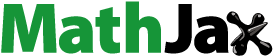
Abstract
A set of mono-metallic nanoparticles catalysts, including palladium, platinum, and gold supported on titania, were prepared via the sol-immobilization technique, and evaluated for the total oxidation of propane as a model reaction of volatile organic compounds (VOCs) oxidation, which is a wide-ranging group of organic pollutants that contribute to serious atmospheric problems. The results showed that 1 wt.% Pt/TiO2 was the most active catalyst toward CO2, as the catalyst was very active, and the complete conversion of propane was achieved with full selectivity toward CO2. The effect of the support type was investigated through the immobilization of platinum nanoparticles on CeO2 and γ-Al2O3. The results indicated that the catalytic activity follows the order 1% Pt/TiO2 > 1% Pt/CeO2 > 1% Pt/Al2O3. For the Pt/TiO2 catalyst, the influence of the calcination temperature and metal loading on the catalytic activity was investigated. There is a slight increase in the Pt particle size when raising the calcination temperature from 300 °C to 500 °C, which enhances the catalytic activity of 1% Pt/TiO2 at 500 °C. Furthermore, increasing the metal loading from 0.1 to 1 wt.% enhances the catalytic activity as a result of the increase in particle size. Different characterization techniques were utilized, including XRD, TEM, XPS, and MP-AES, to determine the structure-activity relationship, and together they indicate that the catalytic activity is influenced more by the particle size of Pt nanoparticles than by the oxidation state.
1. Introduction
Improving air quality is now a central theme in many high-level government strategies, as international organizations are imposing increasingly stringent targets to reduce the emissions of atmospheric pollutants. One class of atmospheric pollutants that is receiving attention is that of volatile organic compounds (VOCs), which is a wide-ranging group of organic pollutants that contribute to serious atmospheric problems. VOCs are commonly powerful greenhouse gases, which are directly involved in atmospheric chemical processes leading to the production of photochemical smog and ground-level ozone (Duan, Tan, Yang, Wu, & Hao, Citation2008; Ryerson et al., Citation2001). VOCs can also be produced through agglomeration and condensation from soot (Flagan & Seinfield, Citation1988; Gurjar et al., Citation2010). Short-chain alkanes, such as propane, are considered to be the most stable VOCs and can remain for a significant period of time in the atmosphere (Choudhary et al., Citation2002). Although the anthropogenic emission of carbon dioxide has received the most attention in the context of greenhouse gas production, the global warming potential of short-chain alkanes is greater than that of carbon dioxide (Neyertz, Volpe, & Gigola, Citation2004). The emission of propane into the atmosphere is currently increasing, as the use of liquefied petroleum gas (LPG) (which consists of propane and butane) as a fuel is on the rise (Ramadhas, Citation2011).
Currently, the emission of VOCs can be controlled by various technologies such as adsorption, thermal oxidation, catalytic oxidation, absorption, and condensation. It is more cost effective to use catalytic oxidation as an abatement technology than thermal combustion, because oxidation can operate at lower temperatures and, thus, requires less fuel for the removal of VOCs. This technique is considered as a highly effective pollution removal technique, as it completely degrades VOCs into carbon dioxide and water, and substantially reduces VOC levels from polluted air streams. Additionally, catalytic oxidation is capable of treating a wide range of concentrations of VOCs, including very dilute streams (He et al., Citation2019; Huang, Xu, Feng, & Leung, Citation2015).
Propane is an excellent model of VOCs for the assay of the efficacy of catalytic total oxidation, in that propane is a common VOC that has a significant atmospheric impact. However, the catalytic total oxidation of short-chain alkanes is challenging and requires the design of an effective catalyst (Choudhary et al., Citation2002). Noble metal-based catalysts have been the most widely studied for the combustion of alkanes, because they demonstrate a high intrinsic total oxidation activity (Chen, Cao, Ran, Wu, & Weng, Citation2018; Debecker, Farin, Gaigneaux, Sanchez, & Sassoye, Citation2014; Hu et al., Citation2018; Khudorozhkov, Chetyrin, Bukhtiyarov, Prosvirin, & Bukhtiyarov, Citation2017; Okal & Zawadzki, Citation2009; Park, Kim, & Park, Citation2015). A wide range of techniques have been used to prepare supported noble-metal nanoparticles, including wet impregnation and incipient wetness impregnation (Avila, Vignatti, Apesteguía, & Garetto, Citation2014; Debecker et al., Citation2014; Khudorozhkov et al., Citation2017; Park et al., Citation2011; Schick et al., Citation2019; Taylor et al., Citation2012). summarizes different catalytic systems that have been reported in the literature for the total oxidation of propane over supported noble-metal catalysts prepared through conventional methods (Avila et al., Citation2014; Chen et al., Citation2018; Garcia et al., Citation2015; Park et al., Citation2013; Citation2015; Ruth et al., Citation2000; Viéitez-Calo, Morgan, Golunski, Taylor, & Twigg, Citation2021; Yang et al., Citation2021).
Table 1. Some catalytic systems reported in the literature for total oxidation of propane.
Recently, Viéitez-Calo et al. (Citation2021) reported the hydration effects of a series of 1%Pt/TiO2–SiO2 catalysts prepared via impregnation method on the oxidation of propane and NO. The results revealed that the activities of the catalysts were affected by the composition of the support material. The existence of residual chloride in the support showed lower activity of the Pt catalysts than the S-containing support. However, the removal of the residual chloride can be achieved by the hydrothermal treatment, yet it also leaves the catalyst in a hydrated form.
Other precious metals were successfully utilized for the catalytic oxidation of propane. The catalytic performance of a series of mixed oxide Ru and Ir catalysts Ru were evaluated for the total oxidation of propane. The catalysts were supported on CeO2, γ-Al2O3 or ZrO2. The results indicated that the pure Ir catalyst showed lower catalytic performance than the mix Ir-Ru oxide catalyst (RuxIr1-xO2 with x < 0.25). Furthermore, the catalytic performance of Ru-Ir on ZnO2 was higher than RuO2 at high conversions, suggesting the synergistic effect for Ir points in the mixed catalyst (Wang et al., Citation2022).
A study conducted by Camposeco et al. (Camposeco, Castillo, & Zanella, Citation2023) demonstrates the catalytic oxidation of propane by Pd nanoparticles on MnOx/TiO2 using the sol-gel method. The results indicate that the interaction between Pd and MnOx/TiO2 is strong, and the TiO2 support temperature affects the catalyst’s properties. Catalysts with 1 and 2 wt% Pd loading showed high activity and stability, and the addition of Pd nanoparticles reduced the oxidation temperature.
Pt/TixAlOy catalysts were prepared using a one-step evaporation-induced self-assembly EISA technique and investigated for catalytic oxidation of propane. The catalysts showed high specific surface area and increased acidic sites with TiO2 content. However, too high acidic sites could lead to coke deposition and pore clogging. The Pt/Ti0.1AlOy catalyst showed superior catalytic activity and superior sulfur resistance (Liu, Han, Dong, Feng, & Zhicheng, Citation2022).
The sol-immobilization technique is one of the promising for the preparation of supported metal nanoparticles. In this technique, the development of the nanoparticles is controlled by the utilization of a stabilizing agent such as polyvinylpyrrolidone (PVP) or polyvinyl alcohol (PVA), resulting in colloidal metal nanoparticles with a well-defined particle size. The metal is then reduced through the utilization of a reagent such as NaBH4 and the colloids are produced. Subsequently, the pH of the solution is adjusted to low values, and the support material is added to ensure that the metal is completely immobilized. Various factors can affect the immobilization step, including the identity of the stabilizer, the isoelectric point, surface functionality, and surface area of the support.
The advantage of this technique is the ability to tune the particle size during the synthesis of metal nanoparticles which can not be achieved by other conventional catalysts preparation techniques such as incipient wetness impregnation (Bahruji et al., Citation2016; Shil & Das, Citation2013; Villa et al., Citation2010).
To the best of our knowledge, there is no study in the literature that deal with the effect of supported metal nanoparticle catalysts prepared using the sol-immobilization technique on the total oxidation of propane. In this work, we synthesize a set of mono-metallic nanoparticles via the sol-immobilization technique and evaluate the catalytic activity of the prepared catalysts for the total oxidation of propane as a surrogate for the oxidation of VOCs in the air. The effect of support material, thermal treatment of the catalyst and metal loading were evaluated for the propane oxidation reaction. Furthermore, the stability of the catalyst was evaluated to provide full image about the catalyst performance.
2. Experimental
2.1. Materials
The reagents used were a hexachloroplatinic acid solution H2PtCl6 (99.99%, Alfa Aesar), potassium tetrachloropalladate K2 PdCl4 (II), (99.99%, Alfa Aesar), Sodiumtetrachloroaurate(III) dihydrate NaAuCl4·2H2O (99.99%, Alfa Aesar), polyvinyl alcohol (99%, Sigma-Aldrich), and sodium borohydride (98%, Sigma-Aldrich). Titanium dioxide (Degussa P25, 50 m2/g), γ-Al2O3 (Cyanamid-Ketjen CK300, 180 m2/g), and CeO2 (Rhodia HSA5, 240 m2/g) were employed as supports.
2.2. Catalyst preparation
The sol-immobilization technique was used to prepare all catalysts. The desired amount of the precursor [(H2PtCl6, 0.0184 mol), (K2PdCl4, 0.0183 mol) or (NaAuCl4·2H2O, 0.0183 mol) or was dissolved in water (800 mL g−1 of catalyst prepared). After stirring the solution for five minutes, polyvinyl alcohol (molecular weight MW ¼ 9000–10,000 g mol−1) was added (metal-to-PVA weight ratio = 0.65). After five minutes of stirring, NaBH4 was added to the solution (NaBH4-to-metal molar ratio = 5) and the sol was generated under continuous stirring. After 30 min of stirring, the pH of the solution was decrease to 2 by adding 0.1 M sulfuric acid. Subsequently, commercial titania support P25 (0.99 g) (or any of the other supports mentioned) was added to the colloidal solution under continuous stirring at 80 °C until the formation of a thick paste. After filtering of the resultant slurry, the catalyst was thoroughly washed several times with distilled water before drying at 110 °C for 16 h. The catalysts were then calcined at 500 °C in static air for three hours.
2.3. Catalyst characterizations
To obtain powder X-ray diffraction (XRD) patterns, a PANalytical X'pert Pro diffractometer was employed. This diffractometer was equipped with a Ni-filtered CuKα radiation source operating at 40 milliamperes and 40 kilovolts. Spanning the 2θ range 10–80°, diffraction patterns were denoted, and the phases were identified by comparison to entries in the International Center for Diffraction Data (ICDD) database. To obtain X-ray photoelectron spectra (XPS), a Kratos Axis Ultra DLD spectrometer was employed. This spectrometer utilized a monochromatic Al Kα X-ray source operating at 100 Watts. Analyzer pass energies of either 40 eV or 160 eV were used to record spectra for survey scans and detailed scans, respectively. Utilizing the C(1s) binding energy of adventitious carbon contamination (284.7 eV), it was possible to reference the binding energies of the resulting spectra via quantification by the CasaXPS™ software (v. 2.3.15). Manufacturer-supplied sensitivity factors were used in this process. To obtain transmission electron microscopy (TEM) imaging, a JEOL JEM-2100 microscope was employed. This microscope was fitted with a LaB6 filament operating at 200 kilovolts. Catalyst samples were powdered and dispersed in the dry state onto lacey carbon-coated 400 mesh copper grids, after which mean particle size distributions could be obtained using Image J software based on analyses of 300 particles. Microwave plasma-atomic emission spectroscopy (MP-AES) was used to confirm metal loading. An Agilent 4100 spectrometer was employed for this purpose using a fixed quantity of the catalyst for each sample. The catalyst was first digested for 16 h in 50 mL of 10% aqua regia solution. Subsequently, the processed solution was passed through PTFE filters (Acrodisc PVDF 0.45 μm) to remove any residual support material. For the spectrometric readings, the prepared catalyst samples were injected into the single-pass spray chamber of the spectrometer at a pressure of 120 kPa. This was done using a stream of nitrogen plasma in the absence of air. To calibrate the spectrometer, reference metal solutions were prepared from the manufacturer-supplied 1000 ppm solution and diluted to 2.5, 5, and 10 ppm. All sample measurements were performed in triplicate so that concentrations could be calculated from the averages.
2.4. Catalyst activity evaluation
To measure the catalytic activity of the samples, a fixed-bed laboratory micro-reactor was employed. Stainless steel reactor tubes (1/4 in. o.d.) were loaded with 50 mg of powdered catalyst and fed 5000 volumetric ppm propane in air at a total flow rate of 50 mL min−1. A gas hourly space velocity (GHSV) of 45,000 h−1 was achieved by packing the catalysts to a constant volume. Online gas chromatography over a 100–400 °C temperature range was used to analyze the reactants and products via thermal conductivity in a flame ionization detector. Reaction temperature was monitored with a thermocouple placed in the center of the catalyst bed and kept consistent with that of the external furnace to ensure that there was no significant detectable exotherm within the bed of the catalyst. At each temperature, three analyses were made for averaging, with the collection of data performed once steady-state activity was attained. Blank experiments conducted in an empty reactor reported negligible activity over the temperature range tested herein, as a control.
The GC results confirmed that we have only CO2 as a products. The following formula was used to calculate the percent propane conversion from the differences in concentrations between the inlet and outlet at each temperature setting:
Carbon balances and selectivity were assessed to be 100 ± 5% for each trial. The selectivity was calculated using the formula:
Arrhenius plots were used to extrapolate the activation energy for all catalysts. Here, values of ln(reaction rate) as a function of the reciprocal of absolute temperature (1/T) were plotted, and the activation energy was estimated from the slope of the resulting linear regression (Park et al., Citation2015). All the calculations of reaction rates and activation energies were performed at temperatures low enough (250 °C) that low conversion values were expected.
3. Results and discussion
3.1. Screening of catalysts
The catalytic oxidation of propane as a model reaction was carried out using a range of catalysts under the same reaction conditions. It is significant that only carbon dioxide (CO2) and water (H2O), were created, with no additional byproducts, proving that this catalytic process constituted a total oxidation reaction.
In the context of total oxidation of propane, the primary objective is to attain complete conversion of propane to (CO2) and (H2O), necessitating the utilization of an appropriate catalyst. Furthermore, the reaction system is enclosed to ensure the provision of an optimal environment for this transformation. It is important to note that interrupting the reaction to recover unintended byproducts would compromise the accuracy and reliability of the obtained results.
To determine the most effective catalyst for propane oxidation, several catalyst screening experiments were carried out. A set of mono-metallic nanoparticle catalysts supported on titania with similar nominal metal loadings of 1% were prepared via the sol-immobilization technique. shows the catalytic performance of 1% wt. of Pt/TiO2, Pd/TiO2, Au/TiO2, and the bare titania support (Degussa P25) for the total oxidation of propane. The data indicate that the TiO2 support exhibited poor catalytic activity for the total oxidation of propane below 350 °C. The value of T10 (the temperature at which 10% of propane was converted) was 396 °C, while 50% of propane conversion (T50) was not achieved until 435 °C. The catalytic performance was enhanced significantly when different precious-metal nanoparticles were loaded onto titania. Importantly, the selectivity toward CO2 was greater than 99% for all the evaluated catalysts. As shown in , the activity of the catalysts for propane combustion increased in the order:
Figure 1. Catalytic activity for total oxidation of propane over 1 wt.% Au, Pd, and Pt titania-supported catalysts prepared by sol immobilization. The feed gas is 5000 vppm propane, and GHSV = 50,000 mL h−1 gcat−1.

The 1% Pt/TiO2 exhibited the greatest catalytic performance, as the conversion started at 200 °C, 50% of the oxidation was observed at only 254 °C, and total oxidation of propane to CO2 was maintained at 350 °C. Lower catalytic activity was observed for 1 wt.% Pd/TiO2. Here, T50 was 327 °C, and the propane was not fully converted to CO2 until 500 °C. Gold nanoparticles proved to be the least active catalysts; the T50 for 1% Au/TiO2 was determined to be 402 °C. These results are in agreement with the study conducted by Schick et al. (Citation2019), who found that the catalytic activity of 2 wt.% Pt supported on silica (prepared by incipient wetness impregnation) was greater than that of 2 wt.% Pd/SiO2, while the 2% Au/SiO2 had the lowest catalytic performance.
The reaction rates and activation energies were calculated for these mono-metallic supported catalysts (). It can be noted that the reaction rate of the Pt catalyst is substantially greater than that of the other catalysts: by a factor of five over Pd and of nine over Au. The measured apparent activation energy of the Pt catalyst was significantly lower than that of Pd and Au.
Table 2. Catalytic activity and kinetic data of Pt, Pd, and Au nanoparticles supported on titania for the total oxidation of propane.
3.2. Effect of support type
As the supported Pt-nanoparticle catalyst prepared by sol immobilization was the most active compared to the Pd and Au nanoparticles, it was selected for further study. To evaluate the effect of support type on the catalytic activity of Pt nanoparticles and to determine the best support type for this type of study, different support materials were investigated for the total oxidation of propane. The Pt nanoparticles were prepared using the sol-immobilization technique and, additionally, were immobilized on CeO2 and γ-Al2O3 supports. The resulting catalysts were denoted as 1% Pt/CeO2 and 1% Pt/Al2O3, respectively. The catalytic performance of these two catalysts was studied in terms of the total oxidation of propane under the same conditions described above for the screening of the catalysts. The comparison of the three catalysts is shown in and . The results indicated that all the catalysts were active toward the complete oxidation of propane. However, the type of support substantially influences the catalytic performance of Pt nanoparticles. The catalytic performance of the catalysts follows this order:
Figure 2. Effect of support type on the catalytic activity of 1% wt. Pt nanoparticles for the total oxidation of propane. The feed gas is 5000 vppm propane, and GHSV = 50,000 mL h−1 gcat−1.

Table 3. Catalytic activity and kinetic data of Pt nanoparticles supported on different types of supports for the total oxidation of propane.
The values of T10 for the 1% Pt/TiO2 and 1% Pt/CeO2 were 209 °C and 210 °C, respectively, indicating that the initial catalytic efficacies of both catalysts were similar. However, the 1% Pt/Al2O3 had lower initial catalytic performance, as the T10 value was higher (257 °C). This ordinal ranking of catalytic activity extended to higher temperatures, as the values of T50 were 247 °C, 257 °C, and 332 °C for 1% Pt/TiO2, 1% Pt/CeO2, and 1% Pt/Al2O3, respectively. These results are consistent with those reported previously by Avila et al. (Citation2014), in that those authors observed the same trend for the catalytic conversion of propane over Pt nanoparticles (prepared by incipient wetness impregnation) and supported on TiO2, CeO2, and Al2O3. It was suggested that the elevated catalytic activity of the Pt/TiO2 can be related to the high acidity of the TiO2 support compared with the CeO2 and Al2O3, as the propane is mainly adsorbed onto the surface Lewis sites of the catalyst (Hasan, Zaki, & Pasupulety, Citation2002).
The estimated activation energies and reaction rates of the catalysts, shown in , indicate that the results are consistent with the catalytic behavior of these nanoparticles, as the value of the activation energy of the 1% Pt/TiO2 was the lowest of the three tested. The activation energy of the 1% Pt/CeO2 was slightly greater than that of the 1% Pt/TiO2. Avila et al. (Citation2014) suggested that the higher reaction rate of the 1% Pt/TiO2 can be attributed to the high uptake of propane over the TiO2 support compared to the CeO2 and Al2O3 supports, a situation that promotes the oxidation of propane over the TiO2 support.
The powder XRD patterns for catalysts on various support types are shown in . No diffraction from the Pt phase was observed, only diffraction peaks related to the supports. These data indicate that the Pt metal is efficiently dispersed on all the support types assayed (Albilali, Douthwaite, He, & Taylor, Citation2018; Baranowska & Okal, Citation2016).
3.3. Effect of thermal treatment
Based on our results of the catalyst screening and the effect of the support, Pt nanoparticles supported on titania were nominated as the best catalysts to conduct further investigation on the influence of different parameters, such as thermal treatment and metal loading of the Pt/TiO2 catalyst. Furthermore, we endeavored to shed light on the structure–activity relationship using XPS data and TEM images. The catalytic performance of thermally treated 1% Pt/TiO2 was studied for the total oxidation of propane with the aim of investigating the possible impact of particle size and oxidation state on catalyst performance. The 1% Pt/TiO2 was prepared via sol immobilization and calcined at three temperatures (300 °C, 400 °C, and 500 °C) in static air for two hours. The resulting samples were denoted as 1% Pt/TiO2(300), 1% Pt/TiO2(400), and 1%Pt/TiO2(500). The catalytic evaluation of these samples is presented in and . As shown in , the total oxidation activity of the catalysts for propane increased according to the following order:
Figure 4. Effect of thermal treatment on the catalytic activity of the 1 wt.% Pt%/TiO2 catalysts for the total oxidation of propane. The feed gas is 5000 vppm propane, and GHSV = 50,000 mL h−1 gcat−1.

Table 4. Catalytic activity and kinetic data of thermally treated 1% Pd/TiO2 for the total oxidation of propane.
It is evident that raising the calcination temperature of the 1%Pt/TiO2 catalyst has a marked influence on catalytic behavior. The value of T50 decreased by 15% (302 °C to 256 °C) when the calcination temperature was elevated from 300 °C to 500 °C ().
The reaction rates and activation energies associated with the results shown in are also presented in . The data show that the reaction rate increased by 111% when the calcination temperature for the 1% Pt/TiO2 catalyst increased from 300 °C to 500 °C. At the same time, there was a decrease in the activation energy from 43 kJ mol−1 to 21 kJ mol−1 when the calcination temperature was boosted from 300 °C to 500 °C.
For the purpose of elucidating the surface composition and electronic state, XPS analyses were carried out for the 1% Pt/TiO2 samples calcined at the same three temperatures (300 °C, 400 °C, and 500 °C), and the results are shown in and . For all catalysts, the doublet of Pt 4f7/2 and Pt 4f5/2 was located at binding energies (B.E.) of 70.7 and 74.0 eV, respectively, and can be assigned to metallic Pt0 supported on the titanium oxides; the typical binding energies of metallic Pt are found at 71.2 and 74.5 eV for the Pt 4f7/2 and Pt 4f5/2, respectively (Camposeco et al., Citation2023; Wang et al., Citation2022). The slight decrease in the binding energies of Pt 4f7/4 and Pt 4f5/4 can be attributed to a potent interaction between the Pt nanoparticles and the titania support (Zhu, Cheng, Yu, & Ho, Citation2016). It should be noted that the catalysts treated at 300 °C contain only surface metallic Pt0. In contrast, for the samples treated at higher temperatures (400 °C and 500 °C), the deconvolution of the peaks showed additional signals at higher B.E.s of 71.6 and 74.9 eV, which can be assigned to Pt2+ existing in the form of PtO or Pt(OH)2 (Cavaliere, Subianto, Chevallier, Jones, & Rozière, Citation2011). The ratio between metallic Pt to Pt2+ decreased with an increase in the calcination temperature (), confirming that, for calcination at temperatures above 300 °C, some of the surface metallic Pt was oxidized.
Figure 5. XPS for the Pt 4f region of the 1 wt.% Pt/TiO2 catalysts calcined at various temperatures.

Table 5. XPS data for thermally treated 1% Pd/TiO2.
TEM was subsequently utilized to investigate the impact of increasing the calcination temperature on the particle size of Pt in the Pt/TiO2 catalysts. shows the TEM images and the resulting particle size distributions of the thermally treated 1% Pt/TiO2. The images reflect the uniform dispersion of the platinum particles on the titania support for all catalysts. Furthermore, no considerable change in the mean particle size of the platinum particles was observed when the calcination temperature of the catalyst was elevated from 300 °C to 400 °C; the measured particle size was 1.40 ± 0.3 nm and 1.41 ± 0.3 nm, respectively. However, raising the calcination temperature to 500 °C influenced the particle size significantly. At that temperature, the mean particle size for the 1% Pt/TiO2(500) was 1.63 ± 0.3 nm, which is a 16.4% increase in the mean particle size as compared to the 1% Pt/TiO2(300) catalyst. This increase can be attributed to the sintering effect of Pt nanoparticles at high temperatures. Otto, Andino, and Parks (Citation1991) proposed that larger crystallites are more likely to contain favorable ensembles of active Pt sites, and that these, in turn, could accelerate the oxidation of propane.
Figure 6. Transmission electron microscopy images and the corresponding particle size distribution for the calcined 1 wt.% Pt/TiO2 catalysts prepared using sol immobilization at different calcination temperatures: (a) 300 °C, (b) 400 °C, and (c) 500 °C.

Interestingly, the particle size effect has a greater influence on the catalytic activity of the Pt nanoparticles than on the surface oxidation state. This result can be observed by comparing these parameters for the 1% Pt/TiO2(300) and 1% Pt/TiO2(400) (). It can be seen that the two samples have almost similar particle sizes, 1.40 ± 0.3 nm and 1.41 ± 0.3 nm, respectively, and similar catalytic performance despite the variation in the surface Pt0/Pt+2 content in the samples.
3.4. Effect of metal loading
The effect of platinum loading was investigated by reducing the platinum loading on the titania support down to 0.5 and 0.1 wt.%. The catalysts were designated as 0.5% Pt/TiO2 and 0.1% Pt/TiO2, respectively. MP-AES confirmed the existence of an appropriate Pt loading in each catalyst, as this was measured to be 0.51% and 0.12%, respectively. The catalytic activities of the reduced loading catalysts were evaluated for the total oxidation of propane under the same conditions used previously. The results are presented in and . Reducing the Pt loading had a noticeable impact on the catalytic activity because the catalytic activity decreased in the following order:
Figure 7. Effect of Pt loading on the catalytic performance of Pt/TiO2 catalysts for the total oxidation of propane. The feed gas is 5000 vppm propane, and GHSV = 50,000 mL h−1 gcat−1.

Table 6. Catalytic activity and kinetic data of Pt/TiO2 at different metal loading conditions for the total oxidation of propane.
A 99% selectivity toward CO2 was measured for all the evaluated catalysts, indicating that a reduction in the metal loading did not affect the selectivity toward CO2. The value of T10 was increased when the Pt loading decreased to 0.5 and 0.1 wt.%. These values were 244 °C and 261 °C, respectively, compared to 210 °C for the 1% Pt/TiO2 catalyst. When the temperature was increased, the differences were more significant. When comparing the T50 values of the catalysts, they increased for the 0.5% Pt/TiO2 and 0.1% Pt/TiO2 to 320 °C and 390 °C, respectively, compared to 255 °C for the 1% Pt/TiO2. The values of the reaction rate and activation energy () demonstrate that increasing the Pt loading from 0.1% to 0.5% had a relatively minor impact on the reaction rate, as it only increased from 4.7 × 10−8 to 6.3 × 10−8 mol s−1 g−1 for the 0.1% Pt/TiO2 and 0.5% Pt/TiO2 samples, respectively. Interestingly, a considerable increase in the reaction rate was seen for the 1% Pt/TiO2, as the reaction rate reached 25 × 10−8 mol s−1 g−1. To investigate the size–activity relationship of the Pt nanoparticles, a morphological investigation using TEM images for the catalysts was conducted (). The images indicate that the Pt nanoparticles were uniformly dispersed on the titania support for the 1 wt.% and 0.5 wt.% Pt/TiO2 catalysts. However, due to the low metal loading, very few particles of Pt were observed for the metal loading of the 0.1 wt.% Pt/TiO2. A slight decrease in the mean particle size was seen upon the reduction of metal loading, as it was 1.41 ± 0.3 nm and 1.33 ± 0.3 nm for the 0.5 wt.% Pt/TiO2 and 0.1 wt.% Pt/TiO2, respectively, compared to 1.63 ± 0.3 nm for the 1% Pt/TiO2. Furthermore, the TEM images reveal that the dispersion of the Pt nanoparticles decreases with an increase in metal loading as the lowest dispersion of titania-supported Pt nanoparticles was observed for the 1 wt.% Pt/TiO2. This result can explain the high activity for this sample as compared to other lower metal-loading samples. A study conducted by Park et al. (Citation2011) reported that the catalytic prowess of Pt nanoparticles is inversely proportional to the dispersion degree of the particles. Larger particles of Pt, which have lower dispersion degree, exhibited higher catalytic activity than the smaller ones (Park et al., Citation2011). Another study, conducted by Sellick, Morgan, and Taylor (Citation2015) on silica-supported Pt catalysts for the total oxidation of polyaromatic hydrocarbons supported this assumption. Furthermore, the values of the activation energy calculated for the different levels of metal loading support this claim, as the activation energy decreases from 39.49 kJ mol−1 to 21.28 kJ mol−1 when the metal loading is increased from 0.1 wt.% (particle size = 1.33 ± 0.3 nm) to 1 wt.% (particle size = 1.63 ± 0.3 nm), respectively, reflecting that the particle size factor significantly impacts the oxidation of propane over the Pt/TiO2 catalyst.
Figure 8. TEM images and the corresponding particle size distribution for (a) 0.1% Pt/TiO2, (b) 0.5% Pt/TiO2.

Another explanation for the increase in catalytic activity with an increase in the metal loading could be that the higher metal loading of the 1% Pt/TiO2 can provide more active sites for the catalyst as compared to the 0.5% Pt/TiO2 and 0.1% Pt/TiO2.
Carbon monoxide chemisorption measurements could be conducted to determine the active sites of the catalysts, but it was not possible to conduct the measurements due to the existence of the stabilizer (PVA) that was used during the synthesis of the catalyst. The presence of PVA affects surface adsorption of CO. Similar behavior has been reported previously for catalysts prepared via the sol immobilization method (Albilali et al., Citation2018; Chinthaginjala, Villa, Su, Mojet, & Lefferts, Citation2012).
The sol-immobilization method employed in this study allows for a maximum metal loading of 1% by weight. Attempts to immobilize metal loadings exceeding 1% have proven unsuccessful during the immobilization process. In our research, we examined three different metal loadings: 0.1%, 0.5%, and 1% by weight of platinum nanoparticles (Pt NPs), with the highest loading achieved being 1% by weight on TiO2. It is not feasible to increase the metal loading beyond 1% in order to obtain larger particles.
3.5. Catalyst stability
To evaluate the stability of the most active catalyst (1% Pt/TiO2(500)), a series of light-off experiments were carried out by cycling the same catalyst through four consecutive runs. The results are presented in . A slight deactivation of the catalyst was observed in the second run, as the value of T50 increased by 20.8% as the temperature rose from 255 °C to 308 °C. Interestingly, no further catalyst deactivation was observed in the third run; the T50 then was 310 °C. Further deactivation was observed during the fourth cycle, as the value of T50 was 352 °C, a 38% increase as compared to the first cycle. The deactivation of the catalyst can be a result of different factors, such as an increase in CO2 (or other species) surface concentration during the oxidation reaction or the loss of surface active sites (Avila et al., Citation2014). Further investigation is required to determine the reasons behind such deactivation.
4. Conclusions
Supported noble metal nanoparticle catalysts, including Pd, Pt, and Au prepared via the sol-immobilization technique, were found to be quite effective for the total oxidation of propane. Among the catalysts tested, 1% Pt/TiO2 exhibited the greatest catalytic behavior. The catalytic performance of 1% Pt/TiO2 was enhanced by the elevation of the calcination temperature from 300 °C to 500 °C, a change that could be a consequence of the increase in particle size. The catalytic activity and reaction rates for Pt/TiO2 increase with the increase in Pt loading from 0.1 wt.% to 1 wt.% because the catalytic activity of Pt nanoparticles is inversely proportional to the dispersion degree of the particles. Larger particles of Pt (formed at higher metal loading) have a lower dispersion degree and exhibited higher catalytic activity than smaller ones, which have a greater dispersion degree and lower metal loading. The size–activity relationship was examined based on TEM images and XPS results, and these data indicated that the particle size effect has a greater influence on the catalytic activity of the Pt nanoparticles than did the oxidation state of the surface.
Acknowledgements
Albilali gratefully acknowledges Professor Stuart H. Taylor from Cardiff University for his useful discussions and scientific support and Cardiff Catalysis Institute, School of Chemistry at Cardiff University for providing access to their facilities. Special thanks goes to Dr. Thomas Davies for TEM analysis and Dr. David Morgan for his help in the XPS data collection. The author is also grateful to Imam Abdulrahman Bin Faisal University for its continued support.
Disclosure statement
No potential conflict of interest was reported by the author(s).
References
- Albilali, R., Douthwaite, M., He, Q., & Taylor, S. H. (2018). The selective hydrogenation of furfural over supported palladium nanoparticle catalysts prepared by sol-immobilisation: Effect of catalyst support and reaction conditions. Catalysis Science & Technology, 8(1), 252–267. doi:10.1039/C7CY02110K
- Avila, M. S., Vignatti, C. I., Apesteguía, C. R., & Garetto, T. F. (2014). Effect of support on the deep oxidation of propane and propylene on Pt-based catalysts. Chemical Engineering Journal, 241, 52–59. doi:10.1016/j.cej.2013.12.006
- Bahruji, H., Bowker, M., Hutchings, G., Dimitratos, N., Wells, P., Gibson, E., … Lalev, G. (2016). Pd/ZnO catalysts for direct CO2 hydrogenation to methanol. Journal of Catalysis. 343, 133–146. doi:10.1016/j.jcat.2016.03.017
- Baranowska, K., & Okal, J. (2016). Performance and stability of the Ru-Re/γ-Al2O3 catalyst in the total oxidation of propane: Influence of the order of impregnation. Catalysis Letters, 146(1), 72–81. doi:10.1007/s10562-015-1619-z
- Camposeco, R., Castillo, S., & Zanella, R. (2023). Catalytic oxidation of propane and carbon monoxide by Pd nanoparticles on Mn/TiO2 catalysts. Catalysis Letters, doi:10.1007/s10562-023-04285-3
- Cavaliere, S., Subianto, S., Chevallier, L., Jones, D. J., & Rozière, J. (2011). Single step elaboration of size-tuned Pt loaded titania nanofibres. Chemical Communications, 47(24), 6834–6836. doi:10.1039/C1CC11716E
- Chen, Y. S., Cao, Y. D., Ran, R., Wu, X. D., & Weng, D. (2018). Controlled pore size of Pt/KIT-6 used for propane total oxidation. Rare Metals, 37(2), 123–128. doi:10.1007/s12598-017-0937-2
- Chinthaginjala, J. K., Villa, A., Su, D. S., Mojet, B. L., & Lefferts, L. (2012). Nitrite reduction over Pd supported CNFs: Metal particle size effect on selectivity. Catalysis Today. 183(1), 119–123. doi:10.1016/j.cattod.2011.11.003
- Choudhary, T. V., Banerjee, S., & Choudhary, V. R. (2002). Catalysts for combustion of methane and lower alkanes. Applied Catalysis A: General, 234(1–2), 1–23. doi:10.1016/S0926-860X(02)00231-4
- Debecker, D. P., Farin, B., Gaigneaux, E. M., Sanchez, C., & Sassoye, C. (2014). Total oxidation of propane with a nano-RuO2/TiO2 catalyst. Applied Catalysis A: General, 481, 11–18. doi:10.1016/j.apcata.2014.04.043
- Duan, J., Tan, J., Yang, L., Wu, S., & Hao, J. (2008). Concentration, sources and ozone formation potential of volatile organic compounds (VOCs) during ozone episode in Beijing. Atmospheric Research. 88(1), 25–35. doi:10.1016/j.atmosres.2007.09.004
- Flagan, R. C., & Seinfield, J. H. (1988). Fundamentals of air pollution engineering (1st ed.). Hoboken, NJ: Prentice-Hall Inc.
- Garcia, T., Agouram, S., Taylor, S. H., Morgan, D., Dejoz, A., Vázquez, I., & Solsona, B. (2015). Total oxidation of propane in vanadia-promoted platinum-alumina catalysts: Influence of the order of impregnation. Catalysis Today. 254, 12–20. doi:10.1016/j.cattod.2015.01.038
- Gurjar, B. R., Jain, A., Sharma, A., Agarwal, A., Gupta, P., Nagpure, A. S., & Lelieveld, J. (2010). Human health risks in megacities due to air pollution. Atmospheric Environment. 44(36), 4606–4613. doi:10.1016/j.atmosenv.2010.08.011
- Hasan, M. A., Zaki, M. I., & Pasupulety, L. (2002). IR investigation of the oxidation of propane and likely C3 and C2 products over group IVB metal oxide catalysts. The Journal of Physical Chemistry B, 106(49), 12747–12756. doi:10.1021/jp0214413
- He, C., Cheng, J., Zhang, X., Douthwaite, M., Pattisson, S., & Hao, Z. (2019). Recent advances in the catalytic oxidation of volatile organic compounds: A review based on pollutant sorts and sources. Chemical Reviews, 119(7), 4471–4568. doi:10.1021/acs.chemrev.8b00408
- Hu, Z., Wang, Z., Guo, Y., Wang, L., Guo, Y., Zhang, J., & Zhan, W. (2018). Total oxidation of propane over a Ru/CeO2 catalyst at low temperature. Environmental Science & Technology, 52(16), 9531–9541. doi:10.1021/acs.est.8b03448
- Huang, H., Xu, Y., Feng, Q., & Leung, D. Y. C. (2015). Low temperature catalytic oxidation of volatile organic compounds: A review. Catalysis Science & Technology, 5(5), 2649–2669. doi:10.1039/C4CY01733A
- Khudorozhkov, A. K., Chetyrin, I. A., Bukhtiyarov, A. V., Prosvirin, I. P., & Bukhtiyarov, V. I. (2017). Propane oxidation Over Pd/Al2O3: Kinetic and in situ XPS study. Topics in Catalysis, 60(1–2), 190–197. doi:10.1007/s11244-017-0733-0
- Liu, L., Han, W., Dong, F., Feng, H., & Zhicheng, T. (2022). Construction of framework confined ordered mesoporous Pt/TixAlOy catalysts and applied for the catalytic oxidation of propane. Microporous and Mesoporous Materials, 341, 112111. doi:10.1016/j.micromeso.2022.112111
- Neyertz, C., Volpe, M., & Gigola, C. (2004). Methane combustion over Pd/γ-Al2O3 and Pd-VOx/γ-Al2O3 catalysts. Applied Catalysis A: General, 277, 137–145. doi:10.1016/j.apcata.2004.09.004
- Okal, J., & Zawadzki, M. (2009). Influence of catalyst pretreatments on propane oxidation over Ru/γ-Al2O3. Catalysis Letters, 132(1–2), 225–234. doi:10.1007/s10562-009-0100-2
- Otto, K., Andino, J. M., & Parks, C. L. (1991). The influence of platinum concentration and particle size on the kinetics of propane oxidation over Pt/γ-alumina. Journal of Catalysis. 131(1), 243–251. doi:10.1016/0021-9517(91)90341-Z
- Park, J. E., Kim, B. B., & Park, E. D. (2015). Propane combustion over Pt/Al2O3 catalysts with different crystalline structures of alumina. Korean Journal of Chemical Engineering, 32(11), 2212–2219. doi:10.1007/s11814-015-0062-6
- Park, J. E., Kim, K. B., Kim, Y. A., Song, K. S., & Park, E. D. (2013). Effect of Pt particle size on propane combustion over Pt/ZSM-5. Catalysis Letters, 143(11), 1132–1138. doi:10.1007/s10562-013-1140-1
- Park, J. E., Kim, K. B., Seo, K. W., Song, K. S., & Park, E. D. (2011). Propane combustion over supported Pt catalysts. Research on Chemical Intermediates, 37(9), 1135–1143. doi:10.1007/s11164-011-0379-7
- Ramadhas, A. S. (2011). Alternative fuels for transportation (6th ed.). Boca Raton, FL: CRC Press.
- Ruth, K., Hayes, M., Burch, R., Tsubota, S., & Haruta, M. (2000). The effects of SO2 on the oxidation of CO and propane on supported Pt and Au catalysts. Applied Catalysis B: Environmental, 24(3–4), L133–L138. doi:10.1016/S0926-3373(99)00100-9
- Ryerson, T. B., Trainer, M., Holloway, J. S., Parrish, D. D., Huey, L. G., Sueper, D. T., … Fehsenfeld, F. C. (2001). Observations of ozone formation in power plant plumes and implications for ozone control strategies. Science, 292(5517), 719–723. doi:10.1126/science.1058113
- Schick, L., Sanchis, R., González-Alfaro, V., Agouram, S., López, J. M., Torrente-Murciano, L., … Solsona, B. (2019). Size-activity relationship of iridium particles supported on silica for the total oxidation of volatile organic compounds (VOCs). Chemical Engineering Journal, 366(February), 100–111. doi:10.1016/j.cej.2019.02.087
- Sellick, D., Morgan, D., & Taylor, S. (2015). Silica supported platinum catalysts for total oxidation of the polyaromatic hydrocarbon naphthalene: An investigation of metal loading and calcination temperature. Catalysts, 5(2), 690–702. doi:10.3390/catal5020690
- Shil, A. K., & Das, P. (2013). Solid supported platinum(0) nanoparticles catalyzed chemo-selective reduction of nitroarenes to N-arylhydroxylamines. Green Chemistry, 15(12), 3421–3428. doi:10.1039/c3gc41179f
- Taylor, M. N., Zhou, W., Garcia, T., Solsona, B., Carley, A. F., Kiely, C. J., & Taylor, S. H. (2012). Synergy between tungsten and palladium supported on titania for the catalytic total oxidation of propane. Journal of Catalysis, 285(1), 103–114. doi:10.1016/j.jcat.2011.09.019
- Viéitez-Calo, S., Morgan, D. J., Golunski, S., Taylor, S. H., & Twigg, M. V. (2021). Structure sensitivity and hydration effects in Pt/TiO2 and Pt/TiO2–SiO2 catalysts for NO and propane oxidation. Topics in Catalysis, 64(17–20), 955–964. doi:10.1007/s11244-021-01415-2
- Villa, A., Wang, D., Dimitratos, N., Su, D., Trevisan, V., & Prati, L. (2010). Pd on carbon nanotubes for liquid phase alcohol oxidation. Catalysis Today. 150(1–2), 8–15. doi:10.1016/j.cattod.2009.06.009
- Wang, Z., Wang, W., Khalid, O., Weber, T., Spriewald Luciano, A., Zhan, W., … Over, H. (2022). Supported RuxIr1-xO2 mixed oxides catalysts for propane combustion: Resistance against water poisoning. ChemCatChem, 14(12), e202200149. doi:10.1002/cctc.202200149
- Yang, A. C., Zhu, H., Li, Y., & Cargnello, M. (2021). Support acidity improves Pt activity in propane combustion in the presence of steam by reducing water coverage on the active sites. ACS Catalysis, 11(11), 6672–6683. doi:10.1021/acscatal.1c01280
- Zhu, X., Cheng, B., Yu, J., & Ho, W. (2016). Halogen poisoning effect of Pt-TiO2 for formaldehyde catalytic oxidation performance at room temperature. Applied Surface Science. 364, 808–814. doi:10.1016/j.apsusc.2015.12.115