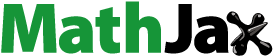
Abstract
Indonesia has the potential to develop biomass, such as MSW and wood pellets, as a source of energy. Syngas from biomass gasification can be used as fuel for dual-fuel diesel engines in order to transition energy. We investigated the gasification of MSW and wood pellets using a downdraft reactor with a capacity of 130–144 kg in order to determine the properties of the produced syngas for use in dual-fuel syngas diesel engines. Four days are dedicated to the process of preheating biomass with sunlight. The experiment was designed with an equivalent ratio of 0.22 and an oxidation zone temperature between 700 and 900 degrees Celsius. According to the experimental results, the average lower heating value of syngas produced by the air gasification of MSW biomass was 5.85 MJ/Nm3, the cold gas efficiency was 56.6%, and the carbon conversion efficiency was 94.77%. The average lower heating value from wood pellet biomass air gasification was 5.95 MJ/Nm3, the cold gas efficiency was 67.7%, and the carbon conversion efficiency was 94.28%. Solar heating of biomass has been shown to increase the calorific value of biomass gasification to syngas.
1. Introduction
Immediate energy requirements are satisfied by fossil fuels, particularly coal, petroleum, and natural gas. Increasing demand for fossil energy, diminishing fossil energy reserves, environmental concerns, and reduction objectives for carbon emissions. The Indonesian government’s participation in the Paris Agreement has facilitated the shift to new and renewable energy (BPPT, Citation2021). Solar, wind, geothermal, and biomass are some of the new and renewable energy sources that are being developed extensively for the energy transition (Situmorang, Zhao, Yoshida, Abudula, & Guan, Citation2020). In Indonesia, the government encourages the use of a new renewable energy mix to reach 23% of total usage by 2025 and 31% of primary energy requirements by 2050 (DEN, Citation2019). According to Ariffin et al. (Citation2017), biomass is the only renewable energy source capable of meeting about 9% of the world’s primary energy demand (Sharma, Sharma, Pulla, & Sahoo, Citation2020). Oil palm kernel (33%), MSW (32%), agricultural waste (24%), and wood (11%) are examples of biomass that have the potential to be utilized as a source of electrical energy. In the meantime, 82% of the most widely utilized technology is co-firing technology and 5% is gasification (Primadita, Kumara, & Ariastina, Citation2020). Biomass gasification generates syngas, which can be utilized as a fuel for gas turbines and combined cycles. Dual-fuel diesel engines can decrease diesel consumption by 44–60% (Sudarmanta, Citation2010). Consequently, further exploration of the use of gasified syngas as a secondary fuel for diesel engines is warranted.
Gases such carbon monoxide (CO), carbon dioxide (CO2), hydrogen (H2), methane (CH4), unburned carbon (unburned C), and light hydrocarbons (LHC) are produced during the thermal decomposition of solid biomass in the process of biomass gasification (Puig-Arnavat, Bruno, & Coronas, Citation2010). The syngas composition is highly sensitive to the kind of feedstock (biomass), gasification technology, and operating parameters (Molino, Larocca, Chianese, & Musmarra, Citation2018). Updraft gasifiers, downdraft gasifiers, and fluidized bed gasifiers are the three most popular types of gasification reactors (Susastriawan, Purwanto, & Purnomo, Citation2021). The commercial gasification process utilizes 75% downdraft gasifiers, 20% fluidized beds, 2.5% updraft, and 2.5% alternative variants (Ruiz, Juárez, Morales, Muñoz, & Mendívil, Citation2013). Because of its low tar content and ease of operation, downdraft gasifier reactor technology is frequently utilized for heating and small to medium-scale thermal generation (Saleh, Sudarmanta, Fansuri, & Muraza, Citation2020). Low efficiency is a disadvantage of using a downdraft reactor, and it is difficult to produce syngas when the moisture and ash level are high (Patra & Sheth, Citation2015). Adding heated air is a great technique to improve gasifier performance (Susastriawan, Saptoadi, & Purnomo, Citation2017), furthermore, a tar-cleaning reactor can advantage from integrated gasification, cooling, and cleaning operations (Heidenreich & Foscolo, Citation2015). The reactor gasifier, cyclone, water scrubber (refinery and cooling unit), and dry filter can all be combined into a single gasification reactor unit to accomplish this. Small and medium-sized thermal power plants can benefit from throatless downdraft gasifier technology since it is simple in concept, production, and evaluation (Susastriawan et al., Citation2017).
In accordance with the National Energy General Plan (RUEN), one of the energy transition program implementations in Indonesia is the conversion of diesel power plants to dual-fuel diesel-syngas power plants. Some study indicates that diesel engines can use gasified syngas with a downdraft reactor as a dual-fuel fuel. Have demonstrated a downdraft reactor gasification efficiency of around 80% and a gas producer calorific value of 4.5 MJ/kg. In addition, syngas can lower fuel consumption by 75% and can be used to measure the program’s potential for success (Susastriawan et al., Citation2017). According to the results of reactor downdraft gasification, pine wood pellets contain more carbon monoxide (CO), hydrogen (H2), and methane (CH4) than pine chips do. The cold gas efficiency (CGE) of pine wood pellets was 90% at an ER of 0.27 at a temperature of 850-950 °C. Pinecone gasification at an ER of 0.28 and temperature of 900–950 °C resulted in a CGE of 78% (Aydin, Yucel, & Sadikoglu, Citation2019). Using various ER and hot air temperatures, the best parameters for the gasification of MSW pellets in downdraft gasifier reactors were obtained by through experimentation. Thus, a syngas with a lower heating value (LHV) of 5254 to 5976 MJ/kg was produced (Saleh, Sudarmanta, Fansuri, & Muraza, Citation2019).
Biomass gasification of switchgrass with a feedstock LHV of 16.49 MJ/kg, ER of 0.2, and temperature of 800–900 °C produced syngas with a high heating value (HHV) of 6.47 MJ/Nm3, with a maximum syngas output of 5 kW from a 10-kW power plant (Indrawan, Thapa, Bhoi, Huhnke, & Kumar, Citation2017). The gasification of MSW feedstock pellets with an HHV of 13.84 MJ/kg at an ER of 0.20 can replace 44% of diesel consumption (Sudarmanta, Sampurno, Dwiyantoro, Gemilang, & Putra, Citation2019). Wood pellet gasification with a downdraft gasifier and a syngas LHV of 4.76 MJ/Nm3 can reduce diesel consumption by 23% (Patra & Sheth, Citation2015). Downdraft gasification of walnut shells with HHV feedstock of 16.72 MJ/kg, calorific value of syngas of 7.30 MJ/Nm3, maximum diesel consumption of 58.18% with a 3.46 kW diesel engine (Sharma & Kaushal, Citation2020). The calorific content of the producing gas effects the diesel power plant’s specific fuel consumption (SFC), according to the review article above. Depending on the type of biomass, diesel consumption can be reduced by 44–60% with an HHV feedstock value of 4–7.30 MJ/kg.
Biomass is characterized by its low density and calorific value, as well as its high proportions of fixed carbon, volatile matter, and low ash content. Around 75–85% of the stuff produced by biomass is volatile, whereas the rest consists of fixed carbon and ash. At least 16% of the fixed carbon in biomass is utilized as an energy source (Basu, Citation2013). Carbon (C), oxygen (O), hydrogen (H), sulfur (S), nitrogen (N), and ash all influence the calorific value of feedstock. The greater the amounts of C, H, and S and the lower the levels of O and ash, the higher the calorific value of the feedstock (Channiwala & Parikh, Citation2002). Torrefaction, carbonization, and densification are all ways to increase the density and calorific value of feedstock. High-quality biomass solid fuel can be made through densification and torrefaction at temperatures between 200 and 300 °C. The process leads to a decrease in moisture levels, an increase in O/C and a decrease in H/C ratios, and a rise in fixed carbon content. When carbon is present, the oxygen consumption drops, leading to higher rates of cold gas efficiency (CGE) and carbon conversion efficiency (CCE) during gasification (Chen et al., Citation2018; Chen, Peng, & Bi, Citation2015; Ding et al., Citation2018; Umeda, Nakamura, Lu, & Yoshikawa, Citation2019). Valuable gases with a CGE of 72–77% and a calorific value of 4.8–5.8 MJ/kg are produced during the gasification and torrefaction of wood pellets (Dudyński, Van Dyk, Kwiatkowski, & Sosnowska, Citation2015).
The majority of processed wood and municipal solid waste (MSW) biomass still has a substantial amount of moisture. Thus, biomass must be dried before being gasified (Nyakuma et al., Citation2023). By decreasing the oxygen to carbon (O/C) and hydrogen to carbon (H/C) ratios and raising the calorific value of feedstock through carbonization at temperatures between 200 and 300 °C, the HHV of syngas, CGE, and CCE are increased (Chen et al., Citation2015, Citation2018; Ding et al., Citation2018; Dudyński et al., Citation2015; Umeda et al., Citation2019). Indonesia is not a suitable option for carbonization due to its tropical location, abundant sunlight, and vast number of rural areas. It is possible to boost the physical, chemical, thermal, and calorific value of biomass by preheating it. Sunshine can be used as a source of preheating. To reduce or eliminate the moisture content of biomass, a pre-treatment method was developed (Nyakuma et al., Citation2023). According to the existing review literature, there is no pretreatment process for drying biomass using sunlight. Using a solar shell, sunlight is commonly utilized in the pyrolysis process (Rahman, Parvej, & Aziz, Citation2021; Rajendran et al., Citation2021). As an alternative to carbonization, solar pretreatment can minimize moisture and volatile matter while enhancing fixed carbon.
This is the first of two research investigated the use of biomass pellets made from wood and MSW to generate syngas for remote dual-fuel power plants. Here, we report the potential features of syngas produced by the gasification of wood and MSW pellets carbonized in sunlight for four days at temperatures above 60 °C in a 145-kg gasifier downdraft reactor. This reactor was created as a prototype for a program to convert diesel power plants to dual-fuel power plants with a capacity of 20 kW or more. The purpose of this section is to evaluate whether the produced syngas, CGE, and CCE have a calorific value that might be used to replace solar energy in diesel engines.
2. Materials and methods
2.1. Characterization of biomass
The biomass materials used in this study were wood pellets and MSW. One of the characteristics considered when choosing pellets was their high density, which can reduce health hazards for operators. Wood pellets are constructed from sawdust and woodchips. Hardwood and softwood are the primary materials utilized to manufacture wood pellets. Teak (Tectona grandis), mahogany (Ssietenia mahagony), and acacia are examples of hardwoods (Acacia auriculiformis). The species of softwood used for sengon (Paraserianthes falcataria). Teak sawdust (T. grandis) has a density of 0.72 to 0.82 (g/cm)3 and a calorific value of 4,888.81 cal/g, while acacia wood (A. auriculiformis) has a density of 0.71 to 0.79 (g/cm)3, sengon wood (paraserianthes falcataria) density 0.58 − 0.66 (g/cm)3 with calorific value (3726.08–4003.84) cal/g.
The raw material for MSW pellets was urban waste collected from the Institut Teknologi PLN campus. MSW pellets were made in four stages, as shown in . In , the first stage of waste selection, urban waste was separated according to the type of organic waste (food waste, leaves, and wooden branches) and non-organic waste (paper and plastic). The second process is bio-drying by utilizing micro-organisms in the bio-activator. EM4 or eco enzyme is the bio-activator liquid used. This concentrate is thawed at a 1:20 ratio. Organic matter decomposition will be carried out aerobically by microbes. After mixing bio-enzymes, the biomass is covered in plastic as part of the bio-drying procedure. This activity generates heat, which reduces the water content of the material. This process involved pouring a bio-activator (bacterial) liquid that softened and shrunk the litter to 50%. All the organic and inorganic garbage was put into an open-topped bamboo cage and dried using the bio-drying method. The bio-drying method was performed by placing the entire organic and inorganic waste into a bamboo cage whose top was exposed and flushed with a bio-activator. After 5–10 days, the litter was completely dry (). As a third step, we tallied all of the pieces by hand and with a cutting machine. The standard size according to SNI 8961:2021 is 3–4 cm in length and 10 mm in diameter (). In the fourth stage, the waste was mixed with an adhesive or aggregate and then formed into pellets or briquettes. ().
Figure 1. The manufacturing process of MSW pellets (a) MSW sorting, waste selection to the type of organic waste and non-organic waste (b) bio-drying by utilizing microorganisms in the bio-activator, (c) chopping process with cutting machine, (d) making MSW pellets.

shows a representative sample of wood pellets and MSW. The average length and diameter of wood pellets were 32 mm and 9 mm, but they were 35 mm and 10 mm for MSW. The biomass was sun-dried for 25 h prior to the proximal and ultimate testing. Using the ASTM D 5376-16 infrared and thermal conductivity detector method, the ASTM D.4239-14 sulfur content standard, and the D.3176-15 oxygen content standard, the C, H, and N contents were examined. Proximate analysis helps detect moisture using ASTM D.3302-17, ash, volatile matter, and fixed carbon levels using ASTM D.7581-15 a thermal gravimetric analyzer (TGA), sulfur analysis with high-temperature combustion, and infrared conversion analysis. Both proximal and ultimate analyses were conducted in the laboratory of PT PLN Pusertif; the results are shown in .
Table 1. Proximate and ultimate biomass test results and reference.
The results of the proximate and ultimate tests, as well as the calorific value of the biomass, are presented in alongside previously published biomass test results. This study compared the biomass characteristics of proximate and final test results to previously published research. The characteristics of MSW test results will be compared to those of MSW that was not initially treated (Saleh et al., Citation2020) and MSW that was torrefied (Chen et al., Citation2020). The characteristics of wood pellets test results will be compared to those of wood pellets without initial treatment and with torrefaction (Dudyński et al., Citation2015). Before biomass was subjected to both proximate and ultimate tests, it was sun-dried for 24 h or 4 days at 60 °C to reduce moisture and volatile matter and enhance levels of fixed carbon. shows that the moisture content of MSW and wood pellets that have been preheated is lower than that of MSW and wood pellets that have not been preheated. In the meantime, the ash content of MSW biomass reached above 10%, which is one of MSW biomass’s downsides. The effect of sunshine on the levels of moisture and volatile matter was greater than that of biomass that underwent torrefaction or carbonization, and the levels of fixed carbon were lower, but the ash content rose.
2.2. Gasification unit set-up
In this experiment, a downdraft gasifier was used as the reactor. The downdraft reactor was selected for this gasification process due to its suitability for small-scale plants, its simple design, high carbon conversion, low tar content, and comparatively low tar cleaning complexity requirements. Among the disadvantages that must be overcome during the gasification process are low efficiency and acceptable feedstock, the need for biomass with a low moisture content, a rather complicated starting procedure, and temperature control (Molino, Chianese, & Musmarra, Citation2016). depicts the facilities of Institut Teknologi PLN of Technology’s Biomass and Waste Gasification Laboratory, Faculty of Energy Technology and Business.
Figure 3. Sketch of biomass gasification reactor facility at the gasification Laboratory and MSW Faculty of Energy Technology and Business, Institut Teknologi PLN, Jakarta.

is a schematic depicting the major component of this research test equipment, which is a downdraft reactor with visible dimensions. There is a diagram with four thermocouples of type K. The gasifier was equipped with a cleaning aid for syngas consisting of a cyclone, water scrubber, filter, and storage reservoir for syngas. Moreover, thermocouples were inserted after the reactor, cyclone, water scrubber, and filter. In the water scrubber, water was utilized as the cooling medium, while activated carbon and wood charcoal were used as the filter’s media. The capacity of the reactor was 150 kg, which was anticipated to burn out in 12 h. In the reactor, a 1.5 kW, 230 VAC, 50 Hz, 6.5 A compressor with a capacity of 220 liters per minute was installed. The water scrubber was supplied with a pump that loaded water at a rate of 50 revolutions per minute. To suction the syngas in the reactor, a suction blower pump with 0.25 kW of suction pump power and 1000 CMH of airflow was added.
2.3. Experimental methods
The biomass was dried and stored after the humidity was measured using an Aory Moisture Meter of type YBG LM15, following a four-day solar heating process that brought the material to an internal temperature of 60 °C. In the second phase, we made sure that everything was running smoothly by double-checking the temperature gauge, compressor, filter, water scrubber, and pump. For preheating, as much as 10 kg of charcoal was burned on the outskirts of the reactor. After 15–30 min, coal containing charcoal was introduced to the reactor. As the oxidation zone temperature hit 300 °C, the biomass was fed into the reactor progressively until its volume reached the maximum allowed above the drying zone’s observation point, or about 134 kg. Compressed air was fed into the reactor at a rate of 180–220 liters per minute to maintain a constant combustion process in the oxidation zone. summarizes the gasification process’s operational parameters. At 700, 750, 800, 850, and 900 °C, we took samples of syngas at various pressures and temperatures. The number of trials in this study was one for each sample because it was difficult to replicate the same temperature conditions. One of the major issues and problem combustion in gasification research is that it is difficult to control the temperature and produce the same conditions every time. The temperature of gasification is highly dependent on the combustion process in the gasifier reactor. If the temperature is stable, the sampling method is used, and data collection is completed without repetition. The gas analyzer’s performance was measured using a time series of test data. We utilize the highest possible reading from the gas analyzer’s tests as our data. For this analysis, we used a Cubic Ruiyi G-3100P Gas Analyzer on the gas samples.
Table 2. Summary of gasification operating parameters.
The gas analyzer tool outputs time series data from syngas composition testing results. Six data points are obtained from the gas analyzer output data at each sampling, and the average value and standard deviation are calculated to display the optimal generated gas. Validation of measurement data was performed at ER 0.22 under five distinct temperature conditions. The five measurement data points are used to determine the mean and standard deviation. The validation of the average results of syngas composition measurements at a specific ER (ER = 0.22) is compared to the results of previous published research with an ER close to 0.22. The root mean squared error (RMSE) method is used to validate measurement data.
2.4. Data processing methods
The ER value is defined as the ratio of the total amount of oxygen used to the amount of stoichiometric oxygen (Basu, Citation2013):
(1)
(1)
where AFRact (kg/kg) is the actual amount of air used in the gasification process and AFRstoich (kg/kg) is calculated by using EquationEq. (2)
(2)
(2) (Basu, Citation2013):
(2)
(2)
The percentages of carbon, hydrogen, oxygen, and sulfur determined from the ultimate analysis are C, H, O, and S, respectively. Using the method outlined by Homdoung, Dussadee, Sasujit, Kiatsiriroat, and Tippayawong (Citation2019), the gas producer rate (Nm3/h) can be estimated from the nitrogen balance.
(3)
(3)
where Qg is the yield gas rate of the producer (Nm3/kg), Qa is the air flow rate (Nm3/h), mf is the biomass flow rate (kg/h), and N2% is the volume fraction of nitrogen in the dry gas products. Carbon conversion efficiency (ηC) is computed as the total of the percentage of carbon transformed into syngas using the following formula (Erlich & Fransson, Citation2011):
(4)
(4)
where CO%, CO2%, and CH4% are the percentages of gas volume in syngas and C% is the percentage of carbon from the ultimate test.
Cold gasification efficiency is defined as the ratio of the total amount of low calorific value of syngas to the low calorific value of biomass feedstock. The efficiency of cold gaseous gasification (ηCGE) was calculated using the following formula
The efficiency of cold gasification is measured as the ratio of the total amount of low calorific value syngas to the low calorific value of biomass fuel. The following formula was used to determine the efficiency of cold gaseous gasification () (Umar et al., Citation2021):
(5)
(5)
where Qg represents the producer yield gas rate (Nm3/kg), LHVg represents the low calorific value of the gas (MJ/Nm3), and LHVf represents the low calorific value of biomass (MJ/kg). Both the HHVf and the LHVf were determined by applying the formula and then recalculating it while taking into account the correlation that Channiwala and Parikh (Citation2002) (MJ/kg):
(6)
(6)
where mC, mH, mS, mO, mN, and mAC are the mass percentages of carbon, hydrogen, sulfur, oxygen, nitrogen, and ash from the ultimate and proximate analyses. The LHVf value of the feedstock was calculated using the following equation shown below (Basu, Citation2013):
(7)
(7)
where hg is the steam’s latent heat (2.26 MJ/kg). The lower calorific value of LHV syngas was calculated using Equation for LHVg (MJ/Nm3) Equation(8)
(8)
(8) (Kurkela, Kurkela, & Ilkka, Citation2014):
(8)
(8)
CO, H2, CH4, and CnHm are the percentages of the volume fractions of hydrogen, carbon monoxide, methane, and light hydrocarbons, respectively.
3. Results and discussion
3.1. Effect of preheating on biomass characteristics
The type of biomass, particle size, moisture and ash contents, and the resulting syngas from biomass gasification are all factors. Pollutants like nitrogen oxide and sulfur oxide are known to be produced from the sulfur and nitrogen levels in biomass (Molino et al., Citation2018). The chemical composition of the outline biomass consists of 40–58% cellulose (C6H10O4), 10–40% hemicellulose (C5H8O5), and the rest is lignin–(27–28%). Lignin consists of lignin-C (C15H14O4), lignin-O (C20H22O10), and lignin-H (C22H28O9) (Lee, Alam, Han, Choi, & Park, Citation2020). Approximately four days of heating influenced the properties of a sample of biomass.
As can be seen in , preheating results in lower oxygen-to-carbon (O/C) and hydrogen-to-carbon (H/C) ratios than does not preheating. Low-temperature carbonization, as indicated, decreases the O/C and H/C ratios, as reported by according Ding et al. (Citation2018), Dudyński et al. (Citation2015), and Umeda et al. (Citation2019). As a result of solar preheating, the O/C and H/C ratios decreased, indicating a decrease in moisture, oxygen, and hydrogen levels in the biomass of MSW and wood pellets while an increase in fixed and molar carbon. The O/C and H/C ratios were found to be in the middle ground between untreated and treated biomass after heat treatment with sunshine. After being heated, the oxygen to carbon ratio in biomass wood pellets was 0.54:1, but in MSW biomass it was 0.5:1, indicating a significant difference in the composition of the two biomasses. Research from Trinh and Uemura (Citation2019), demonstrates that this location of the O/C and H/C ratios indicates that biomass includes a relatively high ratio of cellulose and hemicellulose to lignite, and that this ratio contributes to the efficient generation of syngas (Molino et al., Citation2018). After heating the two biomasses with sunlight, the ratio of O/C = 0.54, H/C = 1.32 for biomass wood pellets and O/C = 0.50, H/C = 1.35 for MSW biomass was determined. However, the cellulose and hemicellulose to lignite ratios were relatively high, indicating that the O/C and H/C ratios were still in the interval between the cellulose and hemicellulose categories. As a result, heating with sunlight has no effect on the cellulose and hemicellulose content of MSW and wood pellets.
shows the effect of the O/C ratio to the HHV of biomass feedstock; the higher the O/C percentage is, the lower the HHV of the feedstock is, and vice versa (Chen et al., Citation2020; Dudyński et al., Citation2015; Saleh et al., Citation2020). The O/C ratio of wood pellets was more than that of MSW biomass; the calorific value of HHV biomass from MSW was greater than that of wood pellets and was greater than the calorific value of biomass without preheating (Lee et al., Citation2020; Saleh et al., Citation2020; Sharma & Kaushal, Citation2020). This higher HHV value was confirmed by the results of previous studies (Dudyński et al., Citation2015; Umeda et al., Citation2019). Preheating with sunlight has an effect on the low O/C ratio, which raises the HHV value of the biomass feedstock without significantly decreasing the quantities of cellulose and hemicellulose.
Figure 5. Effect of O/C ratio to higher heating value feedstock (HHVof MSW, wood pellets, and reference biomass.

According to EquationEq. (6)(6)
(6) , solar preheating decreases the mole fractions of oxygen and hydrogen, moisture content, and volatile matter while increasing the carbon mole fraction and ash content, resulting in a rise in the calorific value of the HHV feedstock. In this study, the O/C and H/C ratios were lower than those of Dudyński et al. (Citation2015) and Saleh et al. (Citation2020), in which the biomass used in the gasification process was not preheated, but higher than those of Chen et al. (Citation2020) and Dudyński et al. (Citation2015), in which the preheating requirement for the used biomass was up to 300 °C. This study demonstrates that solar preheating is highly effective at reducing the O/C and H/C ratios, moisture content, volatile matter, and increasing the value of fixed carbon. Biomass gasification has the ability to raise the calorific value of syngas by increasing the calorific value of the feedstock. This occurs as a result of alterations in the composition of CO, CH4, and H2 gases, those characteristics of biomass () influence the calorific value and yield of syngas. These results are relevant to the correlation founded by Chen et al. (Citation2020), Nimmanterdwong, Chalermsinsuwan, and Piumsomboon (Citation2020) and Subramanian, Sampathrajan, and Venkatachalam (Citation2011). Finally, it has the potential to be used as a diesel fuel substitute in dual-fuel syngas diesel engines substitution.
3.2. Effect of temperature on the composition of gasification results
Gasifier temperature is a crucial variable in the air-based gasification process (Sidek, Abdul Samad, & Saleh, Citation2020). The temperature of the oxidation zone has an effect on the concentrations of CO, CO2, CH4, and H2 produced by gasification. If the temperature increases, the concentration of CO gas increases, the concentration of CO2 tends to decrease, and H2 and CH4 reach their maximum concentrations at a given temperature (Atnaw, Sulaiman, & Yusup, Citation2013). As indicated in , the gasification gas formation complex can normally be characterized as a succession of processes (Chen et al., Citation2015; Saleh et al., Citation2020). CO2 gas formation occurs in the combustion and reduction zones of the water gas reaction shift, which happens between 700 and 900 °C, whereas CO, H2, and CH4 formation occurs in the reduction zone, when temperatures rise over 850 °C.
Table 3. Reactions and their types in gasification processes (Basu, Citation2013).
According to , if the temperature in the gasification reactor rises, the interaction between carbon and oxygen in the oxidation zone, the reaction between carbon and carbon dioxide gas, and the interaction between carbon and water vapor in the reduction process will increase hydrogen gas and carbon monoxide production. In a similar manner, in the reduction zone, the reactions between methane and carbon dioxide and methane and water vapor create CO and H2 gases. At temperatures above 850 °C, hydrogen and carbon dioxide gas formation predominated, whereas carbon dioxide formation predominated at lower temperatures. As the gasifier temperature grew between 700 and 950 °C, the volume of carbon dioxide generation tended to decrease. At increasing temperatures, methane gas production reduces (Aydin et al., Citation2019). These combustible gases are advantageous as dual fuels in diesel engines or engine gases (Chen et al., Citation2020; Sudarmanta, Citation2010; Sudarmanta et al., Citation2019).
shows the characteristics of biomass gasification gas consisting of CO, CO2, CH4, and H2 gases without N2 content and water vapor at temperatures of 700 °C, 750 °C, 800 °C, 850 °C, and 900 °C at ER = 0.22 using biomass as (a) MSW and (b) wood pellets. The composition of gases resulting from air gasification was very different, and the characteristics of the feedstock strongly influenced the composition of H2 and CO2 gases. The gas composition obtained from the gasification of MSW biomass varied from 24.15–27.57%, 15.79–8.89%, 3.35–3.97%, and 9.98–13.10% for CO, CO2, CH4, and H2, respectively. The gas composition obtained from wood pellet biomass was 20.89–24.98%, 16.74–18.43%, 3.61–3.89%, and 14.30–16.91% for CO, CO2, CH4, and H2, respectively. In the gases resulting from biomass gasification for both feedstocks, when the temperature rose, the gas composition of CO and H2 rose, CO2 tended to decrease, and CH4 was relatively constant. This increase in the gas compositions of CO and H2 jika temperature naik ini was consistent with the results of previous studies (Atnaw, Kueh, & Sulaiman, 2014; Calì et al., Citation2020; Ependi, Saleh, & Sudarmanta, Citation2019; Pio & Tarelho, Citation2020; Rupesh, Muraleedharan, & Arun, Citation2015; Umar et al., Citation2021). The oxidation and reduction zone reactions explain the increase in CO and H2 gases and the reduction in CO2 concentrations owing to the temperature increase.
Figure 6. The effect of reactor gasifier temperature on gas composition results in air gasification with biomass from (a) MSW and (b) wood pellets at ER = 0.22.

If the temperature rises, the volume of CO2 and CH4 gases will decrease, while the volume of CO and H2 gases will increase. This statement can be justified from the correlation developed by Pio and Tarelho (Citation2020):
(9)
(9)
(10)
(10)
(11)
(11)
Another justification can be using developed correlations by Rupesh et al. (Citation2015):
(12)
(12)
(13)
(13)
(14)
(14)
(15)
(15)
According to EquationEqs. (9)–Equation(15)(15)
(15) , under constant T conditions, as the amount of air injected into the reactor increases, perfect combustion occurs. The volume of CO2 gas increases while the volume of H2, CO, and CH4 gases decreases. If the ER is kept constant less than one, there is no combustion of the sprinkle during the gasification process. As the temperature rises, the oxidation process produces a large amount of carbon, resulting in more CO and H2 gas being formed during the reduction process than CO2 and CH4 gases.
According to and the correlation of EquationEqs. (9)–Equation(15)(15)
(15) , the ER temperature effects the composition of the syngas yield in addition to the ER temperature. compares the mean and standard deviation of measurement results at ER = 0.22 to published results from the study. The standard deviation of gasification measurement resulted in the highest MSW biomass in carbon dioxide gas composition and the highest wood pellet biomass in nitrogen gas. The average gasification gas production composition for MSW syngas biomass is similar to the research results of Saleh et al. (Citation2020). The average deviation is RMSE 4240, which is less than 10%. When compared to the results of Dudyński et al. (Citation2015), gasification with wood pellet biomass yields syngas that is close to biomass with pre-treatment. The average deviation error (RMSE 7053) is less than 10%.
Table 4. Comparison of experimental (ER = 0.22) and published (ER = 0.20) results from other studies.
Based on an average of the gas composition, the MSW biomass gasification product contained more carbon monoxide gas than wood pellets. Wood pellet biomass gasification produced significantly more CO2, CH4, and H2 with a lower MSW gas percentage. MSW biomass had lower O/C and H/C ratios than wood pellets. The effect of O/C and H/C on the increase in gas composition H2 can be justified from the correlation of Nimmanterdwong et al. (Citation2020)
(16)
(16)
While the effect of H/C on the concentration of CO and CO2 gas volumes can be justified using the correlation of Buragohain, Mahanta, and Moholkar (Citation2012)
(17)
(17)
(18)
(18)
According to EquationEq. (16)(16)
(16) , the H2 concentration of wood pellets is greater than MSW because the ratio of O/C and H/C of wood pellets biomass is greater than MSW. Meanwhile, a high H/C ratio will increase the concentration of lower CO volume and higher CO2 concentration, according to EquationEqs. (17)
(17)
(17) and Equation(18)
(18)
(18) .
Results of MSW gasification at 700 °C and 900 °C showed considerable differences from the norms for CO, CO2, and CH4 gases, whereas variances for CH4 gases were minimal. Gasification results using MSW biomass indicated only significant variations in CO gas, suggesting that this was the only gas that was significantly affected by the increase in gasification temperature (). Understanding how syngas is created during biomass gasification relies heavily on this interaction. As CO and H2 gas generation are more prominent at temperatures above 850 °C, the concentration of these gases tends to increase, while the concentration of CO2 tends to decrease. The gases produced from biomass gasification are influenced by the gasifier’s temperature because of the carbon processes that take place in the oxidation and reduction zones.
This study’s results for CO, H2, and CH4 gas generation were higher than those of prior research that did not involve preheating of biomass (Ependi et al., Citation2019), but lower than those of Chen et al. (Citation2020) due to the influence of preheating. Gasification of biomass wood pellets, however, yielded various outcomes. Research into gasification with wood pellets and torrefaction treatment reportedly did not yield noticeably different gas producers (Dudyński et al., Citation2015). While the H2 and CH4 concentrations in the syngas were higher than in Dudyński et al. (Citation2015), the CO concentrations were lower in this study due to preheating. These results suggest that preheating with sunlight is more efficient in increasing the composition of syngas when using MSW biomass as opposed to wood pellets. Previous research have shown that as temperature increases and the H/C ratio drops, CO gas increases and CO2 declines (Erlich & Fransson, Citation2011; Saleh et al., Citation2020), and this conclusion is supported by these results.
3.3. Effect of temperature on LHV and production rate of syngas
Biomass conversion is a significant parameter in the gasification process; it is defined by the proportion of feedstock converted to gas producer. The LHV and yield of the gas production might be utilized as an indicator. As volatile matter increases, volatile matter and carbon are essential in producing gas output. The gas yield increases according to the gasification temperature (Umar et al., Citation2021). The calorific LHV of syngas is determined by the concentration of gases produced from biomass gasification, particularly CO, H2, and CH4. The airflow rate simultaneously impacts the yield rate of producing gases, the feedstock flow rate, and the nitrogen mass balance. The value of the yield gas is determined by the homogeneous, heterogeneous, exothermic, and endothermic chemical reactions that occur in the gasification zone. Hence, the rate of producer gas production is indirectly dependent on the gas concentration produced by biomass gasification. Gasification cannot be centralized since gas output is dependent on various difficult-to-control characteristics, including as the ER, temperature, pressure, reactor design, and gasification process duration.
shows the impact of gasifier temperature on syngas calorific LHV and producer gas yield rate for the gasification process with biomass from (a) MSW and (b) wood pellets. The LHV of syngas gasified from MSW increased from 5.32 MJ/Nm3 at 700 °C to 6.18 MJ/Nm3 at 900 °C, with an average of 5.85 MJ/Nm3. It increased from 5.47 MJ/Nm3 at 700 °C to 6.26 MJ/Nm3 at 900 °C for wood pellets, with an average of 5.95 MJ/Nm3. The LHV of syngas from wood pellets increased significantly from 700 °C to 850 °C, which can be attributed to a decrease in CO2 concentration and an increase in CO and CH4 concentrations, whereas the efficacy of syngas from MSW was not significant. The temperature effect on LHV can be justified by the correlation of research results from Ariffin, Wan Mahmood, Mohamed, and Mohd Nor (Citation2016), Pio and Tarelho (Citation2020) and Rupesh, Muraleedharan, and Arun (Citation2020), are:
(19)
(19)
(20)
(20)
(21)
(21)
Figure 7. Effect of reactor temperature on LHV and gas output (Qg) using biomass from (a) MSW and (b) wood pellets at ER = 0.22.

According to EquationEqs. (19)–Equation(21)(21)
(21) with constant ER, if the temperature increases, so LHV will also increase. LHV is affected by factors than temperature and ER. There are other correlations that can be explored; examples from Buragohain et al. (Citation2012) are:
(22)
(22)
The average LHV of syngas from wood pellets was higher than that of MSW, which can be explained by the wood pellets’ H2/CO ratio of 0.48, which was higher than that of MSW at 0.46. According to the research results of Hu et al. (Citation2019), the effect of the H2/CO ratio can be justified because the higher the H2/CO ratio, the higher the LHV value. The average concentration of CH4 is the second influencing factor, as the gasification of wood pellets was greater than that of MSW. According to the feedstock characteristics of the proximate test results, the percentage of volatile matter and fixed carbon in wood pellets was higher than in MSW, and the content of CH4 and H2 in wood pellets was higher than in MSW. These two factors explain why syngas from wood pellets had a higher average LHV than MSW. According to EquationEq. (22)(22)
(22) , the factor that causes LHV wood pellets to be higher than MSW is a lower H/C ratio of wood pellets than MSW (), indicating that the impact of LHV is greater. In comparison to previous studies, the average LHV of syngas from MSW was higher than that reported by Noma et al. (Citation2012), and comparable to the results of Saleh et al. (Citation2019). At ER = 0.3, the LHV of syngas from wood pellets was similar to that obtained by Erlich and Fransson (Citation2011), Dudyński et al. (Citation2015), and Umeda et al. (Citation2019) at 300 °C. Furthermore, at ER 0.2, the average gas yield of MSW and wood pellets was similar to that reported by Lee et al. (Citation2020).
According to , the gas yield from gasification of MSW biomass increased from 1.91 Nm3/kg to 1.94 Nm3/kg before falling to 1.91 Nm3/kg at 900 °C, with an average of 1.92 Nm3/kg. Yield gas gasification with wood pellet biomass increased from 1.92 Nm3/kg to 2.16 Nm3/kg, with an average of 2.05 Nm3/kg. LHV of syngas was used for gasification with MSW feedstock and wood pellets as the temperature rose. Meanwhile, at 750–800 °C, gas yield producers have a maximum MSW for gasification. As the temperature increased, so did the producer’s yield gas for gasification with wood pellets. The average gas yield from biomass wood pellet gasification is higher than the average gas yield from MSW gasification. This gas yield is higher than that reported by Sharma et al. (Citation2020) at ER = 0.2, but lower when gasified at ER = 0.34. Thus, in addition to temperature, the ER factor influences the amount of gas yield. The influence of ER and temperature on characteristics on gas yield can be justified by Pio & Tarelho, Citation2020):
(23)
(23)
At constant ER, the gas yield increases as the temperature rises, according to EquationEq. (23)(23)
(23) . This is the case with biomass wood pellet gasification (). Such cases do not occur in MSW gasification. This is possible because of the particle size factor mentioned by Kumari and Mohanty (Citation2022).
When comparing the influence of heating with sunshine, the LHV of syngas with MSW biomass without pretreatment was 3.96–4.6 MJ/kg (Lee et al., Citation2020; Saleh et al., Citation2020), but the LHV in this study was between 5.32–6.18 MJ/Nm3 due to the impact of preheating with sunlight. Similarly, the LHV of syngas gasification from wood pellets biomass without torrefaction treatment obtained by Chen et al. (Citation2020) was 5.60 MJ/Nm3, which is lower than the 5.79 MJ/Nm3 obtained when using a torrefaction treatment, whereas the LHV obtained in this study ranged from 5.47 to 6.26 MJ/Nm3. The LHV of syngas increased as a result of the rise in gasifier temperature and the initial computation of the warming of potential solar radiation. Solar heating improved the LHV of syngas gasification relative to no preheating, which is consistent with the findings of prior research by Lee et al. (Citation2020) and Saleh et al. (Citation2020). Hence, the amount of LHV in syngas grew as a result of the increasing temperature of the gasifier and the early estimation of the warming of the sun’s influencing rays. Solar preheating of biomass has the potential to be used in Indonesia, particularly in the most distant, remote, and isolated regions of the gasification process. A possible increase in the LHV of syngas gasification may already be able to replace diesel fuel. Thus, we propose that this technique can be utilized to feed a dual-fuel diesel engine that utilizes syngas. The lower average gas production of MSW biomass gasification producers compared to wood pellets can be explained by the lower sodium content of wood pellets (39.97% vol) compared to MSW gasification gas (46.44% vol) on average (39.97% vol).
3.4. Effect of temperature on cold gas efficiency and carbon conversion
In addition to the LHV and gas yield, CGE and CCE are essential parameters for gasification. CGE refers to the first law of thermodynamics as the ratio of energy output to input energy. The CGE can be calculated from the ratio of the mass rate of the syngas product and the lower heating value of the gas to the feedstock’s mass rate and lower heating value, as shown in EquationEquation (5)(5)
(5) . Therefore, CGE is determined by the energy conversion performance during the gasification process. The CCE is also one of the measuring tools for the success of the mass conversion process in biomass gasification, which converts solid fuels into fuel-capable gases, as shown in EquationEquation (4)
(4)
(4) . The CCE measures and identifies the amount of unburned carbon and tar as part of the gasification product. It can be determined from the amount of carbon in the feedstock that can be converted into a gas scanned from carbon monoxide, carbon dioxide, and methane gas volume. According to the research results of Lee et al. (Citation2020), CGE is greater than 80% and CCE ranges from 60-84%. According to the research results of Atnaw et al. (Citation2013), when ER rises, CGE decreases and CCE rises. The following correlations show the effect of temperature and ER on CGE and CCE: Ariffin et al. (Citation2016), Pio and Tarelho (Citation2020), and Nemmour, Inayat, Janajreh, and Ghenai (Citation2022), each of them is:
(24)
(24)
(25)
(25)
(26)
(26)
(27)
(27)
shows the impact of gasifier reactor oxidation zone temperature on CGE and CCE with biomass from (a) MSW and (b) wood pellets at an ER of 0.22. shows that as the temperature increased, the CGE gasification for MSW biomass increased from 51.15% at 700 °C to 59.23% at 900 °C, yielding an average CGE of 56.60%. Meanwhile, the CCE dropped from 94.78% at 700 °C to 94.68% at 750 °C, then increased to 84.87% at 900 °C, with the average CCE remaining at 94.77%. shows that the CGE and CCE of wood pellets increased with increasing temperature, from 58.05% at 700 °C to 75.00% at 900 °C. Wood pellets, on the other hand, had an average CGE of 67.90%. At 900 °C, the CCE of wood pellet gasification decreased from 94.88% to 93.77%, while the average CCE was 94.27%. This demonstrates that as the temperature of the gasification process with MSW biomass increased, the amount of feedstock converted into combustion-capable gas increased. In contrast, when wood pellets were used, the amount of feedstock converted to gas decreased. Based on this phenomenon, whenever the gasification process uses wood pellets, the oxidation zone should be controlled to keep the temperature below 1000 °C.
Figure 8. The effect of reactor temperature on cold gas efficiency (CGE) and carbon conversion efficiency (CCE) with biomass from (a) municipal solid waste (MSW), (b) wood pellets at ER = 0.22.

This result with MSW biomass is confirmed by the results of Ariffin et al. (Citation2016) and Pio and Tarelho (Citation2020), which indicate that as the temperature rises, CCE and CGE similarly increase. The results of CCE gasification with biomass wood pellets differ from those presented by Pio and Tarelho (Citation2020), but CCE wood pellets can be justified by research results from (Nemmour et al., Citation2022). T = 838 °C, ER = 0.45 is the optimization point of EquationEq. (27)(27)
(27) . If the temperature rises above 838 °C at constant conditions ER = 0.22, the CCE tends to fall. The average CGE of MSW was 56.6%, and the CGE of wood pellets was 67.9%, yielding results that were similar to those of Atnaw et al. (Citation2013); Erlich and Fransson (Citation2011) at ER = 0.36. The CGE results of this study are also similar to those of Lee et al. (Citation2020), specifically at ER = 0.2. This study’s CGE will be higher than that of (Ding et al., Citation2018; Umeda et al., Citation2019), but lower than that of Aydin et al. (Citation2019); Calì et al. (Citation2020), where CGE reached 80%. When compared to published research results, the results of this study were relatively similar to the results of Atnaw et al. (Citation2013); Dudyński et al. (Citation2015) at ER = 0.36, where the carbon conversion was above 90.0%.
The pre-gasification heating of biomass with sunlight affects the gasification efficiency, specifically CGE and CCE. Compared to the results of this research, the CGE gasification of MSW without initial treatment performed significantly better than the CCE, and the CCE performed significantly better than the early treatment (Ependi et al., Citation2019; Saleh et al., Citation2020). It significantly increased CGE and decreased CCE compared to torrefaction pretreatment of MSW (Umeda et al., Citation2019). Compared to prior research by Chen et al. (Citation2020), the effect of preheating with sunshine on wood pellet gasification resulted in decreased CGE without initial behavior, but was greater than torrefaction as the initial treatment. However, the CCE in this investigation was greater than that of pellets treated with torrefaction prior to gasification and less than that of pellets gasified with tapa pretreatment. Consequently, even though the CCE was lower than that of untreated biomass, the CGE was higher after the initial heating with sun energy.
4. Conclusions
Preheating in the sun for four days can reduce the oxygen, hydrogen, moisture, and volatile matter content, increase the molar and fixed carbon contents, and raise the HHV value of the feedstock. The gasification process utilises a downdraft reactor with a biomass capacity of 135–144 kg and an air rate of 180–220 L/min at an equivalent ratio of 0.22 at temperatures ranging from 700 to 900 °C. The gasification process with MSW biomass yielded gas concentrations of 25.76% CO, 12.16% CO2, 3.66% CH4, and 11.98% H2 at a feedstock mass rate of 11.67 kg/h. Syngas had an LHV of 5.85 MJ/Nm3, a cold gas efficiency of 56.5%, and a carbon conversion efficiency of 94.77%. The gasification process using biomass wood pellets with a feedstock mass rate of 12.73 kg/h resulted in gas concentrations of 22.96% CO, 17.42% CO2, 3.74% CH4, and 15.91% H2. Syngas had an LHV of 5.95 MJ/Nm3, a cold gas efficiency of 67.90%, and a carbon conversion efficiency of 94.27%. According to the findings of this study, using a downdraft reactor for gasification is sufficient to convert biomass into syngas. The method of solar preheating has been shown to increase the calorific value of feedstock and gas yield. The calorific value of yield gas indicates that it could be used as fuel in dual-fuel diesel engines.
Nomenclature | ||
ER | = | equivalent ratio |
O/C | = | oxygen to carbon ratio |
H/C | = | hydrogen to carbon ratio |
LHV | = | lower heating value |
HHV | = | height heating value |
MSW | = | municipal solid waste |
CGE | = | cold gases efficiency |
CCE | = | carbon conversion efficiency |
CO | = | carbon monoxide |
CO2 | = | carbon dioxide |
CH4 | = | methane |
H2 | = | hydrogen |
Author contributions
All authors contributed substantially to the manuscript. Conceptualization, P.S. and H.H; methodology, P.S and R.N.; data curation, P.S, H.H., and M.R.; writing—original draft preparation, P.S. and H.H; writing—review and editing, P.S., R.N., and H.H.; software, P.S.; validation, P.S., H.H, and M.R.; formal analysis, P.S., R.N., and H.H.; supervision, R.N. and M.R. All authors have read and agreed to the published version of the manuscript.
Acknowledgments
We would like to express our gratitude to the Director General of Higher Education of the Ministry of Education and Culture, the LL3 Coordinator of the Ministry of Education and Culture, and Institut Teknologi PLN for the trust given so that this research was conducted properly.
Data availability statement
Data are not publicly available; the data may be made available upon request from the corresponding author.
Additional information
Funding
References
- Ariffin, M. A., Wan Mahmood, W. M. F., Mohamed, R., & Mohd Nor, M. T. (2016). Performance of oil palm kernel shell gasification using a medium-scale downdraft gasifier. International Journal of Green Energy, 13(5), 513–520. doi:10.1080/15435075.2014.966266
- Muhammad Afif Ariffin, Wan Mohd Faizal Wan Mahmood, Ramizi Mohamed, Mohd Tusirin Mohd Nor. (2017). Performance of oil palm frond gasification using medium-scale downdraft gasification for electricity generation. IET Renewable Power Generation, 9 (3), 228–235. doi:10.1049/iet-rpg.2014.0133
- Atnaw, S. M., Sulaiman, S. A., & Yusup, S. (2013). Syngas production from downdraft gasification of oil palm fronds. Energy, 61, 491–501. doi:10.1016/j.energy.2013.09.039
- Aydin, E. S., Yucel, O., & Sadikoglu, H. (2019). Experimental study on hydrogen-rich syngas production via gasification of pine cone particles and wood pellets in a fixed bed downdraft gasifier. International Journal of Hydrogen Energy, 44(32), 17389–17396. doi:10.1016/j.ijhydene.2019.02.175
- Basu, P. (2013). Biomass Gasification, Pyrolysis and Torrefaction: Practical Design and Theory. London: Academic Press. ISBN:978-0-12-396488-5.
- BPPT. (2021). Outlook Engergi Indonesia 2021, Perspektif Teknologi Energi Indonesia. PPIPE BPPT. https://www.bppt.go.id/dokumen/outlook-energi
- Buragohain, B., Mahanta, P., & Moholkar, V. S. (2012). Performance correlations for biomass gasifiers using semi-equilibirium non-stoichiometric thermodynamic models. International Journal of Energy Research, 36(5), 590–618. doi:10.1002/er
- Calì, G., Deiana, P., Bassano, C., Meloni, S., Maggio, E., Mascia, M., & Pettinau, A. (2020). Syngas production, clean-up and wastewater management in a demo-scale fixed-bed updraft biomass gasification unit. Energies, 13(10), 2594. doi:10.3390/en13102594
- Channiwala, S. A., & Parikh, P. P. (2002). A unified correlation for estimating HHV of solid, liquid and gaseous fuels. Fuel, 81(8), 1051–1063. doi:10.1016/S0016-2361(01)00131-4
- Chen, D., Gao, A., Cen, K., Zhang, J., Cao, X., & Ma, Z. (2018). Investigation of biomass torrefaction based on three major components: Hemicellulose, cellulose, and lignin. Energy Conversion and Management, 169(May), 228–237. doi:10.1016/j.enconman.2018.05.063
- Chen, G., Jamro, I. A., Samo, S. R., Wenga, T., Baloch, H. A., Yan, B., & Ma, W. (2020). Hydrogen-rich syngas production from municipal solid waste gasification through the application of central composite design: An optimization study. International Journal of Hydrogen Energy, 45(58), 33260–33273. doi:10.1016/j.ijhydene.2020.09.118
- Chen, W. H., Peng, J., & Bi, X. T. (2015). A state-of-the-art review of biomass torrefaction, densification and applications. Renewable and Sustainable Energy Reviews, 44, 847–866. doi:10.1016/j.rser.2014.12.039
- DEN. (2019). Outlook Energi Indonesia 2019. In S. Abdurrahman, M. Pertiwi, & Walujanto (Eds.), Sekretariat Jenderal Dewan Eenergi Nasional (DEN). DEN, Dewan Energi Nasional. https://www.esdm.go.id/assets/media/content/content-outlook-energi-indonesia-2019-bahasa-indonesia.pdf.
- Ding, L., Yoshikawa, K., Fukuhara, M., Kowata, Y., Nakamura, S., Xin, D., & Muhan, L. (2018). Development of an ultra-small biomass gasification and power generation system: Part 2. Gasification characteristics of carbonized pellets/briquettes in a pilot-scale updraft fixed bed gasifier. Fuel, 220(January), 210–219. doi:10.1016/j.fuel.2018.01.080
- Dudyński, M., Van Dyk, J. C., Kwiatkowski, K., & Sosnowska, M. (2015). Biomass gasification: Influence of torrefaction on syngas production and tar formation. Fuel Processing Technology, 131, 203–212. doi:10.1016/j.fuproc.2014.11.018
- Ependi, D. R., Saleh, A. R., & Sudarmanta, B. (2019). The experimental study of the effect of air preheating in MSW pellet multi-stage downdraft gasifier. IPTEK the Journal for Technology and Science, 30(2), 36–40. doi:10.12962/j20882033.v30i2.5005
- Erlich, C., & Fransson, T. H. (2011). Downdraft gasification of pellets made of wood, palm-oil residues respective bagasse: Experimental study. Applied Energy, 88(3), 899–908. doi:10.1016/j.apenergy.2010.08.028
- Heidenreich, S., & Foscolo, P. U. (2015). New concepts in biomass gasification. Progress in Energy and Combustion Science, 46, 72–95. doi:10.1016/j.pecs.2014.06.002
- Homdoung, N., Dussadee, N., Sasujit, K., Kiatsiriroat, T., & Tippayawong, N. (2019). Performance investigation of a gasifier and gas engine system operated on municipal solid waste briquettes. International Journal of Renewable Energy Development, 8(2), 179–184. doi:10.14710/ijred.8.2.179-184
- Hu, J., Li, C., Lee, D. J., Guo, Q., Zhao, S., Zhang, Q., & Li, D. (2019). Syngas production from biomass using Fe-based oxygen carrier: Optimization. Bioresource Technology, 280, 183–187. doi:10.1016/j.biortech.2019.02.012
- Indrawan, N., Thapa, S., Bhoi, P. R., Huhnke, R. L., & Kumar, A. (2017). Engine power generation and emission performance of syngas generated from low-density biomass. Energy Conversion and Management, 148, 593–603. doi:10.1016/j.enconman.2017.05.066
- Kumari, P., & Mohanty, B. (2022). Maximization of hydrogen production from pine needles steam gasification based on response surface methodology. Biomass Conversion and Biorefinery, 12(7), 2335–2348. doi:10.1007/s13399-020-00761-7
- Kurkela, E., Kurkela, M., & Ilkka, H. (2014). Effect of wood particle size and different process variables on the performance of steam-oxygen blown circulating fluidized bed gasifier. Environmental Progress & Sustainable Energy, 33(3), 681–687. doi:10.1002/ep
- Lee, S. Y., Alam, M. T., Han, G. H., Choi, D. H., & Park, S. W. (2020). Gasification applicability of Korean municipal waste derived solid fuel: A comparative study. Processes, 8(11), 1375. doi:10.3390/pr8111375
- Molino, A., Chianese, S., & Musmarra, D. (2016). Biomass gasification technology: The state of the art overview. Journal of Energy Chemistry, 25(1), 10–25. doi:10.1016/j.jechem.2015.11.005
- Molino, A., Larocca, V., Chianese, S., & Musmarra, D. (2018). Biofuels production by biomass gasification: A review. Energies, 11(4), 811. doi:10.3390/en11040811
- Nemmour, A., Inayat, A., Janajreh, I., & Ghenai, C. (2022). New performance correlations of municipal solid waste gasification for sustainable syngas fuel production. Biomass Conversion and Biorefinery, 12(10), 4271–4289. doi:10.1007/s13399-021-02237-8
- Nimmanterdwong, P., Chalermsinsuwan, B., & Piumsomboon, P. (2020). Simplified empirical model to predict biomass thermal conversion products. Energy Reports, 6(April), 286–292. doi:10.1016/j.egyr.2020.08.051
- Noma, T., Ide, K., Yoshikawa, J., Kojo, K., Matsui, H., Nakajima, R., & Imai, K. (2012). Development of waste gasification and gas reforming system for municipal solid waste (MSW). Journal of Material Cycles and Waste Management, 14(3), 153–161. doi:10.1007/s10163-012-0051-3
- Nyakuma, B. B., Wong, S. L., Oladokun, O., Bello, A. A., Hambali, H. U., Abdullah, T. A. T., & Wong, K. Y. (2023). Review of the fuel properties, characterisation techniques, and pre-treatment technologies for oil palm empty fruit bunches. Biomass Conversion and Biorefinery, 13(1), 471–497. doi:10.1007/s13399-020-01133-x
- Patra, T. K., & Sheth, P. N. (2015). Biomass gasification models for downdraft gasifier: A state-of-the-art review. Renewable and Sustainable Energy Reviews, 50, 583–593. doi:10.1016/j.rser.2015.05.012
- Pio, D. T., & Tarelho, L. A. C. (2020). Empirical and chemical equilibrium modelling for prediction of biomass gasification products in bubbling fluidized beds. Energy, 202, 117654. doi:10.1016/j.energy.2020.117654
- Primadita, D. S., Kumara, I. N. S., & Ariastina, W. (2020). A review on biomass for electricity generation in Indonesia. Journal of Electrical, Electronics and Informatics, 4(1), 1–9. doi:10.24843/JEEI.2020.v04.i01.p01
- Puig-Arnavat, M., Bruno, J. C., & Coronas, A. (2010). Review and analysis of biomass gasification models. Renewable and Sustainable Energy Reviews, 14(9), 2841–2851. doi:10.1016/j.rser.2010.07.030
- Rahman, M. A., Parvej, A. M., & Aziz, M. A. (2021). Concentrating technologies with reactor integration and effect of process variables on solar assisted pyrolysis: A critical review. Thermal Science and Engineering Progress, 25(April), 100957. doi:10.1016/j.tsep.2021.100957
- Rajendran, N., Gurunathan, B., Han, J., Krishna, S., Ananth, A., Venugopal, K., & Sherly Priyanka, R. B. (2021). Recent advances in valorization of organic municipal waste into energy using biorefinery approach, environment and economic analysis. Bioresource Technology, 337(May), 125498. doi:10.1016/j.biortech.2021.125498
- Ruiz, J. A., Juárez, M. C., Morales, M. P., Muñoz, P., & Mendívil, M. A. (2013). Biomass gasification for electricity generation: Review of current technology barriers. Renewable and Sustainable Energy Reviews, 18, 174–183. doi:10.1016/j.rser.2012.10.021
- Rupesh, S., Muraleedharan, C., & Arun, P. (2015). A comparative study on gaseous fuel generation capability of biomass materials by thermo-chemical gasification using stoichiometric quasi-steady-state model. International Journal of Energy and Environmental Engineering, 6(4), 375–384. doi:10.1007/s40095-015-0182-0
- Rupesh, S., Muraleedharan, C., & Arun, P. (2020). Energy and exergy analysis of syngas production from different biomasses through air-steam gasification. Frontiers in Energy, 14(3), 607–619. doi:10.1007/s11708-016-0439-1
- Saleh, A. R., Sudarmanta, B., Fansuri, H., & Muraza, O. (2019). Improved municipal solid waste gasification efficiency using a modified downdraft gasifier with variations of air input and preheated air temperature. Energy & Fuels, 33(11), 11049–11056. doi:10.1021/acs.energyfuels.9b02486
- Saleh, A. R., Sudarmanta, B., Fansuri, H., & Muraza, O. (2020). Syngas production from municipal solid waste with a reduced tar yield by three-stages of air inlet to a downdraft gasifier. Fuel, 263(September), 116509. doi:10.1016/j.fuel.2019.116509
- Sharma, M., & Kaushal, R. (2020). Performance and emission analysis of a dual fuel variable compression ratio (VCR) CI engine utilizing producer gas derived from walnut shells. Energy, 192, 116725. doi:10.1016/j.energy.2019.116725
- Sharma, P. K., Sharma, A. K., Pulla, R. H., & Sahoo, P. K. (2020). Performance analysis of a medium-scale downdraft gasifier using Lantana camera biomass as feeding material. Energy Sources, Part A: Recovery, Utilization and Environmental Effects, . doi:10.1080/15567036.2020.1809565
- Sidek, F. N., Abdul Samad, N. A. F., & Saleh, S. (2020). Review on effects of gasifying agents, temperature and equivalence ratio in biomass gasification process. IOP Conference Series: Materials Science and Engineering, 863(1), 012028. doi:10.1088/1757-899X/863/1/012028
- Situmorang, Y. A., Zhao, Z., Yoshida, A., Abudula, A., & Guan, G. (2020). Small-scale biomass gasification systems for power generation (<200 kW class): A review. Renewable and Sustainable Energy Reviews, 117(January), 109486. doi:10.1016/j.rser.2019.109486
- Subramanian, P., Sampathrajan, A., & Venkatachalam, P. (2011). Fluidized bed gasification of select granular biomaterials. Bioresource Technology, 102(2), 1914–1920. doi:10.1016/j.biortech.2010.08.022
- Sudarmanta, B. (2010). Dual fuel engine performance using biodiesel and syngas from rice husk downdraft gasifier for power generation. In International Seminar on Sustainable Biomass Production and Utilization: Challenges and Opportunities, pp. 1–8.
- Sudarmanta, B., Sampurno, Dwiyantoro, B. A., Gemilang, S. E., & Putra, A. B. K. (2019). Performance characterization of waste to electric prototype uses a dual fuel diesel engine and a multi-stage downdraft gasification reactor. Materials Science Forum, 964, 80–87. doi:10.4028/www.scientific.net/MSF.964.80
- Susastriawan, A. A. P., Purwanto, Y., & Purnomo. (2021). Biomass gasifier–internal combustion engine system: Review of literature. International Journal of Sustainable Engineering, 14(5), 1090–1100. doi:10.1080/19397038.2020.1821404
- Susastriawan, A. A. P., Saptoadi, H., Purnomo. (2017). Small-scale downdraft gasifiers for biomass gasification: A review. Renewable and Sustainable Energy Reviews, 76(February), 989–1003. doi:10.1016/j.rser.2017.03.112
- Trinh, T. H., & Uemura, Y. (2019). A theoretical equation presenting slope in van Krevelen diagram for biomass pyrolysis. Platform - A Journal of Engineering, 3(April), 56–64.
- Umar, H. A., Sulaiman, S. A., Said, M. A., Gungor, A., Ahmad, R. K., & Inayat, M. (2021). Syngas production from gasification and co-gasification of oil palm trunk and frond using a down-draft gasifier. International Journal of Energy Research, 45(5), 8103–8115. doi:10.1002/er.6345
- Umeda, K., Nakamura, S., Lu, D., & Yoshikawa, K. (2019). Biomass gasification employing low-temperature carbonization pretreatment for tar reduction. Biomass and Bioenergy, 126(April), 142–149. doi:10.1016/j.biombioe.2019.05.002