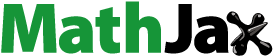
Abstract
Large quantities of defatted grape seed meal (DGSM) are discarded annually in the grape pomace industry. This residue could be exploited as a new source of non-animal protein to improve the chemical and color stabilization of red wines from warm regions. Different protein hydrolysates have been characterized, considering two different variables in their obtaining: type of raw material (DGSM from white and red grape pomaces) and hydrolysis time (1 and 4 h). The protein content of the hydrolysates, their molecular weight distribution, color, amino acid and peptide composition, and antioxidant activity were evaluated. Products obtained were mainly influenced by the type of raw material, regardless of the hydrolysis time. Hydrolysates from red DGSM displayed a higher protein content (∼67.4%) and protein yield (∼28.9%), visually perceptible (ΔE*ab > 3) lower lightness (∼23.3) and color intensity (∼13.2), and a greater variety of peptides related to red wine color stabilization. However, hydrolysates from white DGSM showed a higher amino acid content and antioxidant capacity (∼33.5 µmol TE/g, by DPPH; ∼3710.9 µmol TE/g, by ORAC). According to the results obtained, both red and white DGSM are susceptible starting materials to be selected as optimal for application in winemaking, fixing 1 h as hydrolysis time.
REVIEWING EDITOR:
1. Introduction
Nowadays, the reduction of the environmental impact generated by industries for the purpose of decreasing the effects of climate change is a global goal. In this context, the search for new uses of industrial by-products to reduce or remove the waste generated would contribute to mitigating global warming, apart from the additional economic benefit derived from their circular production (Dwyer et al., Citation2014; Maier et al., Citation2009). In the enology field, the main by-product generated by the wine industry is grape pomace, with a production of approximately 10 million tons annually (García-Lomillo & González-SanJosé, Citation2017), which is mainly consisting of stems, skins, and seeds, and whose composition is influenced by factors such as grape variety, cultivation, climate or the conditions of the winemaking processing (Bordiga, Citation2018). The main constituents of grape pomace are fiber (45-75% of the white and red grape pomace, respectively), and phenolic compounds (∼70%) due to their incomplete removal during vinification, followed by lipids (7-15%) and proteins (8%), characterized by high levels of glutamic and aspartic acids and low content of tryptophan and sulfur amino acids (Mora-Garrido et al., Citation2022).
Grape pomace processed by industry is revalued by extracting and obtaining alcohols, tartaric salts, natural phenolic colorants, and grape seed oil, among others (Mora-Garrido et al., Citation2022). Concretely, as a consequence of the industrial extraction of the grape seed oil from grape pomace seeds, tons of defatted grape seed meal (DGSM) is annually generated as a residue, mainly constituted by fiber (22%) and proteins (7-10%) (with low lipid (2%) and phenol amounts (3%) due to the exhaustion processes) (Mora-Garrido et al., Citation2022). This residue is normally discarded or used as biomass, reducing greenhouse gas emissions and thus contributing to reducing the impact of climate change (Dhanasekar & Sathyanathan, Citation2023; Samimi & Shahriari-Moghadam, Citation2023). The search for a new alternative use means a further reduction of its environmental impact by further reducing its carbon footprint and the generation of an associated economic benefit by obtaining a new product for its commercialization. Considering the huge quantities of grape pomace processed per year, and from an economical and environmental point of view, the DGSM could be likely to be considered as a potential natural low-cost source of vegetable proteins, peptides, or amino acids. This product could have different applications: as a protein substitute in dietary supplements, as an ingredient for vegan foods, for cosmetic formulations with bioactive peptides, or to improve some sensory properties of foods (Alvarez-Ossorio et al., Citation2022; Ma et al., Citation2019). In any of these or other possible uses, a more in-depth and specific study of these compounds would be necessary to determine if they fulfill the desired purpose.
In warm climate zones characterized by high temperatures in summer and a high number of annual hours of sunlight (Mira de Orduña, Citation2010), the time lag between the technological and phenolic ripening of grapes generated by the relentless advance of climate change, is increasingly accentuated. One of the main causes is that, at harvest, grape seeds present an unbalanced phenolic content, turning in a wine color instability by the diminution of the phenolic robustness (copigments) (Boulton, Citation2001). The high grape sugar content due to the high temperatures produces wines with high alcohol content and pH disequilibrium, which, in turn, affects the pigments stability. As a consequence, color instability is leading from the initial stages of winemaking and, therefore, during the aging process (Cejudo-Bastante et al., Citation2017; Gordillo et al., Citation2014). In the search for new strategies for improving the stabilization of red wines in warm climates, previous studies about the addition to wines of grape seed proteins have been proposed, considering their high techno-functional value in modulating the quality characteristics of red wines, such as their stability and color (Gazzola et al., Citation2017; Gordillo et al., Citation2021). Moreover, being a component of the grape itself, it could present fewer legal restrictions and an alternative to the use of animal proteins, such as milk and egg proteins, currently used in winemaking as fining agents (Marangon et al., Citation2019; Tschiersch et al., Citation2010).
In pursuit of wine color stabilizing by the addition of oenological proteins, is needed to assure their good dispersion in it. In this regard, enzymatic hydrolysis contributes solubilizing proteins in aqueous mediums, and affects the molecular weights and amino acid composition of the hydrolysates, improving their biological and technological properties. It also reduces the use of chemical solvents, making it an environmentally friendly alternative to more traditional methods (Bautista et al., Citation1996; Gazzola et al., Citation2017; Parrado et al., Citation2006). The utilization of different enzymes allows obtaining hydrolysates with different structural, functional and antioxidant properties, since each enzyme acts by scission at different sites of protein. Among the great variability of enzymes, alcalase is widely used to obtain peptides from plant proteins (Bučko et al., Citation2018; Ghribi et al., Citation2015b; Zhao et al., Citation2011). This enzyme is an endoprotease, i.e. it breaks bonds between amino acids located in the inner zone of the amino acid sequence, not at the ends, specifically between glutamine-histidine, serine-histidine, leucine-tyrosine, and tyrosine-threonine, generating peptides of small and medium size (Adamson & Reynolds, Citation1996; Suarez et al., Citation2022).
In this way, combining the use of the enzymatic hydrolysis and DGSM as a protein source of oenological origin, and based on the promising results obtained by Cejudo-Bastante et al. (Citation2016) after their pre-fermentative addition, the addition to wine of low and high molecular weight peptides derived from the DGSM by-product, in the early and more advanced stages of the red winemaking process, is proposed as an oenological strategy for a possible early stabilization of the phenolic structure and wine color. As part of a broader investigation, the first step was to optimize the methodology for obtaining protein hydrolysates from grape seed meal, from the processing of grape pomace from the harvest of the year 2021, focused on the protein extraction conditions prior to the enzymatic hydrolysis (Cejudo-Bastante et al., Citation2022). However, other factors such as sources and hydrolysis conditions also influencing the chemical characteristics of the hydrolysates obtained (Pojić et al., Citation2018).
Therefore, the main objective of this study was to scrutinize the effect of different raw materials (type of defatted grape seed meal) and hydrolysis time on the composition of protein hydrolysates. An in-depth chemical characterization of the protein hydrolysates was carried out, focusing on different parameters, viz. the hydrolysis yield, the protein content, the molecular weight (MW) distribution, the colorimetric characteristics, antioxidant capacity, amino acids, and peptide profile. This study could be an important step forward in the oenology field of warm climate, since permits to establish the optima hydrolysate obtaining scheme focused on the red wine color stabilization.
2. Materials and methods
2.1. Chemicals and reagents
Endoprotease Alcalase® (subtilisin A) was acquired from Novozymes® (Bagsvaerd, Denmark), whose enzymatic activity was 2400 U/μL, using azocasein as substrate. Standards: aprotinin (Sigma-Aldrich, Madrid, Spain), cytochrome C (Panreac, Barcelona, Spain), vitamin B12 and triglycine (Alfa Aesar, MA, USA), and glycine (Thermo Fisher Scientific, MA, USA). 2,2-diphenyl-1-picrylhydrazyl (DPPH) was purchased from Alfa Aesar (Ward Hill, MA, USA). 6-Hydroxy-2,5,7,8-tetramethylchromane-2-carboxylic acid (Trolox) was acquired from Fluka (Madrid, Spain). 2,20-azobis(2-methylpropionamidine) dihydrochloride (AAPH) and fluorescein were supplied by Acros Organics (Geel, Germany).
2.2. Raw material preparation
The starting material, DGSM, was supplied by the grape pomace industry Alvinesa Natural Ingredients S.A. (Daimiel, Ciudad Real, Spain), from the processing of grape pomace from the harvest of the year 2022. Briefly, grape pomace from Vitis vinifera (L.), coming from the vinification process, arrives at the industry and is subjected to a series of washes to extract different compounds of interest (alcohol, for subsequent commercialization; tartaric salts, marketed and used as a food additive; phenols, for their use as natural pigments, for food, nutraceutical and animal products and for obtaining and commercializing tannins; etc.). Subsequently, grape pomace is de-stemmed and dried. The processing continues with the separation of grape seeds from grape pomace by densiometric tables, and subsequent milling and pelletization of grape seeds prior to the extraction of seed oil, obtaining DGSM as a residue (Mora-Garrido et al., Citation2022). Two different DGSMs were considered. The first one, from Airén white grape pomace (W) (75:25, Airén:Tempranillo), and the second one, from 100% Tempranillo red grape pomace (R). In the laboratory, DGSM was milled to pulverize to increase the surface area for a better protein extraction. DGSM was stored in darkness and at room temperature until further analysis.
2.3. Obtaining of protein hydrolysates from DGSM
To carry out the obtaining of protein hydrolysates from DGSM, the methodology developed by Cejudo-Bastante et al. (Citation2022) was applied (). First, protein extraction was performed by mixing 700 g of DGSM with 3.5 L of distilled water (20:80, (w/v)) in a 7 L bioreactor (Biorreactor Bio Console ADI 1025 Applikon Biotechnology, Inc.). The mixture was kept under constant agitation (180 rpm) and pH 10.5 for 3 h at 25 °C, and subsequently centrifuged at 9500 rpm and 4 °C for 15 min (Avanti J-26 XP Centrifuge, Beckman Coulter). The non-protein precipitate was discarded, and the supernatant was concentrated under vacuum in a rotary evaporator (70 °C). The protein concentrate remained in a water bath for 5 min at 80 °C for inactivation of its endogenous enzymes (Ghribi et al., Citation2015a). Secondly, enzymatic hydrolysis of the protein concentrate was carried out in a 3 L bioreactor (Biorreactor Bio Console ADI 1025 Applikon Biotechnology, Inc.), using 0.6 v/v Alcalase, under optimal conditions (50 °C and pH 8.5) (Cejudo-Bastante et al., Citation2022).
Two different assays were performed depending on the elapsed time of hydrolysis applied to the protein concentrate, a short duration hydrolysis (1 h, low hydrolysis, LH) and a long hydrolysis time (4 h, high hydrolysis, HH). Both types of protein hydrolysates were obtained from white (W) and red (R) DGSMs, obtaining W-LH and R-LH, and W-HH and R-HH hydrolysates, respectively. After completion of the respective hydrolysis, the enzyme was inactivated by increasing the temperature to 80 °C for 10 min. The pH of the solution was adjusted to 3.5 (usual pH of wine) with 37% HCl and centrifuged to separate the soluble (supernatant) and insoluble peptides (pellet) of each sample. The supernatant was concentrated under vacuum in a rotary evaporator at 80 °C and lyophilized for further characterization.
2.4. Protein content and protein yield of the hydrolysates
The protein yield of the hydrolysates was calculated using the equation:
The total nitrogen content of the samples was determined using the standard Kjeldahl method (AOAC, Citation1995), in a MicroKjeldahl System (J.P. Selecta, Barcelona, Spain). The factor used for the conversion of total nitrogen content to protein content was 5.75. The samples were analyzed in triplicate.
2.5. Molecular weight analysis by size-exclusion chromatography (SEC)
The method described by Bautista et al. (Citation1996) was used to determine the MW distribution by SEC. 0.2 g of the hydrolysates of the different samples (W-LH, R-LH, W-HH, and R-HH) were dissolved in 10 mL of milli-Q water and subsequently filtered through a 0.45 µm filter. A Superdex™ 30 Increase 10/300 GL column (optimum separation range 0.1-7 kDa) was used on an Agilent 1100 chromatography system equipped with a quaternary pump, an automatic injector, UV-vis diode array detector, and ChemStation software (Agilent Technologies, Palo Alto, CA, USA). A flow rate of 0.5 mL/min of Na2HPO4 50 mM (pH 7.5) was used in isocratic mode at 25 °C. A mixture of standards was used to cover the range 100–7000 Da as follows: cytochrome C, 12500 Da; aprotinin, 6500 Da; vitamin B12, 1355 Da; triglycine, 189 Da; and glycine, 75 Da. Peptide fractions were monitored at wavelengths of 215 and 280 nm and analyzed in triplicate.
2.6. Colorimetric analysis
Color of protein hydrolysates was measured in triplicate by diffuse reflectance on a CM-5® spectrophotometer (Konica Minolta, Tokyo, Japan). The CIELAB colorimetric parameters (L*, a*, b*, C*ab, and hab) were acquired from the reflectance spectra using the CIE 1964 10° Standard Observer and Standard Illuminant D65 (Commission Internationale de L'Eclairage) (CIE, Citation2004). The mathematical treatment of the data was performed using Cromalab® (Heredia et al., Citation2004). Data were obtained for the entire visible spectrum (380-770 nm). The color differences (ΔE*ab) (Euclidean distance between two points in the three-dimensional (L*, a*, b*) CIELAB space) were calculated using the following equation:
2.7. Amino acid analysis
The amino acid (AA) profile of the hydrolysates was determined by ion-exchange chromatography, and post-column derivatization using ninhydrin was carried out (Cejudo-Bastante et al., Citation2022). 6 mg of each sample were hydrolysed using 2 mL of 6 N HCl for 24 h at 110 °C. Subsequently, the pH was adjusted to 2.0 with NaOH, and afterwards, 400 µL of each sample was mixed with 100 µL of a norleucine solution (50 µM) as internal standard. Finally, solutions were filtered (0.45 µm nylon filter). An HPLC system (Biochrom 30 + Amino Acid Analyzer HPLC system Ltd., Cambridge, UK) equipped with a high-pressure PEEK cation exchange column packed with Ultropac 8 cation exchange resin and a UV–vis detector was used. The detection wavelengths were 440 and 570 nm for proline and other amino acids, respectively. The samples were measured in triplicate and the results were expressed as mg/g protein hydrolysate.
2.8. Peptide identification by LC–MS/MS
6 mg of each protein hydrolysate were dissolved in 1 mL of milli-Q water, then filtered through a 0.45 µm nylon filter and analysed by RP-LC-MS/MS on an EasynLC II system coupled to an ion trap LTQ-Orbitrap-Velos-Pro hybrid mass spectrometer (Thermo Scientific) (Cejudo-Bastante et al., Citation2022). The peptides were concentrated by reverse phase chromatography using a 0.1 mm × 20 mm C18 RP precolumn (Thermo Scientific), and then separated using a 0.075 mm × 250 mm C18 RP column (Thermo Scientific) with a flow rate of 0.3 μL/min. The duration of the chromatogram was 180 min. The gradient profile was set as follows: 5-25% solvent B for 135 min, 25-40% solvent B for 45 min, 40-100% solvent B for 2 min, and 100% solvent B for 18 min (solvent A: 0.1% formic acid in water; solvent B: 0.1% formic acid, 80% acetonitrile in water). ESI ionization was carried out using a Nano-bore emitters Stainless Steel ID 30 μm (Proxeon) interface at a sputtering voltage of 2.1 kV with a 60% S-Lens. The resolution of the Orbitrap was set to 30.000. Peptides were detected in survey scans from 400 to 1600 amu (1 μscan), followed by 20 data dependent MS/MS scans (Top 20), using an isolation width 2 u (in units of mass-to-charge ratio), normalized collision energy of 35%, and dynamic exclusion applied for periods of 60 seconds. Unassigned and singly charged protonated ions were excluded by screening for charge state. The results were matched with the UniProt database and Peaks X Pro software. Samples were analyzed in triplicate.
2.9. Antioxidant activity
2.9.1. DPPH assay
To evaluate the ability of protein hydrolysates to scavenge DPPH• radicals, the method proposed by Soler-Rivas et al. (Citation2000) was performed with some modifications. 0.02 g of each hydrolysate were dissolved in 10 mL of PBS. First, 300 μL of 108 μM DPPH• methanolic solution were added to 30 μL of sample, standard (Trolox) or 80% (v/v) methanol (blank), and the mixture was diluted with 570 μL of 80% (v/v) methanol. After 30 min under dark and at room temperature, absorbance was measured on an Agilent 8453 UV-vis spectrophotometer (Agilent Technologies, Palo Alto, CA) at 517 nm. Antioxidant activity was expressed as μmol TE (Trolox equivalents)/g of protein hydrolysate. Measurements were made in triplicate.
2.9.2. ORAC assay
The oxygen radical absorbance capacity of the samples was evaluated following the method described previously by Gillespie et al. (Citation2007). Measurements were made in triplicate using the same hydrolysate-PBS solutions as for the DPPH assay. Different dilutions of the samples were tested (1/500, 1/100, and 1/50) to obtain an appropriate concentration relative to the standard (Trolox). Black microplates were used, and 150 μL of fluorescein (80 mM) was placed in each well along with 25 μL of sample. The reaction was initiated by adding 25 μL of AAPH (140 mM) to each well after incubation for 10 min at 37 °C. Fluorescence reduction was measured by reading fluorescein excitation at 485 nm and emission at 530 nm every 5 min for 90 min. The results were expressed as μmol TE/g of protein hydrolysate.
2.10. Statistical analysis
Statistica v.8.0 software was used to perform statistical analysis of the data obtained in the different tests performed (StatSoft Inc., Citation2007). A univariate analysis of variance (ANOVA) was carried out to determine whether the mean scores of the sample data were significantly different from each other. Additionally, multiple correlation analysis and principal component analysis (PCA) were applied to evaluate if there is a relation between the assayed parameters, and to highlight the main contributors to variance between samples. Throughout the text, p values less than 0.05 (p < 0.05) denote statistically significant differences.
3. Results and discussion
3.1. Protein content and yields of the protein hydrolysates
The protein content of DGSMs from the red (R) and white (W) grape varieties was 7.35 and 10.55%, respectively (in concordance with Cejudo-Bastante et al. (Citation2022)) in similar raw materials), being significantly (p < 0.05) higher in white DGSM. The protein content of the different grape seed protein hydrolysates ranged from 58% to 70% (), similarly to that found by Cejudo-Bastante et al. (Citation2022) in similar raw material, and to seeds from other plant species, such as rice bran and sunflower (Kaewjumpol et al., Citation2018; Ugolini et al., Citation2015). However, protein content was significantly (p < 0.05) higher in the case of protein hydrolysates obtained from the DGSM of the red variety, regardless of the hydrolysis time applied ( and ). The protein yields of the hydrolysates were in agreement with the data obtained by Cejudo-Bastante et al. (Citation2022) in hydrolysates from defatted grape seed meal, and, its behavior was similar that of protein content, i.e., the hydrolysates derived from the DGSM of the red variety showed significantly (p < 0.05) higher values. According to the data on protein content and protein yield, proteins and peptides derived from the red DGSM seemed to be more hydrolysable than those from the white DGSM, thus a higher amount of soluble peptides remained in the hydrolysates. Considering that a higher protein content, a higher the probability of the peptides acting as co-pigments by binding to the wine pigments (Ozdal et al., Citation2013), the hydrolysates from the red DGSM, regardless of the hydrolysis time used, could be the most appropriate for being used in winemaking. As no previous studies about hydrolysates derived from different grape seed meal varieties have been reported, it is needed to gain insights in further parameters such as the molecular weight distribution, and the nature of peptides and amino acids, among others, of the studied hydrolysates.
Table 1. Mean ± standard deviation of hydrolysates yield (%), protein content (%), molecular weight (MW) distribution (%), CIELAB parameters (L*, C*ab, hab), and antioxidant activity by DPPH and ORAC (µmol TE/g) of the protein hydrolysates of the different assays.
Table 2. ANOVA analysis applied to all the studied parameters.
3.2. Molecular weight (MW) distribution of protein hydrolysates
shows the data on the MW distribution of the different protein hydrolysates, which was divided into three fractions, according to the size: MW larger than 5 kDa, MW between 5 and 1 kDa, and MW less than 1 kDa.
According to the ANOVA results and considering the typology of grape seed meals regardless of the hydrolysis time, the type of grape seed meal was not a determining factor, since no significant differences (p < 0.05) were found between the three MW fractions (), remaining the MW distribution pattern similar regardless of the grape variety. However, it is noteworthy that different patterns were observed between the protein hydrolysates from red and white DGSMs (). Whereas the percentage of MW > 5 kDa is, as expected, higher in W-LH compared to W-HH, contrary results were observed in R samples. This behavior could be due to an excessive hydrolysis time, which leads to exposing a large extent of hydrophobic groups, with the subsequent formation of aggregations, thus increasing the percentage of peptides with high molecular weight (Creusot & Gruppen, Citation2007; Surówka et al., Citation2004).
The excessive hydrolysis time in R samples could be supported by i) the significantly (p < 0.05) lower protein content of red DGSM compared to white DGSM. In fact, the data of MW fractions > 5 kDa of W-HH were similar to those of R-LH (), which seems to suggest that they reached the maximum degree of hydrolysis. On the other hand, ii) whereas the number of peptides with hydrophobic residues of the protein hydrolysates was similar between W-HH and W-LH (Table S1), more than 50% of the hydrophobic peptides of the protein hydrolysates from R samples were found exclusively in R-HH (Table S2), and could therefore be more susceptible to aggregation, contributing to the increase of the peptide fraction with MW > 5 kDa.
However, when the hydrolysis time factor was considered, some differences became noticeable (). As expected, the fraction with 5 > MW > 1 kDa was significantly (p < 0.05) higher in HH samples, which means that with more time of hydrolysis, new protein bands of low- and medium-MW peptides are formed (Villanueva et al., Citation1999). These findings were also obtained by Cejudo-Bastante et al. (Citation2022) when a similar starting material was hydrolyzed.
Due to the scarce information focused on the use of peptides derived from defatted grape seed meal for color stabilization in red wines, it would be advisable to consider the addition of hydrolysates of different MW to wine in order to properly understand the effects of this parameter.
3.3. Color properties
shows the values of L*, C*ab, and hab for all protein hydrolysates. a* and b* values of all hydrolysates were positive, locating them in the first quadrant of the (a*b*) plane (), similarly to those obtained Cejudo-Bastante et al. (Citation2022) from a similar starting material.
Figure 2. Representation of the protein hydrolysates obtained using different assays in the (a*b*) plane and their lightness (L*) values.

As can be seen in , the type of DGSM used was a determining factor in the color of the protein hydrolysates. Significantly (p < 0.05) higher values of all CIELAB parameters were recorded in samples obtained from white DGSM (W), being R samples darker and browner than W products, but chromatically less intense (). This may be due to the fact that, although a very low amount of residual phenols was presented in DGSM, red DGSM showed higher content than white DGSM, as stated by Mora-Garrido et al. (Citation2022). Consequently, highly colored polymers could be formed during protein extraction by co-precipitation reaction between phenolic compounds and globulin proteins (Marcone & Kakuda, Citation1999), the major protein present in grape seed (Chamizo-González et al., Citation2021). Additionally, the presence of higher amounts of phenols in R samples could lead to enzymatic browning reactions during prolonged hydrolysis, which could also explain the color of the hydrolysates (Wasswa et al., Citation2007). The brownish color of these hydrolysates could be also derived from the colorimetric properties of the meals used (red DGSM: L* 36.87 ± 1.14, C*ab 18.24 ± 0.62, and hab 52.98 ± 0.43; white DGSM: L* 34.75 ± 1.73, C*ab 17.74 ± 0.95, and hab 57.73 ± 0.60), where the aforementioned reactions have already occurred due to the industrial processing of the grape pomace. These colorimetric characteristics are similar to those obtained in other plant protein extracts (Alvarez-Ossorio et al., Citation2022; Coelho & de las Mercedes Salas-Mellado, Citation2018). The CIELAB parameters did not show a definite pattern in terms of the hydrolysis time used ( and ).
In agreement with the aforementioned results (), the analysis of the color differences according to the factors studied reveals that the color differences in the hydrolysates, according to the type DGSM used to obtain them (ΔE*ab = 30.10), are significantly (p < 0.05) more accentuated in contrast to the color differences obtained according to the hydrolysis time used (ΔE*ab = 5.01). In order to know the contribution of each attribute with respect to the total color, the quadratic differences in lightness, chroma and hue, with respect to the quadratic total color difference were calculated (Δ2L*/Δ2E*ab, Δ2C*ab/Δ2E*ab, Δ2H*ab/Δ2E*ab). Depending on the type of grape seed meal, color differences were mainly quantitative due to lightness and chroma (Δ2L* and Δ2C*ab) (49.64% and 44.21%, respectively), showing W samples higher values compared to R samples. These results suggest that the type of DGSM is the main factor influencing quantitative color attributes.
3.4. Amino acid and peptide profile
shows the results obtained for the amino acid profile of the different protein hydrolysates, including individual and total amino acids (TAA) amounts grouped into sulfurous (SAA), hydrophobic (HAA), and aromatic amino acids (AAA).
Table 3. Mean ± standard deviation of amino acids content (mg/g protein product) of the protein hydrolysates of the different assays.
In general, the predominant amino acid in all hydrolysates was Glu (negatively charged), followed by Pro (aliphatic), which constituted 35% and 18%, respectively, of the TAA content. The protein hydrolysates were also rich in Asp, Gly and Arg (19%). By families, hydrophobic amino acids (Ala, Val, Leu, Ile, Pro, Met, and Phe) constituted around 34%, and approximately 25% was attributed to aromatic amino acids (His, Phe, Pro, and Tyr). On the other hand, the minor amino acids corresponded to Thr, His, Lys, and especially the sulfur amino acids, Cys and Met. It is worth to mention that the acid hydrolysis conditions used for the determination of amino acids could provoke the degradation of Trp, and the transformation of Gln and Asn into the corresponding acids Glu and Asp (Tsugita & Scheffler, Citation1982). The amino acid patterns studied were in agreement with those reported in grape seed protein hydrolysates (Cejudo-Bastante et al., Citation2022; Ugolini et al., Citation2015).
According to the statistical analysis performed on the set of data (), the type of grape seed meal significantly (p < 0.05) influenced the amount of total, sulfur, aromatic, and hydrophobic amino acids, with a significantly (p < 0.05) lower content in the hydrolysates from red DGSM, displaying R-HH the significantly (p < 0.05) lowest values (). Same behavior was found individually with some of the amino acids (Gly, Cys, Val, Ile, Leu, His and Pro). When considering the hydrolysis time factor, only some specific significant differences (p < 0.05) were found, viz. sulfur amino acids and Gly depicted a significantly (p < 0.05) higher content after 1h-hydrolysis (LH), whereas Phe showed it after 4h-hydrolysis (HH) ( and ).
Derived from the recently reported interactions between some amino acids and malvidin-3-O-glucoside which lead to protecting and stabilizing the anthocyanins compounds related to the color of red wine (Chamizo-González et al., Citation2021, Citation2022, Citation2023), the knowledge of peptide identification and the terminal amino acids would be of special importance for discussing the effect of the addition of protein hydrolysates into red wine for the purpose of color stabilization. Amino acid sequence of peptides, mass, length, and m/z of the different protein hydrolysates, analyzed by RP-LC-MS/MS, are shown in supplementary material. For all hydrolysates, the number of amino acids in the peptide sequence ranged from 7 to 20, with MWs between 721 and 1982 Da. Similar results were recently reported in the unique reported study about protein hydrolysates of defatted grape seed meal (Cejudo-Bastante et al., Citation2022). However, no information regarding peptide sequences from different varieties of defatted grape seed meal and hydrolysis times was found.
A large number of peptides were detected in all hydrolysates, and although the proteins they come from differed qualitatively, the hydrolysates had in common five proteins ascribed to Vitis vinifera (F6HZK3, F6HZK2, F6HI56, A5C7L5, and D7U302). As mentioned above, the hydrolysates distinguished a considerable percentage of Asp, Phe, Asn, Pro, Ser, and Arg. This fact is of special relevance since these major amino acids (Gly, Gln, Phe, Asn, Arg, and Ser) has been recently reported to interact with malvidin-3-glucoside, the main anthocyanin responsible for the color of red wine (Chamizo-González et al., Citation2021, Citation2022, Citation2023), both by hydrogen bond, Van der Waals Forces, π-π and alkyl hydrophobic interactions depending on the amino acid, potentially acting as co-pigments, thus protecting and stabilizing the color of red wine. Furthermore, those major amino acids played an important role in the terminal positions of the peptide sequence, since Gln, Gly and Glu were mainly sit at the N-terminal of the sequence, and Arg and Gln, followed by Glu, Gly, and Phe were at the C-terminal of the peptide sequence (see supplementary material). These results are in concordance with those obtained by Ghribi et al. (Citation2015b), who found that Alcalase hydrolyses proteins with a high specificity for peptide bonds, preferentially for a large uncharged residue such as Gln and Asn. The position of the amino acids in the peptide sequence could influence the antioxidant activity of the peptide. In particular, the amino acids Arg, Asp and Gly located at the C-terminal of the peptide sequence have been shown to confer high antioxidant activity (Ghribi et al., Citation2015b; Guo et al., Citation2009).
Considering the variability factors, i.e., the type of grape seed meal and the time of hydrolysis, many differences were found among the samples in the peptide sequence. Regarding W hydrolysates, the number of amino acids in the peptide sequence ranged from 7 to 19, with MWs between 721 and 1971 Da. Both W hydrolysates (HH and LH) shared 6 proteins (F6HZK3, F6HZK2, F6HI56, A5C7L5, D7U302 and A5AEI7), although W-HH also exhibited additional peptides derived from the F6HEP7 and A5ASF5 proteins. Cejudo-Bastante et al. (Citation2022) found similar results in hydrolysates from a similar grape seed meal, although a higher number of peptides and proteins have been identified in the present study (Table S1). Concretely, in agreement with the MW fractions, W-HH showed a greater number of peptides than W-LH, with some specific peptides identified in each of them (Table S1). In addition, the amino acids at the N-terminal showed a higher percentage of Glu, following by Asp, Lys, Leu, and Asn, and lower percentages of Gly and Gln in W-HH compared with W-LH, concluding that W-HH provides a more varied qualitative profile at the N-terminal of the sequence than W-LH. Similarly, W-HH showed a higher percentage of Glu and Arg, and the minor Leu, Asp, and Tyr at the C-terminal in the sequence, but a lower percentage of Gln, Phe, and Val.
Regarding R hydrolysates, the number of amino acids in the peptide sequence ranged from 7 to 20, with MWs between 745.4 and 1981.8 Da. Both R-HH and R-LH shared 12 proteins (F6HZK2, F6HZK3, F6HI56, Q9M4H7, D7U302, F6I0M9, F6H3T7, D7T227, C5DB50, A5C7L5, F6HI57, and F6GTY5). However, and contrarily to the behavior of W hydrolysates, the number of peptides, and the proteins from which they are derived, are considerably predominant in R-LH, consistent with the MW results. The amino acids at the N-terminal of the peptide sequence showed a higher contribution of Ala, Glu, and Ser in R-HH and remarkably lower influence of Gly. Among the minor N-terminal amino acids, higher values of Thr and Val, and lower of Arg were also found. At the C-terminal, the major amino acids were remarkably higher in R-HH (Ala, Glu, Gly, and Arg), in detriment of the lower percentage of the minor Asp, Lys, Leu, Met, and Asn. Similarly, to the W hydrolysates, both R samples shared many peptides, but R-HH and R-LH also had their specific peptides (Table S2).
Although many of the peptides were shared by pairs of samples (W-HH and R-HH; W-LH and R-LH), the hydrolysis time did not show a particular pattern, as the peptide sequences were more dependent on the type of starting raw material.
3.5. Antioxidant activity
Some authors affirmed that proteins increase the antioxidant capacity of wine, and thus the stability of anthocyanins preventing their degradation (Chamizo-González et al., Citation2021; Gordillo et al., Citation2021). In addition, protein hydrolysates and peptide fractions can be added as functional antioxidant ingredients in food systems to reduce oxidative changes (Elias et al., Citation2008; Kumar et al., Citation2022; Nunes et al., Citation2021). Therefore, the presence of proteins and peptides are closely related to the antioxidant properties, thus could positively affect red wine color stabilization. Therefore, regarding the present study, it is important to establish the values of antioxidant activity of the protein hydrolysates that will be further added into red wine with that purpose, which have not been previously reported in protein hydrolysates from grape seeds.
exhibits the values of antioxidant activity by DPPH and ORAC of the different protein hydrolysates. Peptides with a length ranged between 2 and 20 amino acid residues, as that present the protein hydrolysates of this work, have been described as bioactive peptides (Ghribi et al., Citation2015b).
According to the data obtained, the type of grape seed meal did not influence significantly (p < 0.05) the antioxidant capacity by DDPH (), but significant (p < 0.05) differences were observed by ORAC. Therefore, it seems that protein hydrolysates from DGSM by-product did not function by donating electrons to the free radicals (DPPH), but through a hydrogen atom transfer-based (ORAC) (López-Pedrouso et al., Citation2023). The antioxidant activity was significantly (p < 0.05) lower for the protein hydrolysates from the red DGSM (R-HH and R-LH) (), fact that could be because they contained a significantly (p < 0.05) lower concentration of aromatic (His and Pro) and basic and positively charged (His) amino acids (Nimalaratne et al., Citation2011; Zou et al., Citation2016). Furthermore, although the R samples exhibited a broader peptide profile (Table S2), their peptides showed a lower presence of basic amino acids in the terminal positions, such as Lys and Arg, a fact that could contribute to their lower antioxidant capacity (Nimalaratne et al., Citation2011). According to the obtained data on antioxidant activity, the addition of hydrolysates from white DGSM to wine could be a priori more favorable, since could improve its antioxidative capacity. Hydrolysis time did not significantly influence the antioxidant capacity by DPPH or ORAC ( and ).
In order to test the relationship between the different amino acids and the antioxidant capacity of the protein hydrolysates, multiple correlations were carried out. Data revealed a direct correlation (R2 = 0.9320) between the antioxidant capacity (ORAC) and the content of SAA (Met and Cys) and HAA (Ala, Val, Leu, Ile, Pro, Met and Phe), being Cys the significantly (p < 0.05) most influential amino acid on this correlation. Several authors also found the remarkable antioxidant capacity of this amino acid (Esfandi et al., Citation2019; Górska-Warsewicz et al., Citation2018).
To our knowledge, no studies have been found on the antioxidant activity of grape seed meal protein hydrolysates, so this study could provide information of great interest for scrutinizing the antioxidant pattern of red wines after the addition of protein hydrolysates.
3.6. PCA analysis
An unsupervised pattern recognition statistical analysis (principal component analysis, PCA) was performed to determine the main parameters responsible for the differences between the protein hydrolysates from different grape varieties and hydrolysis times ().
Three significant principal components (PC) were identified using Kaiser’s criterion (eigenvalues >1), which explained 100% of the total variance. shows the samples to the plane defined by the first three principal components (PCs). PC-1 divided the samples according to the type of grape seed meal used to obtain the protein hydrolysates. R-HH and R-LH were located on the positive side of the axis and had inferior scores to those of W-HH and W-LH for almost all parameters, except for the hydrolysate yield and protein percentages. PC-2 separated samples by their molecular weight pattern and ORAC, distinguishing W-LH from the rest by their higher content of larger peptides (> 5 kDa). PC-3 divided the samples according to the hydrolysis time. R-LH and W-LH obtained negative values, mainly due to the type of amino acid (totals, aromatics, hydrophobic and sulfur), the percentage of proteins, and the low MW fraction.
4. Conclusions
The defatted grape seed meal from industrial grape pomace processing is presenting an emerging alternative for the oenology industry as a source of non-animal proteins. Knowledge of the chemical, colorimetric, and antioxidant properties of protein hydrolysates and how they influence depending on the type of DGSM and obtaining methodology leads us to better choose the type of product depending on the intended purpose. This study is the first attempt to characterize protein hydrolysates of different molecular weights from different DGSMs in-depth, with the end of using them as color-stabilizing agents for warm climate red wines. Based on the results of all the studied parameters, both the hydrolysates obtained from red and white DGSM after 1 h of hydrolysis time have been selected as optimum.
In light of the promising results, the effectiveness of the addition of these protein hydrolysates should be tested in winemaking as a new oenological strategy and further comprehensively evaluate their effect on the colorimetric stabilization of red wines from warm climates, the great challenge wine research typically affected by color loss.
Author contributions
Ana Belén Mora-Garrido: Investigation, Writing – original draft. M. Luisa Escudero-Gilete: Writing – original draft, Writing – review & editing, Funding acquisition. Francisco J. Heredia: Conceptualization, Supervision, Funding acquisition. María Jesús Cejudo-Bastante: Supervision, Writing - review & editing.
Supplemental Material
Download MS Word (89.9 KB)Supplemental Material
Download MS Word (57.5 KB)Acknowledgments
The authors thank the technical staff of the Biology Service (Servicios Generales de Investigación (SGI), Universidad de Sevilla. The authors also thank Alvinesa Natural Ingredients, S.A (Daimiel, Ciudad Real, Spain) for supplying the by-product samples.
Disclosure statement
No potential conflict of interest was reported by the author(s).
Data availability
The authors confirm that the data supporting the findings of this study are available within the article and its supplementary materials.
Additional information
Funding
Notes on contributors
Ana Belén Mora-Garrido
Ana Belén Mora-Garrido is a predoctoral student at the Department of Nutrition and Bromatology of the University of Seville, being part of the research group AGR-225 “Color and Food Quality”. The research line of her thesis deals with the use of by-products of the winemaking industry to improve the organoleptic qualities of wines produced in warm climates.
References
- Adamson, N. J., & Reynolds, E. C. (1996). Characterization of casein phosphopeptides prepared alcalase: Determination of enzyme specificity. Enzyme and Microbial Technology, 19(3), 1–14. https://doi.org/10.1016/0141-0229(95)00232-4
- Alvarez-Ossorio, C., Orive, M., Sanmartín, E., Alvarez-Sabatel, S., Labidi, J., Zufia, J., & Bald, C. (2022). Composition and techno-functional properties of grape seed flour protein extracts. ACS Food Science & Technology, 2(1), 125–135. https://doi.org/10.1021/acsfoodscitech.1c00367
- AOAC. (1995). Official methods of analysis (16th ed.). Association of Official Analytical Chemists.
- Bautista, J., Hernandez-Pinzon, I., Alaiz, M., Parrado, J., & Millan, F. (1996). Low molecular weight sunflower protein hydrolysate with low concentration in aromatic amino acids. Journal of Agricultural and Food Chemistry, 44(4), 967–971. https://doi.org/10.1021/jf940726c
- Bordiga, M. (2018). Post-fermentation and distillation technology: Stabilization, aging, and spoilage. CRC Press.
- Boulton, R. (2001). The copigmentation of anthocyanins and its role in the color of red wine: A critical review. American Journal of Enology and Viticulture, 52(2), 67–87. https://doi.org/10.5344/ajev.2001.52.2.67
- Bučko, S., Katona, J., Petrović, L., Milinković, J., Spasojević, L., Mucić, N., & Miller, R. (2018). The influence of enzymatic hydrolysis on adsorption and interfacial dilatational properties of pumpkin (Cucurbita pepo) seed protein isolate. Food Biophysics. 13(3), 217–225. https://doi.org/10.1007/s11483-018-9528-5
- Cejudo-Bastante, M. J., Oliva-Sobrado, M., González-Miret, M. L., & Heredia, F. J. (2022). Optimisation of the methodology for obtaining enzymatic protein hydrolysates from an industrial grape seed meal residue. Food Chemistry, 370, 131078. https://doi.org/10.1016/j.foodchem.2021.131078
- Cejudo-Bastante, M. J., Rivero-Granados, F. J., & Heredia, F. J. (2017). Improving the color and aging aptitude of Syrah wines in warm climate by wood–grape mix maceration. European Food Research and Technology, 243(4), 575–582. https://doi.org/10.1007/s00217-016-2767-0
- Cejudo-Bastante, M. J., Rodríguez-Morgado, B., Jara-Palacios, M. J., Rivas-Gonzalo, J. C., Parrado, J., & Heredia, F. J. (2016). Pre-fermentative addition of an enzymatic grape seed hydrolysate in warm climate winemaking. Effect on the differential colorimetry, copigmentation and polyphenolic profiles. Food Chemistry, 209, 348–357. https://doi.org/10.1016/j.foodchem.2016.04.092
- Chamizo-González, F., Estévez, I. G., Gordillo, B., Manjón, E., Escribano-Bailón, M. T., Heredia, F. J., & González-Miret, M. L. (2023). First insights into the binding mechanism and colour effect of the interaction of grape seed 11S globulin with malvidin 3-O-glucoside by fluorescence spectroscopy, differential colorimetry and molecular modelling. Food Chemistry, 413, 135591. https://doi.org/10.1016/j.foodchem.2023.135591
- Chamizo-González, F., Gordillo, B., & Heredia, F. J. (2021). Elucidation of the 3D structure of grape seed 7S globulin and its interaction with malvidin 3-glucoside: A molecular modeling approach. Food Chemistry, 347, 129014. https://doi.org/10.1016/j.foodchem.2021.129014
- Chamizo-González, F., Heredia, F. J., Rodríguez-Pulido, F. J., González-Miret, M. L., & Gordillo, B. (2022). Proteomic and computational characterisation of 11S globulins from grape seed flour by-product and its interaction with malvidin 3-glucoside by molecular docking. Food Chemistry, 386, 132842. https://doi.org/10.1016/j.foodchem.2022.132842
- CIE. (2004). Technical report colorimetry. Commission Internationale de l’Eclairage Central Bureau.
- Coelho, M. S., & de las Mercedes Salas-Mellado, M. (2018). How extraction method affects the physicochemical and functional properties of chia proteins. LWT, 96, 26–33. https://doi.org/10.1016/j.lwt.2018.05.010
- Creusot, N., & Gruppen, H. (2007). Hydrolysis of whey protein isolate with Bacillus licheniformis protease: Fractionation and identification of aggregating peptides. Journal of Agricultural and Food Chemistry, 55(22), 9241–9250. https://doi.org/10.1021/jf071584s
- Dhanasekar, S., & Sathyanathan, R. (2023). Bioenergy potential of Chlorella vulgaris under the influence of different light conditions in a bubble column photobioreactor. Global J. Environ. Sci. Manag, 9(4), 789–804. https://doi.org/10.22034/gjesm.2023.04.09
- Dwyer, K., Hosseinian, F., & Rod, M. (2014). The market potential of grape waste alternatives. Journal of Food Research, 3(2), 91–106. https://doi.org/10.5539/jfr.v3n2p91
- Elias, R. J., Kellerby, S. S., & Decker, E. A. (2008). Antioxidant activity of proteins and peptides. Critical Reviews in Food Science and Nutrition, 48(5), 430–441. https://doi.org/10.1080/10408390701425615
- Esfandi, R., Walters, M. E., & Tsopmo, A. (2019). Antioxidant properties and potential mechanisms of hydrolyzed proteins and peptides from cereals. Heliyon, 5(4), e01538. https://doi.org/10.1016/j.heliyon.2019.e01538
- García-Lomillo, J., & González-SanJosé, M. L. (2017). Applications of wine pomace in the food industry: Approaches and functions. Comprehensive Reviews in Food Science and Food Safety, 16(1), 3–22. https://doi.org/10.1111/1541-4337.12238
- Gazzola, D., Vincenzi, S., Marangon, M., Pasini, G., & Curioni, A. (2017). Grape seed extract: The first protein-based fining agent endogenous to grapes. Australian Journal of Grape and Wine Research. 23(2), 215–225. https://doi.org/10.1111/ajgw.12268
- Ghribi, A. M., Sila, A., Przybylski, R., Nedjar-Arroume, N., Makhlouf, I., Blecker, C., Attia, H., Dhulster, P., Bougatef, A., & Besbes, S. (2015b). Purification and identification of novel antioxidant peptides from enzymatic hydrolysate of chickpea (Cicer arietinum L.) protein concentrate. J. Funct. Foods, 12, 516–525. https://doi.org/10.1016/j.jff.2014.12.011
- Ghribi, A., Maklouf Gafsi, I., Sila, A., Blecker, C., Danthine, S., Attia, H., Bougatef, A., & Besbes, S. (2015a). Effects of enzymatic hydrolysis on conformational and functional properties of chickpea protein isolate. Food Chemistry, 187, 322–330. https://doi.org/10.1016/J.FOODCHEM.2015.04.109
- Gillespie, K. M., Chae, J. M., & Ainsworth, E. A. (2007). Rapid measurement of total antioxidant capacity in plants. Nature Protocols, 2(4), 867–870. https://doi.org/10.1038/nprot.2007.100
- Gordillo, B., Cejudo-Bastante, M. J., Rodríguez-Pulido, F. J., Jara-Palacios, M. J., Ramírez-Pérez, P., González-Miret, M. L., & Heredia, F. J. (2014). Impact of adding white pomace to red grapes on the phenolic composition and color stability of syrah wines from a warm climate. Journal of Agricultural and Food Chemistry, 62(12), 2663–2671. https://doi.org/10.1021/jf405574x
- Gordillo, B., Chamizo-González, F., González-Miret, M. L., & Heredia, F. J. (2021). Impact of alternative protein fining agents on the phenolic composition and color of Syrah red wines from warm climate. Food Chemistry, 342, 128297. https://doi.org/10.1016/j.foodchem.2020.128297
- Górska-Warsewicz, H., Laskowski, W., Kulykovets, O., Kudlińska-Chylak, A., Czeczotko, M., & Rejman, K. (2018). Food products as sources of protein and amino acids—The case of Poland. Nutrients, 10(12), 1977. https://doi.org/10.3390/nu10121977
- Guo, H., Kouzuma, Y., & Yonekura, M. (2009). Structures and properties of antioxidative peptides derived from royal jelly protein. Food Chemistry. 113(1), 238–245. https://doi.org/10.1016/j.foodchem.2008.06.081
- Heredia, F. J., Álvarez, C., González-Miret, M. L., & Ramírez, A. (2004). Registro General de la Propiedad Intelectural (SE-1052-04).
- Kaewjumpol, G., Oruna-Concha, M. J., Niranjan, K., & Thawornchinsombut, S. (2018). The production of hydrolysates from industrially defatted rice bran and its surface image changes during extraction. Journal of the Science of Food and Agriculture, 98(9), 3290–3298. https://doi.org/10.1002/jsfa.8832
- Kumar, M., Tomar, M., Punia, S., Dhakane-Lad, J., Dhumal, S., Changan, S., Senapathy, M., Berwal, M. K., Sampathrajan, V., Sayed, A. A. S., Chandran, D., Pandiselvam, R., Rais, N., Mahato, D. K., Udikeri, S. S., Satankar, V., Anitha, T., Singh, S., Amarowicz, R., & Kennedy, J. F. 2022. Plant-based proteins and their multifaceted industrial applications. LWT, 154, 112620. https://doi.org/10.1016/j.lwt.2021.112620
- López-Pedrouso, M., Lorenzo, J. M., Bou, R., Vazquez, J. A., Valcarcel, J., Toldrà, M., & Franco, D. (2023). Valorisation of pork by-products to obtain antioxidant and antihypertensive peptides. Food Chemistry, 423, 136351. https://doi.org/10.1016/j.foodchem.2023.136351
- Ma, Y., Zhao, H., & Zhao, X. (2019). Comparison of the effects of the alcalase- hydrolysates of caseinate, and of fish and bovine gelatins on the acidification and textural features of set-style skimmed yogurt- type products. Foods (Basel, Switzerland), 8(10), 501. https://doi.org/10.3390/foods8100501
- Maier, T., Schieber, A., Kammerer, D. R., & Carle, R. (2009). Residues of grape (Vitis vinifera L.) seed oil production as a valuable source of phenolic antioxidants. Food Chemistry. 112(3), 551–559. https://doi.org/10.1016/j.foodchem.2008.06.005
- Marangon, M., Vincenzi, S., & Curioni, A. (2019). Wine fining with plant proteins. Molecules (Basel, Switzerland), 24(11), 2186. https://doi.org/10.3390/molecules24112186
- Marcone, M. F., & Kakuda, Y. (1999). A comparative study of the functional properties of amaranth and soybean globulin isolates. Nahrung/Food, 43(6), 368–373.
- Mira de Orduña, R. (2010). Climate change associated effects on grape and wine quality and production. Food Research International, 43(7), 1844–1855. https://doi.org/10.1016/j.foodres.2010.05.001
- Mora-Garrido, A. B., Cejudo-Bastante, M. J., Heredia, F. J., & Escudero-Gilete, M. L. (2022). Revalorization of residues from the industrial exhaustion of grape by-products. LWT, 156, 113057. https://doi.org/10.1016/j.lwt.2021.113057
- Nimalaratne, C., Lopes-Lutz, D., Schieber, A., & Wu, J. (2011). Free aromatic amino acids in egg yolk show antioxidant properties. Food Chemistry. 129(1), 155–161. https://doi.org/10.1016/j.foodchem.2011.04.058
- Nunes, L. G. P., Reichert, T., & Machini, M. T. (2021). His-rich peptides, gly- and his-rich peptides: Functionally versatile compounds with potential multi-purpose applications. International Journal of Peptide Research and Therapeutics, 27(4), 2945–2963. https://doi.org/10.1007/s10989-021-10302-z
- Ozdal, T., Capanoglu, E., & Altay, F. (2013). A review on protein–phenolic interactions and associated changes. Food Research International. 51(2), 954–970. https://doi.org/10.1016/j.foodres.2013.02.009
- Parrado, J., Miramontes, E., Jover, M., Gutierrez, J. F., Collantes de Terán, L., & Bautista, J. (2006). Preparation of a rice bran enzymatic extract with potential use as functional food. Food Chemistry. 98(4), 742–748. https://doi.org/10.1016/j.foodchem.2005.07.016
- Pojić, M., Mišan, A., & Tiwari, B. (2018). Eco-innovative technologies for extraction of proteins for human consumption from renewable protein sources of plant origin. Trends in Food Science and Technology. 75, 93–104. https://doi.org/10.1016/j.tifs.2018.03.010
- Samimi, M., & Shahriari-Moghadam, M. (2023). The Lantana camara L. stem biomass as an inexpensive and efficient biosorbent for the adsorptive removal of malachite green from aquatic environments: Kinetics, equilibrium and thermodynamic studies. International Journal of Phytoremediation, 25(10), 1328–1336. https://doi.org/10.1080/15226514.2022.2156978
- Soler-Rivas, C., Espín, J. C., & Wichers, H. J. (2000). An easy and fast test to compare total free radical scavenger capacity of foodstuffs. Phytochemical Analysis, 11(5), 330–338.
- StatSoft Inc. (2007). STATISTICA (data analysis software system). Version 8. www.statsoft.com.
- Suarez, L. M., Salcedo, J. G., & Zapata, J. E. (2022). Biological activity of Venezuelan variety cassava leaf hydrolyzates obtained by using different microbial enzymes. Information Technology, 33(2), 77–88. https://doi.org/10.4067/s0718-07642022000200077
- Surówka, K., Żmudziński, D., & Surówka, J. (2004). Enzymic modification of extruded soy protein concentrates as a method of obtaining new functional food components. Trends in Food Science and Technology. 15(3–4), 153–160. https://doi.org/10.1016/j.tifs.2003.09.013
- Tschiersch, C., Nikfardjam, M. P., Schmidt, O., & Schwack, W. (2010). Degree of hydrolysis of some vegetable proteins used as fining agents and its influence on polyphenol removal from red wine. European Food Research and Technology, 231(1), 65–74. https://doi.org/10.1007/s00217-010-1253-3
- Tsugita, A., & Scheffler, J. J. (1982). A rapid method for acid hydrolysis of protein with a mixture of trifluoroacetic acid and hydrochloric acid. European Journal of Biochemistry, 124(3), 585–588. https://doi.org/10.1111/j.1432-1033.1982.tb06634.x
- Ugolini, L., Cinti, S., Righetti, L., Stefan, A., Matteo, R., D’Avino, L., & Lazzeri, L. (2015). Production of an enzymatic protein hydrolyzate from defatted sunflower seed meal for potential application as a plant biostimulant. Industrial Crops and Products. 75, 15–23. https://doi.org/10.1016/j.indcrop.2014.11.026
- Villanueva, A., Clemente, A., Bautista, J., & Millán, F. (1999). Production of an extensive sunflower protein hydrolysate by sequential hydrolysis with endo- and exo-proteases. Grasas y Aceites, 50(6), 472–476. https://doi.org/10.3989/gya.1999.v50.i6.697
- Wasswa, J., Tang, J., Gu, X. H., & Yuan, X. Q. (2007). Influence of the extent of enzymatic hydrolysis on the functional properties of protein hydrolysate from grass carp (Ctenopharyngodon idella) skin. Food Chemistry. 104(4), 1698–1704. https://doi.org/10.1016/j.foodchem.2007.03.044
- Zhao, G., Liu, Y., Zhao, M., Ren, J., & Yang, B. (2011). Enzymatic hydrolysis and their effects on conformational and functional properties of peanut protein isolate. Food Chemistry. 127(4), 1438–1443. https://doi.org/10.1016/j.foodchem.2011.01.046
- Zou, Y., Wang, W., Li, Q., Chen, Y., Zheng, D., Zou, Y., Zhang, M., Zhao, T., Mao, G., Feng, W., Wu, X., & Yang, L. (2016). Physicochemical, functional properties and antioxidant activities of porcine cerebral hydrolysate peptides produced by ultrasound processing. Process Biochemistry. 51(3), 431–443. https://doi.org/10.1016/j.procbio.2015.12.011