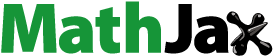
ABSTRACT
Pre-stressing steels used in pre-stressed concrete undergo degradation when exposed to a corrosive environment resulting in eroded mass. This paper presents the experimental results of tensile strength variation of prestressing strand with respect to section loss. Surface morphology, corrosion type and mode of rust deposition under the influence of accelerated corrosion were also investigated. 12.7 mm seven wire strands of 1860MPa characteristic strength were used for the study in comparison with non-corroded strand as control specimens. The variation of above-mentioned factors was initially compared on the basis of target weight loss in the range of 0.1%, 0.5% and 1%. It is observed from the accelerated corrosion that hydrogen embrittlement mechanism occurred resulting in shallow and wide eroded mass in the form of uniform corrosion occurring with increase in the corrosion rate. Results were more pronounced for 1% weight loss with 36% drop in tensile strength. The paper concludes that accelerated corrosion is a widely chosen procedure by researchers to validate the study on corrosion as it can be achieved in a shorter time period and this method was effectively utilized in the study of relation between loss of weight due to corrosion and tensile strength.
Introduction
Prestressed concrete is a type of concrete which counteracts the external loads with the internal compressive actions. Prestressing steel is a high-strength steel which transfers compressive stress to the surrounding concrete through the bond between them. Chemical composition of it includes continuous cast steel with high carbon and manganese with limited amounts of sulphur and phosphorus. This cold drawn high strength prestressing steel is individually available in the form of wire, bar and strand. Similar to conventional steel these high-strength steel also suffer corrosion passivation offered by concrete alkalinity plays a major role against deterioration (Rajbanul Akhond et al., Citation2023). On comparison of conventional steel with prestressing steel the latter is subjected to higher stresses and catastrophic failure is expected due to corrosion. Consequences of corrosion of prestressing steel are considered detrimental as it results in loss of bond, reduction in cross-sectional area, mechanical property degradation and ductility drop (Andrade, Citation2017; Belletti et al., Citation2020; Li et al., Citation2011; Menoufy & Soudki, Citation2014). Many insights are available regarding fundamental mechanisms involved in steel reinforcement corrosion where presence of pozzolans in concrete is said to decrease the rate of corrosion (Tran et al., Citation2023). In conventional concrete deposition of rust on steel exerts expansive pressure on surrounding concrete which results in cracking, delamination and spalling of concrete cover. Analytical study by Arunkumar et al. (Citation2023) revealed corrosion-based deterioration in conventional reinforcement affected bond performance. The predicted conditions were verified with experimental results conveying the risks associated with structural damage due to steel corrosion.
Durability deterioration is a major concern in prestressed concrete. Corrosion naturally occurs as electrochemical reactions involving anodic and cathodic reactions where oxidation and reduction take place respectively. Uniform general corrosion does not affect the prestressing steel to a higher extent but local corrosion attack with sectional area loss is the major concern when exposed to marine environments. The capacity of prestressing steel to sustain the damage is to the level of four to five times that of conventional bars due to its pronounced strength features. Loss of cross-sectional area in prestressing steels due to general or pitting corrosion is detrimental. Throughout its life steel experiences stress level in the range of 55%-65% of that of its ultimate tensile strength which can temporarily surpass to higher levels at the time of prestressing. Local yielding and fracture are expected when the net stress in the steel increases as a result of material loss due to corrosion.
Strands used to transfer pre-stress undergo corrosion in an aggressive environment. Water containing chlorides coming in contact with the steel surface through un tight joints and concrete pores leads to localized corrosion (Nürnberger, Citation2002). Strands are used both in pre and post tension applications. They are more sensitive to corrosion due to their high strength (Nürnberger, Citation2002) leading to serious consequences like catastrophic failure. Corrosion as sectional loss is quicker and increase in net stress leads to local yielding and failure. Rengaraju et al. (Citation2020) observed that strand embedded in concrete did not show visible signs up to 6% cross sectional area loss. Dai et al. (Citation2020) observed circumferential stress is induced in steel concrete interface due to corrosion and when this exceeds tensile strength of concrete cracking occurs. Chai et al. (Citation2021) achieved three corrosion levels (1%,3% and 5%) by immersion of metal in aqueous solution both oxidation and reduction mechanism occurred until an equilibrium was reached. The specimens were simultaneously subjected to sustained loads (25%,45% and 65%) after corrosion induction. 1% corrosion level led to 1.22 times increase in bond strength due to the friction that developed between bar and concrete compared to control specimen, while obvious trend of bond strength drop was observed for higher level of corrosion. Bond between strand and concrete can also be affected by accumulation of aggregates at the beam top during casting of self -consolidating concrete (Sadhasivam et al., Citation2017). The significant cause of strand failure was considered as the corrosion loss of single wire with respect to that of average corrosion in wires by Giriraju et al. (Citation2022). Li et al. (Citation2011) studied the surface characteristics of rebar and found that there existed differences in the course of occurrence as well as surface distribution of corrosion. Corrosion was pronounced on the side of rebar facing concrete cover in natural corrosion while bar surface was corroded in the case of accelerated corrosion. Menoufy and Soudki (Citation2014) mentioned the chances of result misinterpretation in induced corrosion as different degrees of intended corrosion were tried to be induced in the same amount of time. Saraswathy et al. (Citation2018) and Song et al. (Citation2020) mentioned that the corrosion rate of prestressing steel is greater than that of conventional rebar. Jeon et al. (Citation2019), evaluated spring model with 7 springs representing wires of 7 wired strand and concluded that the rupture of highly corroded wire represents the failure of the strand. Vecchi et al. (Citation2021) studied 10 year old prestressed concrete beams and concluded that under natural exposure conditions pitting corrosion occurs in pre-stressing steels. Wang et al. (Citation2020) studied corroded strands in artificial climate chamber. Strands were classified based on initial stressing level which varied from 45% to 75% of yield strength. The higher level of stress promoted propagation of micro cracks which affected ultimate strength and had little effect on modulus of elasticity. Study by Vu et al. (Citation2009) revealed that micro cracks reduce the ultimate strain of strand. Study by Vélez et al. (Citation2016) indicated occurrence of pitting corrosion in highly stressed steel. Li et al. (Citation2011) studied the effect of the level of prestressing on corrosion in strands and found a linear relation between both. Strands were prestressed in the range of 0.3, 0.5 and 0.7 times that of characteristic strength of strand. Since the quality of strands was superior to that of regular reinforcement pitting and general corrosion were observed. Accelerated method based on migration was confirmed as the best method to initiate corrosion. Hansson (Citation2016) mentioned that stress corrosion cracking is a matter of concern in the case of pre stressing steel. Lifespan of the metallic structure is determined based on corrosion rate. Cui et al. (Citation2022) explored the deterioration characteristics that were observed after aging of concrete using freeze-thaw cycle and corrosion media was chosen as 5% sodium chloride solution and corrosion current density was maintained at the range of 0.967–0.983 mA/cm2. Slight corrosion improved interface bonding between reinforcement and concrete. On coupling of these two-aging process, they tend to promote each other. 86.11% drop was observed in cracking load whereas 57.04% drop in ultimate load was encountered revealing the differed effect of aging on mechanical behaviour of prestressed beams. Campione and Zizzo (Citation2022) numerically simulated the flexural behaviour of prestressed beams with corroded strand and revealed failure mode and load bearing capacity are severely affected. Steel area reduction due to pitting, dropping of yield stress and ultimate strain and loss of bonding between concrete and strand are considered as factors of strand corrosion affecting flexural behaviour. It is observed that prestressed concrete failed due to crushing in the compression zone when corrosion levels were below 10% and above this value girder – deck system exhibited rupture failure of strands. Su et al. (Citation2022) investigated the coupled effect of strand corrosion and fatigue loads on performance degradation of prestressed concrete. Beams were subjected to accelerated corrosion in 5% sodium chloride solution as electrolyte with 250 μA/cm2 current density. Uncorroded beams exhibited brittle failure of steel bar (longitudinal), whereas corroded beam under faitigue exhibited brittle failure of strands followed by steel bar brittle failure
The most accepted method to measure the corrosion rate is the gravimetric method where samples are exposed to a corrosive environment, later weight loss is measured with respect to time. Corrosion rate exhibits the corrosion vulnerability of metals. Corrosion rate is influenced by oxygen concentration, resistivity changes and temperature. Study of local characteristics of corrosion initiations will provide information regarding steel concrete interface in corroded structures (Angst, Citation2018). It’s a well-known fact that the behaviour of strands is governed by most corroded wire with a smaller area (Jeon et al., Citation2019). Though the fundamental corrosion mechanism of pre stressing steel is similar to that of conventional reinforcement their mode of occurrence is different. Usually, brittle failure occurs in pre-stressed steel due to corrosion hence there is a necessity to collect more information regarding the same. Large number of research findings are available on corrosion of conventional steel reinforcements. However, there is a need for study on durability of pre-stressing steel in corrosive environments as there is less visible warning before catastrophic failures. Lab simulated chloride environment is a common method utilized by researchers for corrosion study. Corrosion induction by applying current is considered as an effective method to induce accelerated corrosion (Li et al., Citation2011). Trejo et al. (Citation2009) experimentally showed chloride concentration, void type and stress levels are statistically significant parameters governing corrosion.
Research Significance
It is observed that previous studies have focused mainly on stressed strand corrosion and the output is highly affected due to stressing (Franceschini et al., Citation2022; Li et al., Citation2022; Vecchi et al., Citation2021; Wang et al., Citation2020). Corrosion media concentration influences the extent of corrosion-based mass loss and cross-section area loss which in turn significantly influence the residual prestressing force. Study by Li et al. (Citation2023) observed two stages in degradation of prestressing force. In first stage under stressed condition linear drop with respect to mass loss was observed and second stage on reaching 40% area loss of wire in strand was observed. It is evident from the previous studies that individual wires of strand (under stressed condition) with small diameter ranging from 3 mm to 5 mm when subjected to corrosion will quickly experience structural deficiencies. Present work intended to provide insight pertaining to effect of corrosion on strand excluding the effect of applied stress as confounding factor. It was found that despite of absence of applied stress the corrosion impacted mechanical performance severely thus making the observations relevant for pre-consideration in design of durable structures. Further, study established the correlation between weight loss and subsequent drop in tensile strength which added practical dimension to the study as weight loss is both observable and measurable parameter which serves as a potential indicator of structural deterioration. The severity of corrosion impact was quantified in terms of weight loss v/s tensile strength drop along with that the classification of impact as triggering, alarming and detrimental adds to the qualitative assessment. The study has practical implications for durability and maintenance aspects which make the observations serve as valuable information to maintenance engineers and stakeholders involved in infrastructural projects.
Overall the present study on prestressing strands contributes to the maintenance programs by engineers and future researchers. Firstly, results of unstressed strand corrosion can be used as a base for economically estimating the capacity of stressed strands embedded within concrete with chloride exposure conditions. As it is a well-known fact that the stressed samples exhibit quicker pace of capacity losses compared to unstressed samples. Secondly, present study results reassured the facts that despite the good quality of steel material loss plays a critical role on tensile strength.
Corrosion in general implies loss of material with drop in strength, pre-stressing applications are generally utilised in the case of significant construction work. Researchers usually prefer accelerated corrosion in concrete to study the features of concrete failure. Hence it is necessary to understand the behaviour of pre-stressing steel under these accelerated conditions of corrosion as the potential in natural corrosion is lower compared to accelerated methods. Present work is a small-scale electrochemical study pertaining to Strands (7 wired 12.7 mm diameter) used in prestressing application. This paper investigates the mode of deposition of rust and tensile behaviour of strands in a corrosive environment subjected to accelerated corrosion conditions. Strand behaviour during failure as a result of corrosion induced weight loss is presented in the following work. Strands were polarized artificially and the behaviour of corroded strands was studied.
Experimental program
Preparation of specimen is associated with significant resources which included controlled exposure conditions, obtaining of standard specimen length. The strands were in accordance with ASTM 416/A416M–18 (Burgatti & Lacerda, Citation2009). The strands used were of 12.7 mm diameter with outer wires of 4.15 mm diameter (6 in number) helically bound around core wire of 4.4 mm diameter. Strands were subdivided in to pieces of 780 mm long. represents characteristics of strand according to Indian Standards 14,268 –1995 (Bureau of Indian Standards, B, Citationn.d.-a).
Table 1. Strand details.
Accelerated corrosion technique
Present study implemented an accelerated corrosion technique which is generally used by researchers to conduct corrosion studies. The basic assumption involved in this technique is that this process is similar to natural corrosion on the basis of that entire current passing in the system produces ferrous ions at anode. Further proceeding to the discussion regarding the influence of chloride concentration and time of exposure, initiation period was premature due to direct exposure of strands into 3% sodium chloride (NaCl) solution (3 grams of NaCl dissolved in 100 ml of distilled water). The sodium chloride undergoes hydrolysis. Formula used for mass of NaCl (grams) is desired percentage of solution multiplied by volume of solution (milliliters). In order to nullify the ambiguity regarding influence of exposure time it was kept constant (96 hours). Accelerated corrosion setup involved external power source (galvanostatic or potentiostatic), prestressing steel as anode with suitable cathode arrangement to induce corrosion was used by Nossoni and Harichandran (Citation2012), similar criteria were adopted in present work. The accelerated corrosion reduced the initiation period of corrosion from years to days meanwhile the corrosion product formed was not altered.
Specimen preparation
The main aim of the study was to induce corrosion in strand and observe the variation of its tensile strength with respect to section loss. The strands were directly exposed to the electrolyte i.e 3% NaCl that aided dissociation of Fe2+ ions. represents the anode, cathode and 3% NaCl. In the present study duration of strand exposure to chloride solution was kept constant (96 hours). Totally 21 strands were utilized for the study with seven variation groups (A, B, C, D, E, F, G) containing three samples each under each variation group. This technique consists of supply of constant current from a DC power source. The current values adopted range from 0.2 mA/cm2 to 2.1 mA/cm2. After inducing corrosion for a determined time, corrosion current Icorr was calculated using Faraday’s Law. Post corrosion samples were cleaned with reference to ASTM G1 03, (Citation2011). Gravimetric weight loss method was used to calculate weight loss. Corroded strand surface was cleaned with hydrochloric acid to remove the corrosion products on the surface. Later the samples were washed with running water and dried in the oven for 15 mins (70°C) (Oparaodu & Okpokwasili, Citation2014) after drying, the samples were weighed and compared to their initial weight. Three range of weight loss percentages 0.1%, 0.5% and 1%were classified as low, moderate and high level of corrosion respectively in order correlate these ranges to varying tensile strength.
Visual inspection and weight loss criteria
Visual inspection classification was compared with characterization of corrosion made by Vecchi et al. (Citation2021). They divided corrosion into 3 levels low, medium and high whose criteria are given in . In the present study, percentage weight loss of 0.1%, 0.5% and 1% were classified as low, moderate and high respectively. Vernier caliper was used to measure the diametrical variation of strand and its wires after corrosion.
Tensile strength test for corroded strands
The tensile strength testing machine consisted of high-capacity hydraulic grips suitable for holding strand specimens firmly without slippage. Following the cleaning of corroded strand specimens and calculation of sectional loss, tensile test was carried out in universal testing machine for group A, D,G and non-corroded specimens to calculate breaking load. The maximum load reached in the tensile test of strand is termed as breaking load (Bureau of Indian Standards, B, Citationn.d.-b) According to A1061/A1061M − 20a (Lequesne et al., Citation2019) specimens were loaded up to failure. Strain gauge connected to the specimen represented deformation which was detached from the specimen prior to the failure to prevent its damage.
Results and discussion
Morphology of corroded strand
Corrosion mechanism involved was “Hydrogen Embrittlement”. As per ACI 222.2 R–01 sufficient amount of chloride can result in hydrogen embrittlement. The setup acted as cathodic protection with strand as anode. Availability of sufficient amount of chloride in contact with the strand surface disturbed the existing passive layer of strand. The dissolution of iron into positively charged ions which attracted the negatively charged chloride ions toward the surface resulting in formation of ferric chloride which on hydrolysis produces insoluble rust and free acid.
Water dissociation at anodic site produced H+ ions which later on travelling to cathodic site got converted to hydrogen atoms which got adsorbed on the strand surface and resulted in hydrogen embrittlement.
Morphology of the accelerated corroded specimens was examined. Even though this technique is advantageous in inducing desired corrosion behaviour, the drawback exists as it fails to completely simulate the natural corrosion as induced corrosion is dependent on applied corrosion current density. Usually, immersion techniques (Griess & Naus, Citation1980) or salt spray on stressed strands (Song et al., Citation2020) are chosen by researchers to induce corrosion but in present work accelerated corrosion was chosen as the former is time consuming (2000 hours − 6500 hours) and latter can be achieved within few days. Former technique results in air – water interface attack which results in variation of diameter above and below the water. Meanwhile rust deposition occurred in interstitial spaces which was partially compatible to that of naturally corroded strands. As the strands were directly in contact with solution macro-cell formation was difficult and without any choice general corrosion took place with hydrogen embrittlement as corrosion mechanism. Though there was free access of air to the corrosion set up no cracks were encountered but broad eroded mass was developed on the strand surface and this was in accordance with study by Griess and Naus (Citation1980). Needle and flock like corrosion product deposition was observed which was comparable to that of study by Song et al. (Citation2020) who observed 0.01 mm wide corrosion pits on galvanized steel strand under non-stressed conditions subjected to salt fog spray. In present work it was the crevice region where the corrosion was initiated. This occurred due to the lack of oxygen in the crevice area which led to mobility of chloride ions in order to maintain charge neutrality; this phenomenon was in agreement with study conducted by Moser et al. (Citation2011). With no time difference gradual rust deposition was initiated on the surface in the form of general corrosion. Since the strands were directly exposed into electrolyte there was simultaneous rust deposition in interstitial space between the wires similar phenomena occurs in pre-stressing steel within concrete also, but this is the criteria where pre-stressing steel differs from conventional steel where the former experiences corrosive product deposition in the spaces between the wires therefore there will be very less or no visible signs observed in concrete and latter with external deposition exerts pressure on surrounding concrete gives prior information with crack development. shows the rust deposition in the crevice and interstitial region.
Uniform surface attack was observed along with formation of localized pits of shorter depth as shown in .
The regions of contact among helically bound wires and the central wire acted as potential corrosion sites which can be described as a crevice corrosion. It is observed from the study that helically wound 6sixwires of strand were the ones that were most affected by accelerated corrosion compared to central wire which seemed least affected. This can be observed in .
Compared to naturally corroded strands, accelerated corroded strands represented drastic reduction in strength and ductility within a short time of exposure which is in agreement with the investigations by Chai et al. (Citation2021) and Andrade et al. (Citation1989). In present work corrosion rate was determined for various corrosion current density. On consideration of naturally corroded strands which are exposed to service the current density keeps varying throughout the service life (Sun & Hong, Citation2002).The loss of materials and its deposition as rust reduced the strength and ductility of strand which is depicted in the results of tensile strength. represents various corrosion rates for seven different groups.
Table 2. Corrosion rates.
represented good correlation between corrosion current density and corrosion rates. The positive slope implies increase in independent variable (corrosion current density) increases the dependent variable (corrosion rates). From present study it is clear that irrespective of the level of corrosions considered, the morphology and mode of occurrence of corrosion did not vary in initial stages. Only the propagation and deposition of rust varied as follows, for Icorr values from 0.2 mA/cm2–0.6 mA/cm2 (slow), 0.6 mA/cm2–1 mA/cm2 (medium), 1 mA/cm2–2.1 mA/cm2 (Fast) shown in . Based on the seven observations made on A-G specimens group as per the study requirement, group A, D and G were chosen as three categories of observation.
showed mean weight loss caused 15.24% drop in tensile strength. Null hypothesis with assumption that there is no effect of weight loss on tensile strength was efficiently rejected (p < 0.05) due to the P -value of 0.033. Thus, cross-section loss is considered as a statistically significant parameter affecting the tensile capacity of strand. Loss in section decreased the tensile load bearing capacity of the strand. Pearson correlation in depicted strong negative correlation exists between weight loss and tensile strength. Based on the conclusion of Giriraju et al., (Citation2022) even single wire failure was considered as strand failure itself.
Table 3. Descriptive statistics.
Table 4. Pearson correlations.
Section loss of the strand
The pits evolving into cracks due to corrosion under stress is a general phenomenon observed in prestressing steel (Turnbull et al., Citation2006). In the case of prestressing steel, structural implications are critical even when section loss is minor as it influences the mechanical behaviour of the strand. As the surface quality of strand is superior to that of deformed bars the type of corrosion observed was surface material erosion and general corrosion respectively which is in accordance with study of Li et al., (Citation2011) the wires incurred corrosion attack in the form of shallow and wide eroded mass. The total area of the un-corroded strand with12.7 mm diameter is 98.7 mm2. The corroded section in represents the shape of corroded regions which were helpful in identifying the section loss. It is mentioned in the work by Sason (Citation1992), that central wire is protected by outer wires during humid exposure conditions assuming the above mentioned condition to be true outer wires (6 in number) were measured in .
Tensile test for corroded strands
It is a well-known fact that the most corroded wire with high sectional loss influences the mechanical property of the strand (Jeon et al., Citation2019). Cross-section loss is the parameter influencing tensile strength of steel and mode of failure. The test specimens were tested in universal testing machine with two ends of the strand held in grip. Strand specimen in test setup and failed specimen are shown in respectively.
Elongation was measured using extensometer which was detached prior to the failure in order to prevent its damage. Tensile strength degradation is attributed to the section loss of wires. represents the tensile capacity of strands for non-corroded, group A, D and G specimens.
Characteristic strength of the strand used was 1860 MPa. Ultimate load decay was observed and this was in accordance with Scott Calabrese Barton et al. (Citation2000). However, group A specimens with 0.1% material loss had minor effect on tensile strength, they suffered 2% drop in tensile strength which is in accordance with previous study by Rengaraju et al. (Citation2020). Trejo et al. (Citation2009) reported difference in capacity loss between stressed and unstressed samples ranged from 1.6% to 18.7% and the exposure period ranged up to 12 months which is a long waiting period. In present work within a few hours desired corrosion ranges were obtained and 0.1% material loss due to corrosion was taken as low-range deterioration and is considered as trigger warning for the structural safety. Group D specimens with 0.5% weight loss behaved ductile too and tensile strength drop was 7%. This is considered as mid-range deterioration; thus 0.5% weight loss is alarming and suitable precautionary measures and retrofitting should be carried out. Group G strands with 1% weight loss suffered brittle failure with 36% drop in tensile strength. This high-range corrosion with 1% weight loss is proved to be detrimental when structural safety is considered as it can lead to sudden failure. This can be attributed to the fact that deposition of corroded material in interstitial spaces and crevice corrosion products made the strands fail in brittle manner with “chevron pattern” observed at the failure cross section which can be observed in the . It is observed that outer wires were the first to fail. For the study purpose the specimen was unmounted after outer wire failure. There exists a strong negative correlation between weight loss percentage and tensile strength as represented in where both the parameters are inversely related to each other. Group G specimens failed in brittle manner as accelerated corrosion of strand in chloride environment induced hydrogen adsorption.
Material loss affected the breaking strength. As a matter of fact, the group D strands with moderate corrosion possessed shallow eroded mass due to which the strands failed to reach the required tensile strength. Meanwhile group G strands failed at lower levels of load in brittle manner .
Conclusion
Accelerated corrosion is an effective method applied by researchers for the study of corrosion in steel. Due to acceleration of corrosion as contrary to natural corrosion, macro-cell formation did not occur and initially the surface was eroded which further proceeded as general corrosion. Relationship between weight loss and tensile capacity of prestressing strands was investigated in the present study and following are the conclusions made. Aggressive attack of chloride ions prior to passivation led to hydrogen embrittlement. Material loss being a statistically significant parameter that affects the tensile capacity of the pre-stressing strands.
Rust deposition also occurred in interstitial spaces which is the factor comparable to that of naturally corroded strands. Occurrence of general corrosion implied proportionality between corrosion rate and corrosion current.
Tensile behaviour of strand was dominated at the location having greatest sectional loss. Thus, drop in tensile strengths were classified as triggering, alarming and detrimental for low- mid and high range of corrosion.
Material loss of 0.1% due to corrosion was taken as low-range deterioration and is considered as trigger warning for the structural safety. 0.5% weight loss is alarming and suitable precautionary measures and retrofitting should be carried out.
Strands behaved ductile at low and moderate levels of corrosion but at high levels of corrosion strands behaved brittle (chevron pattern at failure surface) with 36% drop in tensile strength.
Author contribution
Aishwarya Lakshmi: Literature search/Data analysis/Writing – original draft
Poornachandra Pandit: Conceptualization/Data analysis/Writing – review & editing
Gopinath Nayak: Conceptualization/Writing – review & editing
Yamuna Bhagwat: Visualization/Writing – review
Sooraj Kumar: Visualization/Writing – review
Acknowledgments
The authors would like to express their gratitude towards ‘Manipal Academy of Higher Education’ for supporting the research by providing laboratory facility and materials.
Disclosure statement
No potential conflict of interest was reported by the author(s).
References
- Andrade, C. (2017). Reliability analysis of corrosion onset: Initiation limit state. Journal of Structural Integrity & Maintenance, 2(4), 200–10. https://doi.org/10.1080/24705314.2017.1388693
- Andrade, C., Alonso, C., Gonzalez, J. A., Andrade, C., Alonso, C., & Rodriguez, J. (1989, September 6-8). Remaining service life of corroding structures remaining service life of corroding structures, durability of structures. IABSE Symposium. (Iabse Report Volume 57/1). https://doi.org/10.5169/seals-44238
- Angst, U. M. (2018). Challenges and opportunities in corrosion of steel in concrete. Materials and Structures/Materiaux et Constructions, 51(1). https://doi.org/10.1617/s11527-017-1131-6
- Arunkumar, Y. M., Prashanth, S., Pandit, P., Girish, M. G., & Shetty, A. (2023). Finite element analysis of bond behavior in corroded reinforced concrete beams: State-of-the-art. Journal of Applied Engineering Science, 21(4), 1031–1042. https://doi.org/10.5937/jaes0-42252
- ASTM G1 03. (2011). In American society for testing and materials : Vol. G1 03.
- Belletti, B., Rodríguez, J., Andrade, C., Franceschini, L., Sánchez Montero, J., & Vecchi, F. (2020). Experimental tests on shear capacity of naturally corroded prestressed beams. Structural Concrete, 21(5), 1777–1793. https://doi.org/10.1002/suco.202000205
- Bureau of Indian Standards, B. (n.d.-a). IS 14268-1995: Uncoated stress relieved low relaxation seven-ply strand for prestressed concrete -.
- Bureau of Indian Standards, B. (n.d.-b). IS 6006-1983: Uncoated stress relieved strand for prestressed concrete.
- Burgatti, J. C., & Lacerda, R. A. (2009). Standard specification for low-relaxation, seven-wire steel strand for prestressed concrete 1. American Society for Testing and Materials, 43(1), 237–244. https://doi.org/10.1520/A0416_A0416M-18
- Campione, G., & Zizzo, M. (2022). Influence of strands corrosion on the flexural behavior of prestressed concrete beams. Structures, 45(September), 1366–1375. https://doi.org/10.1016/j.istruc.2022.09.073
- Chai, X., Shang, H., & Zhang, C. (2021). Bond behavior between corroded steel bar and concrete under sustained load. Construction and Building Materials, 310, 125122. https://doi.org/10.1016/j.conbuildmat.2021.125122
- Cui, W., Liu, M., Wang, L., Guan, W., Song, H., & Li, F. (2022). Experimental study on deterioration characteristics of prestressed concrete under the coupling of freeze–thaw and corrosion. Journal of Materials in Civil Engineering, 34(2), 1–13. https://doi.org/10.1061/(asce)mt.1943-5533.0004084
- Dai, L., Bian, H., Wang, L., Potier-Ferry, M., & Zhang, J. (2020). Prestress loss diagnostics in pretensioned concrete structures with corrosive cracking. Journal of Structural Engineering, 146(3). https://doi.org/10.1061/(asce)st.1943-541x.0002554
- Franceschini, L., Belletti, B., Tondolo, F., & Sanchez Montero, J. (2022). A simplified stress–strain relationship for the mechanical behavior of corroded prestressing strands: The SCPS-model. Structural Concrete. https://doi.org/10.1002/suco.202200170
- Giriraju, R., Sengupta, A. K., & Pillai, R. G. (2022). Tensile behaviour of corroded strands in prestressed concrete systems. Journal of the Institution of Engineers (India): Series A, 103(3), 867–879. https://doi.org/10.1007/s40030-022-00656-y
- Griess, J. C., & Naus, D. (1980). Corrosion of steel tendons used in prestressed concrete pressure vessels. American Society for Testing and Materials. www.astm.org
- Hansson, C. M. (2016). An introduction to corrosion of engineering materials. In Corrosion of steel in concrete structures (pp. 3–18). Woodhead Publishing. https://doi.org/10.1016/B978-1-78242-381-2.00001-8
- Jeon, C. H., Lee, J. B., Lon, S., & Shim, C. S. (2019). Equivalent material model of corroded prestressing steel strand. Journal of Materials Research and Technology, 8(2), 2450–2460. https://doi.org/10.1016/j.jmrt.2019.02.010
- Lequesne, Rémy, D., Jokymaityte, M., Mirnajafizadeh, A., Carty, C. P., Lloyd, D., & Stewart, R. A. (2019). Development of 18 quality control gates for additive manufacturing of error free patient-specific implants. American Society for Testing and Materials, 12(19), 1061. https://doi.org/10.3390/ma12193110
- Li, F., Yuan, Y., & Li, C. Q. (2011). Corrosion propagation of prestressing steel strands in concrete subject to chloride attack. Construction and Building Materials, 25(10), 3878–3885. https://doi.org/10.1016/j.conbuildmat.2011.04.011
- Li, J., Miki, T., Yang, Q., & Mao, M. (2022). Experimental study on prestressing force of corroded prestressed concrete steel strands. Journal of Advanced Concrete Technology, 20(9), 550–563. https://doi.org/10.3151/jact.20.550
- Li, J., Miki, T., Yang, Q., & Mao, M. (2023). Influence of corrosion conditions on prestressing force and residual tensile capacity of corroded prestressed concrete steel strands. Journal of Advanced Concrete Technology, 21(11), 956–970. https://doi.org/10.3151/jact.21.956
- Menoufy, A. E., & Soudki, K. (2014). Flexural behavior of corroded pretensioned girders repaired with CFRP sheets. PCI Journal, 59(2), 129–143. https://doi.org/10.15554/pcij.03012014.129.143
- Moser, R. D., Singh, P. M., Kahn, L. F., & Kurtis, K. E. (2011). Chloride-induced corrosion of prestressing steels considering crevice effects and surface imperfections. Corrosion, 67(6), 1–14. https://doi.org/10.5006/1.3595096
- Nossoni, G., & Harichandran, R. (2012). Current efficiency in accelerated corrosion testing of concrete. Corrosion, 68(9), 801–809. https://doi.org/10.5006/0428
- Nürnberger, U. (2002). Corrosion induced failure mechanisms of prestressing steel. Materials & Corrosion, 53(8), 591–601. https://doi.org/10.1002/1521-4176(200208)53:8<591:AID-MACO591>3.0.CO;2-X
- Oparaodu, K. O., & Okpokwasili, G. C. (2014). Comparison of percentage weight loss and corrosion rate trends in different metal coupons from two soil environments. International Journal of Environmental Bioremediation & Biodegradation, 2(5), 243–249. https://doi.org/10.12691/ijebb-2-5-5
- Rajbanul Akhond, M., Irfan, A., & Sharif, A. (2023). An overview of corrosion behavior and contemporary management techniques of thermomechanically treated rebars in concrete structures. Journal of Structural Integrity & Maintenance, 1–20. https://doi.org/10.1080/24705314.2023.2259721
- Rengaraju, S., Godara, A., Alapati, P., & Pillai, R. G. (2020). Macrocell corrosion mechanisms of prestressing strands in various concretes. Magazine of Concrete Research, 72(4), 194–206. https://doi.org/10.1680/jmacr.18.00284
- Sadhasivam, K., Bella Canet, E., Wendling, A., & Floyd, R. W. (2017). Effect of vertical strand location on bond performance of prestressing strands cast in lightweight self-consolidating concrete. Journal of Structural Integrity & Maintenance, 2(1), 39–47. https://doi.org/10.1080/24705314.2017.1280588
- Saraswathy, V., Lee, H. S., Karthick, S., & Kwon, S. J. (2018). Stress corrosion behavior of ungrouted pretensioned concrete beams. Advances in Materials Science and Engineering, 2018, 1–11. https://doi.org/10.1155/2018/8585162
- Sason, A. S. (1992). Evaluation of degree of rusting on prestressed concrete strand. PCI Journal, 37(3), 25–30. https://doi.org/10.15554/pcij.05011992.25.30
- Scott Calabrese Barton, B., Vermaas, G. W., Member, S., Duby, P. F., West, A. C., & Betti, R. (2000). Accelerated corrosion and embrittlement of high-strength bridge wire. Journal of Materials in Civil Engineering, 12(2000), 33–38. https://doi.org/10.1061/(ASCE)0899-1561(2000)12:1(33)
- Song, J., Chen, F., & Guo, G. (2020). Corrosion and microstructure behaviour of steel strand used in bridges. Integrated Ferroelectrics, 207(1), 27–36. https://doi.org/10.1080/10584587.2020.1728662
- Su, X., Ma, Y., Wang, L., Guo, Z., & Zhang, J. (2022). Fatigue life prediction for prestressed concrete beams under corrosion deterioration process. Structures, 43(March), 1704–1715. https://doi.org/10.1016/j.istruc.2022.07.043
- Sun, J., & Hong, H. P. (2002). Effect of reinforcement corrosion on reliability of bridge girders. Civil Engineering and Environmental Systems, 19(1), 67–85. https://doi.org/10.1080/10286600212160
- Tran, D. V. P., Sancharoen, P., Klomjit, P., Tangtermsirikul, S., & Nguyen, T. H. Y. (2023). Prediction equations for corrosion rate of reinforcing steel in cement-fly ash concrete. Journal of Structural Integrity & Maintenance, 8(2), 91–99. https://doi.org/10.1080/24705314.2023.2165749
- Trejo, D., Pillai, R. G., Hueste, M. B. D., Reinschmidt, K. F., & Gardoni, P. (2009). Parameters influencing corrosion and tension capacity of post-tensioning strands. ACI Materials Journal, 106(2), 144–153. https://doi.org/10.14359/56461
- Turnbull, A., McCartney, L. N., & Zhou, S. (2006). Modelling of the evolution of stress corrosion cracks from corrosion pits. Scripta materialia, 54(4 SPEC. ISS.), 575–578. https://doi.org/10.1016/j.scriptamat.2005.10.053
- Vecchi, F., Franceschini, L., Tondolo, F., Belletti, B., Sánchez Montero, J., & Minetola, P. (2021). Corrosion morphology of prestressing steel strands in naturally corroded PC beams. Construction and Building Materials, 296, 123720. https://doi.org/10.1016/j.conbuildmat.2021.123720
- Vélez, W., Matta, F., & Ziehl, P. (2016). Electrochemical characterization of early corrosion in prestressed concrete exposed to salt water. Materials and Structures/Materiaux et Constructions, 49(1–2), 507–520. https://doi.org/10.1617/s11527-014-0514-1
- Vu, N. A., Castel, A., & François, R. (2009). Effect of stress corrosion cracking on stress-strain response of steel wires used in prestressed concrete beams. Corrosion Science, 51(6), 1453–1459. https://doi.org/10.1016/j.corsci.2009.03.033
- Wang, L., Li, T., Dai, L., Chen, W., & Huang, K. (2020). Corrosion morphology and mechanical behavior of corroded prestressing strands. Journal of Advanced Concrete Technology, 18(10), 545–557. https://doi.org/10.3151/jact.18.545