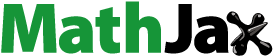
ABSTRACT
New possibilities for sustainable and climate-friendly plastic production are urgently needed. One degradable alternative could be polyhydroxybutyrate naturally produced out of CO2 via photosynthesis within cyanobacteria. Therefore, the polyhydroxybutyrate production of the so far little investigated polyhydroxybutyrate overproducing Synecho-cystis sp. PCC6714 mutant Mt_a24 should be further increased. The impact of reducing the exopolysaccharide production on the polyhydroxybutyrate yield was examined by knocking the exoD gene out. The generated transformants KOexoD#4 and KOexoD#65 were compared to the control strain under standard and nutrient limiting cultivation conditions regarding their growth, glycogen, polyhydroxybutyrate and exopolysaccharide production. Whereas the growth of all three strains was comparable under standard conditions, only minor differences could be detected under limited conditions. An increase of polyhydroxybutyrate could be observed in both transformants (KOexoD#4: 1.17-fold, KOexoD#65: 1.26-fold) under limited conditions. The exopolysaccharide production was increased in both transformants under standard cultivation conditions, showing a strong increase of soluble exopolysaccharides. In contrast, during limitation, the soluble exopolysaccharides decreased in all cultures whereas the tightly bound exopolysaccharides fraction strongly increased. Hence, the influence of limiting medium, and connection to the energy storage pathways could be shown as well as the great potential of Mt_a24 as a sustainable production system for polyhydroxybutyrate.
Introduction
In 2018, the European Commission has developed a strategy for plastics in a circular economy to reduce the environmental damage caused by plastic products and their production. This should lead to more efficient recycling strategies and efficient production of plastics. This production and the incineration of waste causes approximately 400 million tons of carbon dioxide (CO2) annually [Citation1]. As this greenhouse gas contributes significantly to global warming, emissions should be reduced accordingly [Citation2]. The avoidance or economic use of greenhouse gases is therefore of great importance. Bioplastics are a means of addressing global warming [Citation3]. One possible method of using CO2 could be the conversion into valuable products by photosynthesis, which is carried out by plants, algae, and cyanobacteria [Citation4–9].
Cyanobacteria are a promising option as a microbial cell factory due to their ability to convert up to 3–9% of the solar energy into biomass compared to ≤0.25–3% achieved by crop plants, for example, corn and sugar cane. Furthermore, the production via algae or cyanobacteria does not compete with agricultural land, as no land, which is needed for feed or food supply, is used. Thus, these advantages of this production system could be combined with the production of valuable industrial products [Citation10–13]. One of these valuable products is polyhydroxybutyrate (PHB), which is a natural storage compound of some cyanobacteria. Under nutrient (like phosphorus or nitrogen) limiting conditions, its natural production can be increased. Currently, PHB is obtained by processing different plant materials and can be used as a plastic alternative to polypropylene (PP) with its benefits of being biodegradable and produced with cyanobacteria also sustainably [Citation14–16]. The natural PHB content in wild type cyanobacteria varies between 3% (Synechococcus elongatus PCC 7942) and 85% per dry cell weight (DCW) (Alusira fertilisima CCC444) depending on the species, carbon source, and growth conditions [Citation12]. For commercial use, higher yields in a shorter production time are desired.
For further enhancement of the PHB production yields, one possibility is the improvement of the strains next to the optimized cultivation and recovery process [Citation12]. For genetic improvement, strains like S. elongatus PCC7002 and PCC7942 as well as Synechocystis sp. PCC6803 are chosen, whose genomes are already sequenced. This information is mandatory for the use of molecular techniques like the overexpression of genes relevant for PHB production, which lead up to 2.5-fold increased PHB (26% PHB per DCW) in Synechocystis sp. PCC6803 [Citation17]. Other approaches deal with the increase of the precursor availability like acetyl CoA, from which PHB is produced, within three steps with acetoacetyl-CoA and 3-hydroxybutyryl-CoA as step products. An increase of the PHB yield to 232 mg L−1 (12% per DCW) could be observed [Citation10]. The knockout of competitive storage pathways like glycogen resulted in enhanced storage of PHB up to 19% per DCW compared to 3% in the wild type Synechocystis sp. PCC6803 [Citation18]. Further approaches are dealing with UV mutagenesis resulting in the strain Mt_a24 with an up to 2.5-fold increase of PHB (38% per DCW) compared to the wild type Synechocystis sp. PCC6714. Synechocystis sp. PCC6803 was mainly used as model strain in the last decades, is well characterized and lots of techniques for transformation and genetic engineering are well known. In contrast, the genome sequence of the strain Synechocystis sp. PCC6714 shows a similarity of over 75% to Synechocystis sp. PCC6803 and differences in their tolerance toward metal ions and salt concentrations were detected [Citation19]. Even though, the strain Synechocystis sp. PCC6714 was not further characterized so far. The Synechocystis sp. PCC6714 UV mutant (Mt_a24), described by [Citation20], shows a high potential for PHB production, which will be further investigated in the scope of this work.
The availability of general energy supply should be further increased by the reduction of the exopolysaccharide (EPS) production. EPS is an extracellular matrix consisting of different monosaccharides. It protects the cells from environmental stress (irradiation, osmotic stress) and binds nutrients to improve the growth [Citation21–23]. Three different types of EPS are built: the capsular EPS, which is attached to the cells and can be divided into the loosely (LB-EPS) and tightly (TB-EPS) bound fractions. Another type is the soluble EPS (S-EPS), which is released to the surrounding environment [Citation24,Citation25]. The composition of bacterial EPS varies with the type of microorganisms, nutrient availability, growth phase, and environmental conditions [Citation26–33].
The synthesis of EPS in the Synechocystis strains is still not fully understood, yet. It is assumed that it follows the pathways described for other bacteria in which the Wzy, ABC transporter or synthases are involved [Citation30]. Whereas some proteins, e.g., for the polymerization and export, are conserved among bacteria, strain-dependent enzymes, e.g. for sugar activation/modification, leading to different EPS compositions [Citation34]. Due to the ability of cyanobacteria to perform oxygenic photosynthesis and their physical organization differs from other bacteria, other types of regulation were suggested. Supporting the fact, that up to 12 different sugars like glucose, galactose, mannose, fructose, ribose, xylose, arabinose, different types of deoxyhexosen and methyl and/or amino sugars were found in cyanobacterial EPS, whereas less than 4 different monomers were described in other bacteria [Citation29,Citation35–37]. Further information could be gained by the knockout of the two genes expressing the exopolysaccharide synthesis protein exoD (slr1875) and the putative polysaccharide export protein gumB (sll1581), which are involved in several EPS synthesis pathways. This double knockout resulted in a reduction of up to 50% in the strain Synechocystis sp. PCC6803 [Citation24]. The knockout of the single or both genes did not have an effect on the growth or biomass production. Therefore, EPS seems to be not necessary for cultivation under laboratory conditions. Thus, we assume that by reducing the EPS production, cyanobacteria could have more precursors available to produce other metabolites, e.g. PHB.
The role of the exoD gene within the EPS synthesis as well as its impact on the EPS production and its correlation with the production of the main storage compounds PHB and glycogen are still not clear in the strain Synechoycstis sp. PCC6714 as well as in its mutant Mt_a24. Hence, the impact of the knockout of the exoD gene within the PHB overaccumulating mutant Mt_a24 should be evaluated. Therefore, exoD knockout transformants were generated out of this mutant. The growth and the production of the main storage compounds glycogen and PHB under different cultivation conditions as well as their EPS production will be compared with the mutant Mt_a24. Additionally, effects on the phenotype will be pointed out by scanning electron microscope measurements.
Materials and methods
Strain and inoculum preparation
In this study, the mutant strain Mt_a24, producing increased PHB yield due to UV mutagenesis of the wild type strain Synechocystis sp. PCC 6714 [Citation20], was used. For genetic engineering, the strain was cultivated in BG11 medium [Citation38] supplemented with 10 mmol L−1 NaHCO3 at 30°C, 10–50 μmol photons m−2 s−1 and 60 rpm. For the cultivation on plates, 1.5% agar was added to the BG11 medium. 2.5 µg mL−1 chloramphenicol (Cm) was added to the medium if engineered strains were cultivated.
The screening experiment for PHB production was carried out with 50 mL cultures in 250 mL Erlenmeyer shake flasks. The starting optical density (OD) at 750 nm was 0.1 and the conditions in the illuminated Minitron incubation shaker with CO2 atmosphere (Infors, CH) were 28°C, 120 rpm, continuous illumination at 50 μmol m−2 s−1 and 5% CO2. The starting medium was BG11. After 7 days the culture was centrifuged at 25°C and 2,500 g for 5 min and resuspended in 50 mL BG11 medium without nitrogen and phosphorus in order to start the limiting phase for additional 7 days. 2.5 mL samples were taken at the end of growth phase before media exchange on day 7, after 4 days (day 11), and after 7 days of limiting phase (day 14). The screening experiment was carried out in triplicates. OD measurements for estimation of DCW (see EquationEquation (1))(1)
(1) were carried out on a Nanodrop One photometer (Thermo Fischer Scientific, USA). The culture sample was split into 1 mL reaction tubes and centrifuged at 4°C, 20,000 g for 10 min to get cell pellets for subsequent PHB and glycogen determinations.
For the determination of the EPS production as well as the scanning electron microscope (SEM) measurements, 100 mL cultures with a starting OD at 730 nm of 0.5 were cultivated in 250 mL Erlenmeyer shake flasks with 50 mmol L−1 NaHCO3 as CO2 source, at 30°C, 60 rpm and continuous illumination at 50 μmol m−2 s−1. 10 mmol L−1 NaHCO3 was added each day. On day 7 the culture was split and centrifuged at 3,000 rpm for 20 min and one half resuspended in 100 mL standard BG11 medium and the second half in 100 mL BG11 medium without nitrogen and phosphorus and cultivated for another 7 days (D14). The experiment was carried out in triplicates.
The E. coli strain XL1 blue for plasmid construction was obtained from the Institute of Biophysics (Linz, Austria). It was grown on LB agar (1.5%) supplemented with 100 µg mL−1 ampicillin or 50 µg mL−1 Cm at 37°C for the plasmid construction and in LB medium at 37°C and 170 rpm for plasmid isolation.
Construction of knockout plasmids
In order to inhibit EPS synthesis for increased PHB production, the gene D082_28320, of the strain Synechocystis sp. PCC6714 encoding for the exopolysaccharide synthesis protein (exoD) was selected to be knocked out of the genome. The genomic DNA of Mt_a24 was extracted according to Citation39, up- and downstream flanking regions (500 bp) of the gene were amplified out of the genome to be used as homologous integration sites by the DNA polymerase KAPA HiFi (Kapa Biosystems). The antibiotic resistance cassette for Cm (CmR) replacing exoD was amplified out of the plasmid pEERM4 (addgene). The amplified fragments were cloned into the plasmid pBlueskript II SK (+) using the Gibson Assembly® Cloning Kit (New England Biolabs, NEB). The used primers are summarized in . The plasmids were transformed into chemically competent E. coli XL1 blue by heat shock [Citation40] and were isolated using the Plasmid Miniprep Kit GeneJET (Thermo Scientific). The plasmids were verified with colony PCR using either the Firepol® DNA polymerase (Solis BioDyne) or the OneTaq® DNA polymerase (NEB) and were sequenced at Eurofins Genomics (Ebersberg, Germany).
Table 1. List of primers used for the plasmid construction and genome integration screening of the transformants. Italic – sequence of overhang for Gibson Assembly®; pBS – pBlueskript II SK (+)
Construction of transformants
Before the transformation, the strain Mt_a24 was picked from the plate and cultivated in BG11 medium for 7 days to ensure the viability of the culture. The sample preparation for the electroporation was done according to [Citation41]. The complete supernatant was removed after the last washing step and the pellet was re-suspended in 100 µL 1 mmol L−1 4-(2-hydroxyethyl)-1-piperazineethanesulfonic acid (HEPES) buffer (pH 7.5). 70 µL cell suspension was mixed with 1–5 µg linear or plasmid DNA (diluted in ultrapure water) and pipetted into a cuvette (gap 0.1 mm, Laktan). The electroporation was carried out with the Amaxa General Nucleofection (Lonza) using the bacterial program 3. Immediately after the electroporation, the cells were re-suspended in 1 mL BG11 and transferred into 250 mL shaking flasks filled with 50 mL BG11 medium containing 10 mmol L−1 NaHCO3. The cells were incubated for 5 days at 30°C, 50 μmol photons m−2 s−1 and 60 rpm. The culture was harvested at 3,000 rpm for 20 min and re-suspended in 300 µL BG11. 50 µL and 150 µL of the culture were, respectively, plated on BG11 agar supplemented with 2.5 µg mL−1 Cm and 10 mmol L−1 NaHCO3. The culture was incubated at 30°C and 10 μmol photons m−2 s−1 for 2–4 weeks until single colonies occurred. Those single colonies were transferred onto new plates and tested via Multiplex-PCR using the OneTaq® Quick Load® DNA polymerase (NEB) and the primers 6–34 F, 10–15 F, and 10–16 R for the genomic integration of the knockout construct. The visualization was done on a 1% agarose gel. The primer sequences are summarized in .
Determination of polyhydroxybutyrate content
The quantification of the PHB content was done as previously described by [Citation42]. The cell pellet of 1 mL culture was dried at 80°C for 24 h. 1 mL of conc. H2SO4 was added to the pellet and the sample was placed on a heating block at 95°C and 900 rpm for 60 min. Subsequently, the sample was centrifuged at 4°C and 20,000 g for 10 min. 100 μL of the supernatant was added to 1,900 μL H2O, mixed, and filtered through a 0.22 μm PTFE filter. PHB, from natural origin (Sigma Aldrich, USA) was treated accordingly for the preparation of calibration standards. The measurements were done on an UltiMate 3000 high-performance liquid chromatography (HPLC) system (Thermo Fischer Scientific, USA). The stationary phase was an Aminex HPX-87 H column (BioRad, USA) temperature controlled at 60°C. The isocratic mobile phase was 4 mmol L−1 H2SO4 and the flow rate was set to 0.6 mL min−1. The detection was done using a diode array detector (DAD) at 210 nm. Instrument control and evaluation of the spectra were done by Chromeleon 7.2 (Thermo Fischer Scientific, USA). The PHB content per DCW was calculated with EquationEquation (2)(2)
(2) .
Determination of glycogen content
Glycogen quantification was done similarly as described by [Citation43]. The cell pellet of 1 mL sample was dried at 80°C for 24 h. The pellet was resuspended in 1 mL of 7.5% (v/v) H2SO4 and placed on a heating block at 95°C and 900 rpm for 120 min. Then, the sample was centrifuged at 4°C and 20,000 g for 10 min. 100 μL supernatant was added to 900 μL H2O in an IC vial made of PE (Thermo Fischer Scientific, USA). Glycogen (Sigma Aldrich, USA) was treated accordingly for the preparation of calibration standards. Analysis were executed on an ICS-6000 ion chromatography (IC) system (Thermo Fischer Scientific, USA). A Dionex CarboPac PA10 column (Thermo Fischer Scientific, USA) was run at 30°C with NaOH eluent. The isocratic elution of analyte by 20 mmol L−1 NaOH for 15 min was followed by a 200 mmol L−1 NaOH washing step for 10 min and 20 mmol L−1 NaOH equilibration step for 35 min before the next sample was injected. An electrochemical (EC) detector was used for spectrum generation. Chromeleon 7.2 (Thermo Fischer Scientific, USA) was the software for method control and peak evaluation. The glycogen content per DCW is calculated with EquationEquation (3)(3)
(3) .
Determination of exopolysaccharide content
The sample preparation to determine the EPS content was done according to [Citation25]. 1 mL sample was taken and centrifuged at 2,500 rpm for 15 min. The supernatant was collected to determine the soluble EPS (S-EPS). The remaining cell pellet was re-suspended in 1 mL 0.05% NaCl and centrifuged at 5,000 rpm for 15 min. The supernatant was collected to determine the loosely bound EPS (LB-EPS). The remaining cell pellet was re-suspended in 1 mL 0.05% NaCl, incubated for 30 min at 60°C, centrifuged at 13,000 rpm for 15 min and the supernatant was collected to determine the tightly bound EPS (TB-EPS). The analyses of the EPS content was done according to [Citation44]. 2.5 mL 96% H2SO4 was added to each harvested supernatant (S-EPS, LB-EPS, TB-EPS) to hydrolyze the EPS into monosaccharides, which form 5-(hydroxymethyl)-2-furfural aldehyde complexes due to the resulting heat. Then, 0.4 mL 5% phenol was added resulting in yellow-brown coloring due to the reaction of phenol with the complexes during the 20 min incubation at room temperature. 2 mL of the sample was transferred into a quartz glass cuvette (SUPRASIL® 100-QS, Hellma, light path: 10 mm) and analyzed with a spectrophotometer (XION 500, Hach Lange GmbH, LPG385) at 494 nm. Glucose (Sigma Aldrich, USA) was treated and analyzed accordingly as an external calibration standard.
Scanning electron microscope measurements
In order to visualize cells with the scanning electron microscope (SEM), they were first fixed in their growth state according to [Citation24]. 1 mL sample was taken, the OD at 730 nm measured and the OD adjusted to 0.7 in 1 mL. 25 µL glutaraldehyde was added to fix the cells at 4°C overnight. Afterward, the cells were centrifuged at 2,400 rpm for 10 min and the supernatant discarded. The pellet was washed three times by adding 1 mL ultra-pure water, centrifuged at 2,400 rpm for 10 min and the supernatant discarded. 100 µL ultra-pure water was added to the sample. 1 µL sample was mounted on an aluminum pin stub (diameter top), dried at room temperature and Au-sputter coated using the cressington sputter coater 108auto. The images were observed with a TESCAN MIRA3 FEG-SEM microscope, operated at 10.0 kV, at a SEM magnification of 30.0 kx and a working distance of 10.17 mm – 10.22 mm.
Results and discussion
The gene exoD was described to be involved in EPS production within the cyanobacteria Synechocystis sp. PCC6803 [Citation24], whereas its role in the synthesis is still unclear. As this gene has not been studied beforehand within the strain Synechocystis sp. PCC6714, its impact on the EPS production as well as its correlation with the production of the main storage compounds PHB and glycogen should be investigated within this work. Moreover, the hypothesis that a decrease in EPS production could lead to an increase of available precursors to produce e.g. storage compounds like PHB will be explored.
Production and screening of transformants
In order to reduce the EPS production, the gene exoD, known to be part of its synthesis process, was knocked out. Therefore, a knockout plasmid carrying the flanking regions of the gene exoD referred to as upstream (US) and downstream (DS) and a chloramphenicol resistance cassette replacing exoD, was designed and cloned (shown in )). Using electroporation, this knockout plasmid was taken up by the cells and the resistance cassette was integrated via double homologous recombination into the genome. The transformed cells were cultivated for 5 days without antibiotic selection pressure to recover from the electroporation. Then, they were transferred on agar supplemented with antibiotics until single colonies occurred. 233 clones of those single colonies were transferred on new plates, of which 13 grew after the transfer. Seven transformants, which constantly grow throughout the passages, were screened via Multiplex-PCR. The verification of the complete exoD-deletion in all genomes was done with the transformant genome-specific primers 6–48 F and 10–16 R as well as the wild type genome-specific primers 10–15 F and 10–16 R. Their binding sites are shown in ). The tested transformants are shown in ). In four out of the seven screened transformants only the transformant-specific fragment of 0.966 kb could be detected, verifying the complete deletion of the gene exoD out of each genome copy. Transformant #2 showed the transformant genome-specific (0.966 kb) as well as the wild type genome-specific fragment (0.676 kb), indicating some genome copies still have the gene exoD integrated. As the signal intensity of transformant KOexoD#4 and KOexoD#65 is the strongest, those were used for further analysis regarding growth behavior, storage compound, and EPS production.
Figure 1. Genetic modification of a PHB overaccumulating mutant Mt_a24. a) Schematic demonstration of genome section of the wild type with the gene exoD and the generated transformant with exchange of exoD through a chloramphenicol resistance gene and the binding sites of the primers for the verification of genomic integration. b) Multiplex-polymerase chain reaction with the OneTaq® Quick Load® DNA polymerase (NEB) and the primers 6–34 F, 10–15 F and 10–16 R to verify the knockout (KO) of exoD in the transformants generated via electroporation of the Mt_a24 mutant

Determination of growth behavior and storage compound production
The influence of the genetic modification on the cell’s physiology was investigated by analyzing their growth behavior and production of storage compounds compared to the control strain Mt_a24. The cell growth was determined as DCW in g L−1 by calculating the value using the OD at 750 nm and EquationEquation (1)(1)
(1) . The cell growth is shown in ). The cultures were cultivated first under normal conditions for 7 days followed by a 7-day cultivation under nitrogen and phosphorus limitation, which should increase the natural storage of glycogen and PHB [Citation45]. The control strain and the transformants grew similar until the media exchange on day 7 when KOexoD#65 showed a slightly increased growth of 1.11-fold. After 4- and 7-days of nitrogen and phosphate limitation, KOexoD#4 showed no negative effects on its growth behavior due to the gene knockout as it gained a similar DCW of 2.06 g L−1 as the control strain with 2.11 g L−1. While KOexoD#65 grew minor (1.86 g L−1) during the limitation step compared to the other two.
Figure 2. Characterization of the unmodified mutant strain Mt_a24 and its two transformants KOexoD#4 and KOexoD#65 carrying a knockout of the gene exoD by replacement with a chloramphenicol resistance gene, during a cultivation of 7 days in standard BG11 medium followed by a 7 days cultivation within medium deficient of nitrogen and phosphorus with an intermediate sampling on day 4 of limitation. a) determination of the dry cell weight (DCW) by measurement of the optical density at 750 nm; b) analysis of the polyhydroxybutyrate (PHB) yield after acid hydrolyzation and HPLC measurement; c) analysis of the glycogen yield after acid hydrolyzation and IC measurement

The yield of PHB was determined by HPLC which values are correlated to the DCW and presented in % as g L−1 PHB per g L−1 DCW. As shown in ), differences at the produced PHB value could be observed after 7 days of cultivation in a limited medium. An increase of PHB in both transformants compared to the control could be observed, with an absolute increase of 2% (1.17-fold) for KOexoD#4 and 3% (1.26-fold) for KOexoD#65. Thus, suggesting that the knockout of the gene exoD correlates with the PHB production under limiting conditions. Moreover, so far the highest reached space time yield (STY) of PHB by [Citation20], for the Mt_a24 was 134.2 mg L−1 d−1 with an average production of 36.9 mg L−1 d−1 compared to the wild type Synechocystis sp. PCC6714 with the highest STY of 101.6 mg L−1 d−1 and an average production of 11 mg L−1 d−1. A maximum of 37% PHB per DCW was gained in the mutant. The same medium, limitation, and cultivation conditions were used within a 250 mL DASbox Mini Bioreactor System. The reproduction of the results within this paper by four separate cultivations of Mt_a24 showed a varied PHB amount between 15% and 20% after 7 days of limitation (process time 283 h). Thus, in the scope of this work, the PHB production of the control strain was at 13% after 7 days limitation, this yield is in agreement with the study of [Citation20].
The glycogen production (shown in )), measured by ion chromatography, showed increased glycogen production of the transformants during the cultivation with standard medium after 7 days. The presented values were calculated in % as g L−1 glycogen per g L−1 DCW. Both generated transformants produced 4.8-fold more glycogen (KOexoD#4: 1.50% and KOexoD#65: 1.52%) than the control Mt_a24 with 0.31%. Nitrogen and phosphorus limitation increased the glycogen production in all tested strains, whereas the control showed the highest increase of 134-fold after 7 days of limitation (41.83%). These results are comparable to the results of the Mt_a24 in [Citation20], showing values between 20% and 40% glycogen after 283 h. The glycogen production of the transformants within limited medium was slightly reduced with 0.96-fold for KOexoD#4 and 0.84-fold for KOexoD#65 compared to the control. As both transformants showed less glycogen and higher PHB production, this suggests that the exoD knockout may have an influence on both storage compounds. Moreover, the hypothesis of increased PHB due to the exoD gene knockout could be shown in both transformants. A further approach for an increased PHB yield could be the knockout of the glycogen synthesis, in order to increase the amount of precursors for the PHB production.
Determination of EPS production
The production of EPS within the cyanobacteria control Mt_a24 as well as its generated transformants KOexoD#4 and KOexoD#65 were analyzed at different cultivation days and under different cultivation conditions by extracting the three different EPS fractions from the cells. The soluble EPS (S-EPS) fraction was analyzed directly from the culture medium, the loosely bound EPS (LB-EPS) were removed by centrifugation from the cells and the tightly bound EPS (TB-EPS) were gained through heat incubation of the cells. The quantification was done by hydrolyzation of the EPS into monomers, successive reaction with phenol and measured via a spectrophotometer. In the EPS production of the different strains in standard BG11 medium as well as in BG11 medium without nitrogen and phosphorus on different cultivation days is shown. The transformants show an increase of the EPS production within standard medium throughout the cultivation, whereas the EPS production of the control was first doubled from day 7 to day 11 and then reduced to the starting value on day 14. Moreover, the highest total EPS production was observed within KOexoD#4 (110.23 mg L−1 EPS g L−1 DCW) after 7 days in limited medium (D14). This strain produced most total EPS on each analyzed cultivation day. The distribution of the three EPS fractions was changed in all strains through the change of the cultivation conditions. In the standard medium, most S-EPS were produced within all strains, whereas the transformants produced 1.96-fold (KOexoD#4) and 1.31-fold (KOexoD#65) more compared to the control on day 7 and 2.76-fold and 2.69-fold more on day 14, respectively. The production of the LB-EPS throughout the cultivation did not change much, whereas the TB-EPS increased 3-fold within the control and only 1.76-fold (KOexoD#4) and 1.66-fold (KOexoD#65) in the transformants from day 7 to day 11. These results suggest that the knockout of exoD did have an influence on the EPS production and the distribution of the EPS fractions. Under standard conditions, more EPS is produced and especially the S-EPS are increased due to the knockout, suggesting, that exoD may influence the bondage to the cells [Citation24], showed the same behavior for the strain Synechocystis sp. PCC6803, when its exoD gene was knocked out. Within their study, the combined knockout of exoD with the gene gumB, which expresses the putative polysaccharide export protein, resulted in a reduction of the total EPS production. As the behavior of the exoD knockout was comparable to the strain Synechocystis sp. PCC6803, a double transformant containing both knockouts may result in decreased EPS production in the strain Synechocystis sp. PCC6714 as well. Hence, more precursors may be available for producing storage substances like PHB.
Figure 3. Analysis of the three different EPS fractions, which are either excreted into the medium (S-EPS), loosely bound (LB-EPS) or tightly bound (TB-EPS) to the cell, of the unmodified control strain Mt_a24 and its two transformants KOexoD#4 and KOexoD#65 in standard and nitrogen and phosphorus limited medium (-NP). The analysis was done by hydrolyzation of EPS into monomers, reacted with phenol and measured via a spectrophotometer

In contrast, in this presented study under limiting medium conditions in all strains, the production of TB-EPS was enhanced and the S-EPS was reduced. On day 11, the control produced half S-EPS and double TB-EPS under limiting conditions compared to the standard cultivation on the same day. The transformants showed the same behavior with an increase and decrease of 3-fold for KOexoD#4 and 2.7-fold increase and 1.9-fold decrease for KOexoD#65 on day 11. This suggests, that under nutrient deprivation the organism stores the EPS around the cells for a faster supply compared to standard conditions where they are mainly released into the medium. As the transformants behave similar to the control under nutrient limiting conditions, the gene exoD may not be involved in the EPS production during nutrient limitation, as deletion did not affect the EPS production very much in limited medium.
As it is published that nitrogen limitation forces the cell to increase storage compounds [Citation45], this could be the reason for fewer S-EPS within limiting medium and that most secreted sugars are located around the cells. Those differences in the composition of EPS caused by cultivation under different nutrient availability, growth phase, and environmental conditions were already shown in other bacteria [Citation26–29,Citation31–33].
Scanning electron microscope measurements
The impact of the gene knockout on the phenotype of the cells was investigated by fixation of the cells in glutaraldehyde and analysis with a scanning electron microscope (SEM). In order to remove glutaraldehyde residues, the cells were washed three times with water, which may cause the removal of the EPS fractions, too. As a result, only the impact of the knockout on the phenotype and not on EPS production is discussed. shows the cells of the control culture Mt_a24 and its two generated transformants with a knockout of the gene exoD (KOexoD#4 and KOexoD#65) on different cultivation days (days 7, 11, and 14) and conditions (standard or nitrogen and phosphorus limiting medium). The analyzed cells in were cultivated in standard medium without any limitations. The shape of the transformants does not differ from those of the control and cell division can be seen in all three strains on both sampling days (day 4 and day 7). From these results observed, the knockout of the gene exoD does not seem to affect the phenotype of the cells within standard cultivation conditions. In , the strains after 4 days of nitrogen and phosphate limitations are shown. All three strains show changes in their phenotype due to the limitation, whereas the phenotype of the knockout strains showed stronger changes. More cell aggregates are visible and the round cell shape changed to be angular. The liquid culture of those transformants became yellowish whereas the control remained slightly green-yellowish during nutrient limitation (not shown). This suggests that the knockout may have an influence on the cells during nitrogen and phosphorus limiting conditions. Nevertheless, the control as well as the transformants recovered from the limitation after transferring them into the standard medium for 24 h. Thus, the knockout causes no deadly effects during limitations compared to knockouts of main storage pathways like glycogen. There, the recovery after the deletion of the gene glgC could not be observed [Citation46]. As the PHB production is increased during nitrogen and phosphate limitation, no lethal changes due to the exoD gene knockout are mandatory for the production.
Figure 4. Impact of exoD gene knockout in the two transformants KOexoD#4 (b, e, h, k, n) and KOexoD#65 (c, f, i, l, o) compared to the control strain Mt_a24 (a, d, g, j, m) under different cultivation conditions showed by scanning electron microscope (SEM) imaging. The samples were prepared by fixing the cells (OD730 = 0.7) in glutaraldehyde overnight followed by three washing steps, Au-spattering and imaging at the TESCAN MIRA3 FEG-SEM microscope. Images a – c, standard cultivation conditions on day 7 (D7); images d – f standard cultivation conditions on day 11 (D11); images g – i nitrogen and phosphorus limited medium on day 11 (D11-NP); images j – l standard cultivation conditions on day 14 (D14); images m – o nitrogen and phosphorus limited medium on day 14 (D14-NP). Black marks (D11) on the cells resulted from the measurement. The pattern on the cell surface results from the Au spatter coating

In , the strains after 14 days under standard conditions are shown. Changes in the cell shape can be observed, suggesting that those cells may be nutrition limited. As the shape of the nutrient-limiting cells () is looking similar to those under standard conditions, this supports the hypothesis of nutrient limitation of the cells in . In order to enhance the cell density prior to nutrient limitation an enriched (2- or 3-fold) BG11 medium could be used to reach high cell densities prior to the PHB production [Citation24], which investigated the EPS production in the strain Synechocystis sp. PCC6803 under standard conditions showed no changes of the phenotype as well. Their generated double mutant, lacking the genes exoD and gumB, seemed to have no negative effects on its phenotype but the double knockout lead to less visible EPS around the cells compared to the wild type. As a result, the additional deletion of gumB within the strains used in this work may show the same results as in the strain Synechocystis sp. PCC6803 and could be the next approach to be tested.
Conclusion
The hypothesis that the knockout of the EPS production may have an influence on the production of storage compounds like PHB and glycogen in cyanobacteria was analyzed within this study. The knockout of the gene exoD, whose influence on the EPS production is still unclear, resulted in an overall increase of EPS production in standard medium with the highest EPS fraction of secreted EPS. In the limited medium, the PHB production was increased and the glycogen production decreased within the transformants, assuming that the gene knockout may influence the production of storage compounds.
As the double knockout of exoD and gumB resulted in an EPS reduction within the Synechocystis sp. PCC6803, this could be used in a further approach to ulteriorly enhance the PHB production in the strain Synechocystis sp. PCC6714 used in this study. Moreover, the targeted genetic modification of the Mt_a24 by the integration of the genes involved in the PHB synthesis or the knockout of competing storage pathways like glycogen have not been done so far. Thus, there is a huge potential for increased PHB production within the Mt_a24 strain, which could enable the use of these organisms in industrial applications. This can not only improve the CO2 balance but also reduce the damage caused by improper disposal.
Acknowledgments
We thank the members of the research group AG Biosciences at the University of Applied Sciences Upper Austria for the helpful discussions.
This work was supported by the FFG under Grant [874108].
We thank the Technische Universität Wien for funding the doctoral college “bioactive”.
Disclosure statement
No potential conflict of interest was reported by the authors.
Additional information
Funding
Notes on contributors
Sandra Mittermair
Sandra Mittermair did her master degree in bio- and environmental technologies at the University of Applied Sciences Upper Austria, Austria, and is a research associate at the University of Applied Sciences Upper Austria, Austria, working in the fields of molecular biotechnology.
Juliane Richter
Juliane Richter is a biologist, did her PhD at the Friedrich-Alexander-University in Erlangen, Germany, and is now working as a Post-Doc at the University of Applied Sciences Upper Austria, Austria, in the fields of molecular biotechnology.
Philipp Doppler
Philipp Doppler is a PhD student within the doctoral college “bioactive” at the Institute of Chemical, Environmental and Bioscience Engineering at Technische Universität Wien.
Kevin Trenzinger
Kevin Trenzinger is a master student in bio- and environmental technologies at the University of Applied Sciences Upper Austria, Austria.
Cecilia Nicoletti
Cecilia Nicoletti did her master degree in chemical engineering at the University of Calabria, Italy, and is working as a research associate at the University of Applied Sciences Upper Austria, Austria, in the fields of bioprocess engineering.
Christian Forsich
Christian Forsich is a chemist and works as a research associate at the University of Applied Sciences Upper Austria, Campus Wels in the field of Materials Technology.
Oliver Spadiut
Oliver Spadiut is associate professor at the Institute of Chemical, Environmental and Bioscience Engineering at Technische Universität Wien.
Christoph Herwig
Christoph Herwig is a bioprocess engineer from RWTH Aachen and the head of the institute of Biochemical Engineering at Technische Universität Wien.
Maximilian Lackner
Maximilian Lackner is a scholar and entrepreneur with experience in the polymer industry and in bioplastics in particular.
References
- European Commission. A European strategy for plastics in a circular economy. zuletzt geprüft am. 2019;11(8):2020.
- Machalaba C, Romanelli C, Stoett P, et al. Climate change and health: transcending silos to find solutions. Ann Glob Health. 2015;81(3):445–458.
- Lackner M. Bioplastics. In: Kirk-Othmer, editor. Inc (Hg.) 2015 – kirk-othmer encyclopedia of chemical technology, Hoboken, NJ, USA: John Wiley & Sons, Inc.; 2015, pp. S. 1–41. doi:https://doi.org/10.1002/0471238961.koe00006.
- Bekker A, Holland HD, Wang P-L, et al. Dating the rise of atmospheric oxygen. Nature. 2004;427(6970):117–120.
- Cardona T. Early Archean origin of heterodimeric Photosystem I. Heliyon. 2018;4(3):e00548.
- Darko E, Heydarizadeh P, Schoefs B, et al. Photosynthesis under artificial light: the shift in primary and secondary metabolism. Philos Trans R Soc Lond B Biol Sci. 2014;369(1640):S. 20130243.
- Dutkiewicz A, Volk H, George SC, et al. Biomarkers from Huronian oil-bearing fluid inclusions: an uncontaminated record of life before the great oxidation event. Geol. 2006;34(6):S. 437.
- Schirrmeister BE, de Vos JM, Antonelli A, et al. Evolution of multicellularity coincided with increased diversification of cyanobacteria and the great oxidation event. Proc Nat Acad Sci. 2013;110(5):1791–1796.
- Szathmáry E, Smith JM. The major evolutionary transitions. Nature. 1995;374(6519):227–232.
- Carpine R, Du W, Olivieri G, et al. Genetic engineering of Synechocystis sp. PCC6803 for poly-β-hydroxybutyrate overproduction. Algal Res. 2017;25:S. 117–127.
- Gundolf R, Oberleitner S, Richter J. Evaluation of new genetic toolkits and their role for ethanol production in cyanobacteria. Energies. 2019;12(18):S. 3515.
- Kamravamanesh D, Lackner M, Herwig C. Bioprocess engineering aspects of sustainable polyhydroxyalkanoate production in cyanobacteria. Bioengineering (Basel). 2018b;5(4). DOI:https://doi.org/10.3390/bioengineering5040111
- Lau N-S, Matsui M, Abdullah -A-A-A. Cyanobacteria: photoautotrophic microbial factories for the sustainable synthesis of industrial products. Biomed Res Int. 2015;2015:S. 754934.
- Hempel F, Bozarth AS, Lindenkamp N, et al. Microalgae as bioreactors for bioplastic production. Microb Cell Fact. 2011;10:S. 81.
- Lackner M, Kamravamanesh D, Krampl M, et al. Characterization of photosynthetically synthesized poly(3-hydroxybutyrate) using a randomly mutated strain of Synechocystis sp. PCC 6714. Int J Biobased Plast. 2019;1(1):48–59.
- Markl E, Grünbichler H, Lackner M. Cyanobacteria for PHB bioplastics production: a review. In: Wong YK, editor. Algae. London, UK: IntechOpen; 2018, p. 1–10. doi:https://doi.org/10.5772/intechopen.81536
- Khetkorn W, Incharoensakdi A, Lindblad P, et al. Enhancement of poly-3-hydroxybutyrate production in Synechocystis sp. PCC 6803 by overexpression of its native biosynthetic genes. Bioresour Technol. 2016;214:761–768.
- Wu GF, Shen ZY, Wu QY. Modification of carbon partitioning to enhance PHB production in Synechocystis sp. PCC6803. Enzyme Microb Technol. 2002;30(6):710–715.
- Kopf M, Klähn S, Pade N, et al. Comparative genome analysis of the closely related Synechocystis strains PCC 6714 and PCC 6803. DNA Res. 2014;21(3):S. 255–266.
- Kamravamanesh D, Kovacs T, Pflügl S, et al. Increased poly-β-hydroxybutyrate production from carbon dioxide in randomly mutated cells of cyanobacterial strain Synechocystis sp. PCC 6714. Mutant generation and characterization. Bioresour Technol. 2018a;266:34–44.
- Fisher ML, Allen R, Luo Y, et al. Export of extracellular polysaccharides modulates adherence of the Cyanobacterium synechocystis. PloS One. 2013;8(9):e74514.
- Pembroke JT, Balakrishnan N, Armshaw P. The potential of the photoautotroph synechocystis for metal bioremediation. In: Shiomi N, editor. Advances in bioremediation of wastewater and polluted soil: inTech. London, UK: IntechOpen; 2015, p. 51–72. DOI:https://doi.org/10.5772/60514.
- Simkovsky R, Daniels EF, Tang K, et al. Impairment of O-antigen production confers resistance to grazing in a model amoeba-cyanobacterium predator-prey system. Proc Nat Acad Sci. 2012;109(41):S. 16678–16683.
- Jittawuttipoka T, Planchon M, Spalla O, et al. Multidisciplinary evidences that Synechocystis PCC6803 exopolysaccharides operate in cell sedimentation and protection against salt and metal stresses. PloS One. 2013;8(2):e55564.
- Shen L, Li Z, Wang J, et al. Characterization of extracellular polysaccharide/protein contents during the adsorption of Cd(II) by Synechocystis sp. PCC6803. Environ Sci Pollut Res Int. 2018;25(21):20713–20722.
- Bahat-Samet E, Castro-Sowinski S, Okon Y. Arabinose content of extracellular polysaccharide plays a role in cell aggregation of Azospirillum brasilense. FEMS Microbiol Lett. 2004;237(2):195–203.
- Fischer SE, Miguel MJ, Mori GB. Effect of root exudates on the exopolysaccharide composition and the lipopolysaccharide profile of Azospirillum brasilense Cd under saline stress. FEMS Microbiol Lett. 2003;219(1):53–62.
- Panhota RS, Bianchini I, Vieira AAH. Glucose uptake and extracellular polysaccharides (EPS) produced by bacterioplankton from an eutrophic tropical reservoir (Barra Bonita, SP–Brazil). Hydrobiologia. 2007;583(1):223–230.
- Pereira S, Zille A, Micheletti E, et al. Complexity of cyanobacterial exopolysaccharides: composition, structures, inducing factors and putative genes involved in their biosynthesis and assembly. FEMS Microbiol Rev. 2009;33(5):917–941.
- Pereira SB, Santos M, Leite JP, et al. The role of the tyrosine kinase Wzc (Sll0923) and the phosphatase Wzb (Slr0328) in the production of extracellular polymeric substances (EPS) by Synechocystis PCC 6803. MicrobiologyOpen. 2019;8(6):e00753.
- Ricciardi A, Parente E, Crudele MA, et al. Exopolysaccharide production by Streptococcus thermophilus SY: production and preliminary characterization of the polymer. J Appl Microbiol. 2002;92(2):297–306.
- Vaningelgem F, Zamfir M, Mozzi F, et al. Biodiversity of exopolysaccharides produced by Streptococcus thermophilus strains is reflected in their production and their molecular and functional characteristics. Appl Environ Microbiol. 2004;70(2):S. 900–912.
- de Vuyst L, Degeest B. Heteropolysaccharides from lactic acid bacteria. FEMS Microbiol Rev. 1999;23(2):153–177.
- Reeves PR, Hobbs M, Valvano MA, et al. Bacterial polysaccharide synthesis and gene nomenclature. Trends Microbiol. 1996;4(12):495–503.
- Hu C. Extracellular carbohydrate polymers from five desert soil algae with different cohesion in the stabilization of fine sand grain. Carbohydr Polym. 2003;54(1):33–42.
- de Philippis R, Sili C, Paperi R, et al. Exopolysaccharide-producing cyanobacteria and their possible exploitation: a review. J Appl Phycol. 2001;13(4):293–299.
- de Philippis R, Vincenzini M. Exocellular polysaccharides from cyanobacteria and their possible applications. FEMS Microbiol Rev. 1998;22(3):151–175.
- Stanier RY, Kunisawa R, Mandel M, et al. Purification and properties of unicellular blue-green algae (order Chroococcales). Bacteriol Rev. 1971;35(2):171–205.
- Formighieri C, Melis A. Regulation of beta-phellandrene synthase gene expression, recombinant protein accumulation, and monoterpene hydrocarbons production in Synechocystis transformants. Planta. 2014;240(2):309–324.
- Mülhardt C. Der Experimentator Molekularbiologie/Genomics. Berlin, Heidelberg: Springer Berlin Heidelberg; 2013.
- Ludwig A, Heimbucher T, Gregor W, et al. Transformation and gene replacement in the facultatively chemoheterotrophic, unicellular cyanobacterium Synechocystis sp. PCC6714 by electroporation. Appl Microbiol Biotechnol. 2008;78(4):729–735.
- Kamravamanesh D, Pflügl S, Nischkauer W, et al. Photosynthetic poly-β-hydroxybutyrate accumulation in unicellular cyanobacterium Synechocystis sp. PCC 6714. AMB Express. 2017;7(1):S. 143.
- Kamravamanesh D, Slouka C, Limbeck A, et al. Increased carbohydrate production from carbon dioxide in randomly mutated cells of cyanobacterial strain Synechocystis sp. PCC 6714: bioprocess understanding and evaluation of productivities. Bioresour Technol. 2019;273:277–287.
- Schirmer M (2015): Bestimmung und Optimierung verfahrenstechnischer und metabolischer Kenngrößen bei photoautotrophen Mikroorganismen unter Verwendung nichtlinearer Optimierungsalgorithmen. Dissertation. Friedrich-Alexander-Universität Erlangen-Nürnberg, Erlangen. Lehrstuhl für Bioverfahrenstechnik.
- Yoo S-H, Keppel C, Spalding M, et al. Effects of growth condition on the structure of glycogen produced in cyanobacterium Synechocystis sp. PCC6803. Int J Biol Macromol. 2007;40(5):498–504.
- Grundel M, Scheunemann R, Lockau W, et al. Impaired glycogen synthesis causes metabolic overflow reactions and affects stress responses in the cyanobacterium Synechocystis sp. PCC 6803. Microbiology. 2012;158(Pt 12):S. 3032–3043.