SCM): Scientific and technical considerations. Journal of Cleaner Production, 148, 467–476. https://doi.org/10.1016/j.jclepro.2017.02.048
- Memon, B. A., Oad, M., & Buller, A. H. (2023). Effect of fine aggregate type on workability and compressive strength of recycled aggregate concrete. International Journal of Research and Review, 10(4), 152–162. https://doi.org/10.52403/ijrr.20230420
- Mohajerani, A., Nguyen, B. T., Tanriverdi, Y., & Chandrawanka, K. (2017). A new practical method for determining the LA abrasion value for aggregates. Soils and Foundations, 57(5), 840–848. https://doi.org/10.1016/j.sandf.2017.08.013
- Nair, H., & Ozyildirim, H. C. (2019). Lightweight aggregates and shrinkage-reducing admixtures for low-cracking concrete. ACI Materials Journal, 116(5), 91–98. https://doi.org/10.14359/51716830
- Nedeljković, M., Visser, J., Šavija, B., Valcke, S., & Schlangen, E. (2021). Use of fine recycled concrete aggregates in concrete: A critical review. Journal of Building Engineering, 38, 102196. https://doi.org/10.1016/j.jobe.2021.102196
- Ngugi, H. N., Mutuku, R. N., & Gariy, Z. A. (2014). Effects of sand quality on compressive strength of concrete: A case of Nairobi County and its environs, Kenya. Open Journal of Civil Engineering, 04(03), 255–273. https://doi.org/10.4236/ojce.2014.43022
- Nicoara, A. I., Stoica, A. E., Vrabec, M., Rogan, N. Š., Sturm, S., Ow-Yang, C., Gulgun, M. A., Bundur, Z. B., Ciuca, I., & Vasile, B. S. (2020). End-of-life materials used as supplementary cementitious materials in the concrete industry. Materials, 13(8), 1954. https://doi.org/10.3390/MA13081954
- Nilimaa, J. (2023). Smart materials and technologies for sustainable concrete construction. Developments in the Built Environment, 15, 100177. https://doi.org/10.1016/j.dibe.2023.100177
- Othman, R., Jaya, R. P., Muthusamy, K., Sulaiman, M., Duraisamy, Y., Abdullah, M. M. A. B., Przybył, A., Sochacki, W., Skrzypczak, T., Vizureanu, P., & Sandu, A. V. (2021). Relation between density and compressive strength of foamed concrete. Materials, 14(11), 2967. https://doi.org/10.3390/ma14112967
- Ozyildirim, H. C., Nair, H., & Sharifi, M. (2020). Field performance of low-cracking concretes for the closure pours and overlays of bridge decks. Transportation Research Record: Journal of the Transportation Research Board, 2674(5), 361–370. https://doi.org/10.1177/0361198120915703
- Prokopski, G., Marchuk, V., & Huts, A. (2020). The effect of using granite dust as a component of concrete mixture. Case Studies in Construction Materials, 13, e00349. https://doi.org/10.1016/j.cscm.2020.e00349
- Quayson, J. H., & Mustapha, Z. (2019). Impact of coarse aggregate on compressive strength of concrete. Built Environment Journal, 16(1), 52. https://doi.org/10.24191/bej.v16i1.9674
- Rahman, S. (2020). Analysis on compressive strength of concrete using different sources of fine aggregates. 2nd International Conference on Research and Innovation in Civil Engineering, Springer, January (pp. 25–29).
- Rashid, K., Ahmad, M., & Tahir, M. (2018). Influence of organic agents to compressive strength of cement mortar. Construction and Building Materials, 175, 434–438. https://doi.org/10.1016/j.conbuildmat.2018.04.177
- Saberian, M., Shi, L., Sidiq, A., Li, J., Setunge, S., & Li, C. Q. (2019). Recycled concrete aggregate mixed with crumb rubber under elevated temperature. Construction and Building Materials, 222, 119–129. https://doi.org/10.1016/j.conbuildmat.2019.06.133
- Sabih, G., Tarefder, R. A., & Jamil, S. M. (2016). Optimization of gradation and fineness modulus of naturally fine sands for improved performance as fine aggregate in concrete. Procedia Engineering, 145, 66–73. https://doi.org/10.1016/j.proeng.2016.04.016
- Sarireh, M., & Al-Baijat, H. (2019). Local aggregate in production of concrete mix in Jordan. Open Journal of Civil Engineering, 09(02), 81–94. https://doi.org/10.4236/ojce.2019.92006
- Skominas, R., Gurskis, V., Šadzevičius, R., & Ramukevičius, D. (2020). Effects of aggregates impurity on concrete properties. Proceedings of the 9th International Scientific Conference. Rural Development 2019, Vytautas Magnus University (vol. 2019, no. 1, pp. 198–202).
- Sohail, M. G., Alnahhal, W., Taha, A., & Abdelaal, K. (2020). Sustainable alternative aggregates: Characterization and influence on the mechanical behaviour of basalt fibre reinforced concrete. Construction and Building Materials, 255, 119365. https://doi.org/10.1016/j.conbuildmat.2020.119365
- Ukpata, J. O., Ewa, D. E., Success, N. G., Alaneme, G. U., Otu, O. N., & Olaiya, B. C. (2024). Effects of aggregate sizes on the performance of laterized concrete. Scientific Reports, 14(1), 448. https://doi.org/10.1038/s41598-023-50998-1
- Wang, C., & Song, M. (2021). Influence of water-cement ratio and type of mixing water on the early hydration performance of calcium sulphoaluminate (CSA) cement. Advances in Materials Science and Engineering, 2021, 1–10. https://doi.org/10.1155/2021/5557763
- Wang, X., Song, P., Yu, H., Taylor, P., Sadati, S., Freeseman, K., & Ning, Y. (2021). Extended life concrete bridge decks utilizing internal curing to reduce cracking – materials characterization and engineering demonstration. Construction and Building Materials, 275, 122163. https://doi.org/10.1016/j.conbuildmat.2020.122163
- Wang, Y., Xiao, R., Lu, H., Hu, W., Jiang, X., & Huang, B. (2023). Effect of curing conditions on the strength and durability of air entrained concrete with and without fly ash. Cleaner Materials, 7, 100170. https://doi.org/10.1016/j.clema.2023.100170
- Wembe, J. T., Ngueyep, L. L. M., Moukete, E. E. A., Eslami, J., Pliya, P., Ndjaka, J.-M B., & Noumowe, A. (2023). Physical, mechanical properties and microstructure of concretes made with natural and crushed aggregates: Application in building construction. Cleaner Materials, 7, 100173. https://doi.org/10.1016/j.clema.2023.100173
- Xiang, Z., Youjun, X., Guangcheng, L., & Jiangteng, L. (2021). Effect of surface characteristics of aggregates on the compressive damage of high-strength concrete based on 3D discrete element method. Construction and Building Materials, 301(124101), 1–14. https://doi.org/10.1016/j.conbuildmat.2021.124101
- Yaragal, S. C., Gowda, S. N. B., & Rajasekaran, C. (2019). Characterization and performance of processed lateritic fine aggregates in cement mortars and concretes. Construction and Building Materials, 200, 10–25. https://doi.org/10.1016/j.conbuildmat.2018.12.072
- Zaid, O., Ahmad, J., Siddique, M. S., Aslam, F., Alabduljabbar, H., & Khedher, K. M. (2021). A step towards sustainable glass fibre reinforced concrete utilizing silica fume and waste coconut shell aggregate. Scientific Reports, 11(1), 12822. https://doi.org/10.1038/s41598-021-92228-6
- Zhao, Y., Liu, Y., & Xu, B. (2021). Effect of coarse aggregate size distribution on fracture toughness of concrete based on boundary effect model. Theoretical and Applied Fracture Mechanics, 113, 102970. https://doi.org/10.1016/j.tafmec.2021.102970
- Zhong, Y., Wang, P., Zhang, B., Wang, Y., Liu, T., Li, X., & Niu, Y. (2023). Research on detection method of concrete compressive strength based on dielectric properties. Journal of Building Engineering, 76, 107090. https://doi.org/10.1016/j.jobe.2023.107090
- Zhou, L. J., Sun, F. N., & Zhao, Q. H. (2014). Particle size distribution of sandstone aggregate influence on recycled aggregate concrete properties. Applied Mechanics and Materials, 584–586, 1504–1508. https://doi.org/10.4028/www.scientific.net/AMM.584-586.1504