ABSTRACT
Halogenated organic compounds (HOCs) are prevalent in environmental waters, but current analytical techniques are unable to identify and quantify all individual HOCs. To enhance the understanding of unregulated and unknown HOCs, total organic halogen (TOX) is proposed as a total quantity index for the overall content of HOCs in waters, and has achieved significant progresses. This paper presents a review of TOX analysis from perspectives of detection and pretreatment methods, including the measurement of halide ion (IX) or TOX content by several instruments; separation methods with enrichment based on activated carbon-adsorption, solid-phase extraction, and liquid-liquid extraction, as well as without enrichment based on the subtraction method and IX removal. The relevant fundamentals, operational processes, and key influencing factors are especially concerned. This review should be useful in understanding TOX analysis and the risk of HOCs in waters, and calls for developing more efficient TOX measurement approaches in the future.
GRAPHICAL ABSTRACT
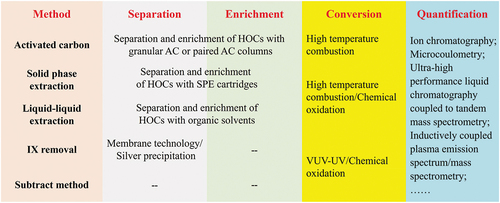
1. Introduction
Halogenated organic compounds (HOCs) are organic compounds with one or more halogen atoms bonded to the carbon. HOCs are abundant in aquatic environments, with anthropogenic and natural sources constituting their primary sources [Citation1], [Citation2]. The mass production and release of anthropogenic HOCs cause many environmental problems [Citation1]. These chemicals, such as organochlorine pesticides, perfluorinated compounds, and polychlorinated biphenyls, are often environmentally persistent, bioaccumulative, long-distance transportable, and highly toxic [Citation3]. Halogenation of dissolved organic matter (DOM) is an important natural source of HOCs in the water environment, and the most prominent of these are disinfection byproducts (DBPs) [Citation4,Citation5], which have aroused considerable attention due to their potential adverse health effects such as bladder cancer, rectal cancer, premature births, miscarriages, and birth defects [Citation5,Citation6].
The occurrence and risk of HOCs in aquatic environments promote their environmental analysis and risk evaluation. Identifying individual HOCs relies primarily on chromatography-mass spectrometry methods [Citation3,Citation7]. Recently, liquid chromatography-based methods have been widely used in detecting and identifying new DBPs [Citation8]. However, a substantial portion of HOCs is still unknown, especially those are formed by halogenating DOM precursors, which are truly unknown compounds [Citation7]. Because of the extreme complexity and heterogeneity of DOM, the formed HOCs have complex compositions and structures, thereby making them difficult to identify and quantify. Another problem with analyzing HOCs is that currently unidentified HOCs are often absent from existing mass spectrometry databases, leaving the unavailability of standards to confirm tentative identifications [Citation7]. Consider DBPs as an example, over 700 of them have been identified to date, and many have been confirmed by synthetic standards [Citation7]. Even so, the identified and quantifiable DBPs only cover a small part of all DBPs in terms of their occurrences and toxic effects [Citation4,Citation5,Citation7]. Studies have shown that many unregulated and unrecognized DBPs are much more toxic than regulated DBPs [Citation5,Citation6]. This implies that the largely unidentified HOCs cannot be ignored and need to be quantified. However, due to the great difficulty in measuring all HOCs and assessing their toxicity, total organic halogen (TOX) has been proposed as a total quantity index to quantify the overall concentration of HOCs in environmental waters.
TOX has dramatically advanced our understanding of generating unknown and unidentifiable HOCs and their toxicity in environmental waters. An earlier review concluded that the quantifiable DBPs accounted for around 50% of TOX in chlorinated waters and less than 20% in chloramine and chlorine dioxide disinfected waters [Citation9]. Some studies demonstrated that the toxicity of unregulated DBPs was higher than the regulated DBPs, and the decrease of TOX contents significantly reduced the toxicity of the disinfected waters, indicating the importance of TOX measurement for water safety and human health [Citation10–12]. From the aspect of operating conditions, TOX refers to the total amount of halogens chemically bound to organic compounds measured under specific analytical conditions. The adsorbed HOCs from water samples by activated carbon (AC) are commonly referred to as absorbable organic halogen (AOX). A number of standard analysis methods for TOX have been developed internationally as a total quality indicator, including ISO 9562 published by the International Organization for Standardization, EPA 1650/9020B provided by the US Environmental Protection Agency, APHA 5320B from the American Public Health Association, ASTM 4744 from the American Society for Testing and Materials, GB/T 15,959 and HJ/T 83–2001 from the Chinese Ministry of Ecology and Environment., etc.
This review begins with the TOX and halide ions (IX) detection methods. Then, it focuses on currently available separation methods for HOCs from waters with enrichment based on AC-adsorption, solid-phase extraction, and liquid-liquid extraction, and without enrichment based on subtraction methods (i.e. subtracting IX content from the total halogen (TX)) and removing IX. For pretreatment and detection procedures, the emphasis is placed on the fundamentals, operational processes, and key influencing factors. The purpose of this review is to facilitate comprehension of the available TOX analysis approaches, and it should be of relevance and benefit for the measurement of TOX in waters and the future development of more efficient alternatives.
1.1. Detection methods for TOX and IX
TOX concentrations are mainly determined by converting TOX into IX and usually measured by microcoulometry (MC) and ion chromatography (IC). Currently, MC and IC are the most common methods for IX detection. MC method is based on the silver-halide reaction, which can cause the current change and signal [Citation4,Citation13]. MC has a method detection limit (MDL) as low as 1.0 µg/L () [Citation4,Citation13]. MC is easy to use and has a low cost, but it is unable to differentiate total organic chlorine (TOCl) from total organic bromine (TOBr) and total organic iodine (TOI) [Citation4]. Thus, TOX measurements by MC are given in chloride equivalents, i.e. the concentration of TOX is reported as an equivalent concentration of organically bound chlorine [Citation14]. Because bromide and iodide are heavier than chloride, and brominated and iodinated DBPs are more toxic than their chlorinated analogs [Citation15], the measured TOX using MC is likely to underestimate the mass and toxic contribution of TOBr and TOI [Citation4,Citation13,Citation14]. Despite this, MC is still widely used for analyzing bulk TOX as a conventional detector.
Table 1. A summary of detection methods for TOX analysis.
IC has emerged as a popular technique of sensitive halide detection in recent decades. It can distinguish among different halides to separately measure halogen-specific TOX in waters (as TOCl, TOBr, and TOI). The MDLs of IC with conductivity and UV detector are typically at ≤10 μg/L for TOCl, TOBr, and TOI, improvements in IC detector technology have increased the sensitivity of halide detection (). The determination conditions for halide analysis have been optimized using various IC columns and IC eluents [Citation13]. For IC with a conductivity detector, Thermofisher AS14 shows good separation of Cl– and Br–, AS16 is appropriate for the separation of I– [Citation13], and AS19 gives good separation of all IX (Cl–, Br–, and I–) [Citation14]. IC eluents mainly use carbonate and hydroxide solution; the former has a stronger anion exchange capacity than the latter and is suitable for detecting I–, while the latter has relatively lower background interference and is suitable for detecting trace IX [Citation4]. The concentration and flow rate of eluents also can significantly impact the separation and detection of IC IX [Citation4]. IC mainly primarily uses a conductivity detector to detect all IX, and some IC use a UV detector for Br– and I– analysis due to their absorption of UV light [Citation4,Citation13].
Inductively coupled plasma mass spectrometry (ICP-MS) provides high sensitivity and low MDLs for TOX analysis (). Because ICP-MS can atomize and ionize elements under a high temperature, thus ICP-MS is suitable for determining IX and directly detecting organically bound halogens to achieve TOX measurement [Citation21]. However, a trace amount of chlorine is challenging to be detected by ICP-MS due to its high first ionization potential (12.967 eV) and the susceptibility of polyatomic interference of 16O18OH+ for Cl+ detection at m/z 35. This interference can be minimized under the hydrogen mass transfer mode [Citation22]. Compared to chlorine, bromine and iodine have a heavier mass and lower first ionization potential, so ICP-MS is more suitable for their detection [Citation22]. The lowest reported MDLs for TOI measurement is as low as 0.14 µg/L [Citation23]. During operation, the ICP wash solution is essential to eliminate the memory effect caused by the residual bromine and iodine, which is especially serious for iodine. This is because the iodine in an acidic solution tends to enter the ICP as volatile iodine like HI and I2, using dilute ammonia and tetramethylammonium hydroxide as the ICP wash solution can effectively eliminate the memory effect [Citation19]. Due to the higher first ionization potential of fluorine (17.42 eV), ICP-MS is unable to measure TOF, and high cost is the biggest limitation for its usage in TOX measurement. Recently, ultra-high performance liquid chromatography coupled to tandem mass spectrometry (UPLC-MS/MS) has also been used for TOI detection, which is performed in negative ion mode at a m/z of 127 [Citation20]. The quantification limit of TOI can be as low as 5 µg/L for a 40 mL water sample and 2.5 µg/L for an 80 mL water sample [Citation20].
1.2. TOX measurement based on AC adsorption
Due to the low concentrations of HOCs in aquatic environments, TOX analysis usually starts with separating and enriching HOCs from water samples and removing inorganic halides and other substances. Because adsorption on solid substrates is preferred for separating and enriching HOCs in the aqueous environment, AC is regarded as the most suitable substrate due to its porous structure and high specific surface area (500–1700 m2/g). Therefore, TOX determination based on AC separation and adsorption is currently the most widely used analytical method for TOX both domestically and internationally [Citation5]. AC is a porous carbon-based material that is made from carbon-based materials like wood, coal, and fruit shells that have been activated at high temperatures and under anoxic conditions at high temperatures. AC can only recover the adsorbable fraction of HOCs; therefore, TOX determined using AC-based methods is also referred to as AOX [Citation4]. Nowadays, most individuals accept and acknowledge that AOX and TOX are equivalent [Citation4].
Using AC separation and adsorption to determine TOX comprises three primary steps [Citation4,Citation13,Citation14]: 1) separation of HOCs by AC adsorption [Citation2]; 2) conversion of HOCs to IX in a high-temperature combustion furnace [Citation3]; 3) determination of IX by MC and IC; the detailed steps and conditions of which are shown in [Citation4,Citation13]. For the first step, the water sample is first acidified to pH ≤ 2 with an acidic solution such as nitric acid, which is to protonate HOCs to enhance their adsorption onto AC, as well as inhibits microbial activity [Citation4,Citation14,Citation24]. Regarding the adsorption of HOCs onto AC, the batch shaker procedure and the adsorption column method are the two most described [Citation9]. In the first method, granular AC is introduced to the water sample in a volumetric flask, and HOCs are adsorbed by shaking for at least one hour. Granular AC is separated from the water by filtration, usually a quartz filtering membrane [Citation9]. For the latter, the acidified water sample is passed through a pair of mini-columns often mounted in a series at a flow rate of approximately 3 mL/min with an inert gas.
The quality of AC is crucial to recovery of TOX, particularly in terms of its adsorption performance, background halogen content, and reducibility. To ensure the optimum recovery of HOCs, standard methods require that the TOX adsorbed by the second AC column should not exceed 10.0% or 25.0% of the total adsorbed TOX. However, some researchers consider that a ratio of at least 2.0 between the TOX adsorbed by the first AC column and the second column is sufficient [Citation19]. High-quality AC yields good recovery for the majority of HOCs (>90%) [Citation13,Citation14,Citation25], but is poor in the adsorption of polar, low molecular weight, hydrophilic, and highly soluble HOCs () [Citation4,Citation26,Citation27].
Table 2. Recoveries of common halogenated organic compounds.
Halogen impurity is another concern for AC since it can interfere with or introduce bias into the TOX analysis [Citation4,Citation14,Citation47]. It has been reported that the background concentration of TOCl in various ACs (produced from coconut shells, coal, synthetic carbon, molecular sieves, and lignite) ranges from 3.6 µg Cl/L to 738.0 µg Cl/L [Citation14]. Even after nitric acid washing, certain commercial ACs still contain up to 307.0 g Cl/L of background noise [Citation14]. To eliminate background errors, AC for TOX analysis should be as halogen-free as possible, and all current standard methods require the background halogen to be ≤1 µg Cl/40 mg AC [Citation4]. Compared to chlorine, the background fluorine of AC products is substantially lower, ranging from 0.08 to 9.22 µg F/g [Citation27]. Meanwhile, it should prevent introducing halogens during the AC storage process. In the same way, the amount of IX that AC adsorbed should be as low as possible to eliminate background noise, and it has been demonstrated that coconut shell-based AC from CPI retained significantly less IX than coal-based AC from Calgon [Citation13]. The interference of AC-based TOX analysis can result from the reduction potential of AC, which may result in the reduction of HOCs during AC adsorption and the release of IX, thereby underestimating the amount of TOX [Citation26]. This can be avoided by oxidizing the AC in the right way to enhance its oxygen-containing functional groups. However, adding too much oxidant will impair the AC’s adsorption ability for HOCs [Citation4,Citation48,Citation49].
Since IX can cause an overestimation of TOX, and high levels of dissolved organic carbon (DOC) can reduce AC adsorption sites and thus the adsorption performance of HOCs, current standards limit dissolved IX and DOC levels to less than 1 g/L and 100 mg/L, respectively [Citation9]. There is no consensus about the influence of IX on the measurement of TOX using the AC method, with some research indicating that 500 mg/L of Cl– has no effect on the determination of TOX [Citation45]. However, it has shown that 13–27 µg Cl/L of TOX can be detected when spiked with 400 mg/L of Cl–, TOX can be detected in terms of Cl– concentrations as low as 100 mg/L [Citation50]. AC has a higher adsorption capacity for inorganic iodine than other IXs; hence, it can interfere with the detection of adsorbable organic iodine (AOI) when the concentrations of IO3– and I– exceed 100 g/L and 500 g/L [Citation14]. At the operational level, the volume of nitrate solution for rinsing the AC needs to be optimized; In general, for most samples containing 50–500 mg Cl/L and 500–5000 mg Cl/L of chlorine, 2.5 mL and 5.0 mL of potassium nitrate solution (5 g/L) are effective in removing the retained or background IX in the column, and too large a volume tends to reduce TOX recovery [Citation14,Citation51]. In addition, 25–100 mL of water is often sufficient for TOX determination. Water samples containing high levels of TOX and IX must be diluted before being separated with AC [Citation14].
The conversion of HOCs to IX prior to measurement is performed in a high-temperature combustion furnace: AC and the adsorbed HOCs are introduced to the furnace through a combustion boat (typically made of quartz or ceramic material), where the AC and its adsorbed organic carbon are burned to carbon dioxide, the AC-bound HOCs are burned to hydrogen halide and halogen gas in the presence of O2. O2 flow rate, combustion temperature and time, and an inorganic halogen gas trapping solution are the main factors influencing the conversion process of HOCs. Previous studies have shown that the flow rate of O2 between 300 and 500 mL/min has no significant effect on TOX measurement [Citation13], and increasing the O2 flow rate from 150 mL/min to 200 mL/min has little effect on TOX determination [Citation14]. To completely combust organic carbon and HOCs, and the temperature of the combustion furnace is usually set to > 800°C [Citation5], the combustion time is suggested to be set to 10–12 min [Citation4]. The combustion condition can be maintained for 10 min after the complete combustion of HOCs, which enables the continuous conversion of partially burned OX and a more thorough transfer of combustion gases to the trapping solution, hence boosting the recovery of TOX [Citation50].
Furthermore, it has been demonstrated that introducing ultrapure water into the combustion furnace during the combustion process enhances the conversion of TOX to IX. Because IX may be condensed and retained in the exhaust pipe, some researchers have suggested heating the exhaust pipe and flushing it at the end of the combustion to recover condensed IX, or drying the exhaust gas from the combustion furnace with concentrated sulphuric acid before entering the exhaust pipe [Citation4,Citation13]. Typically, the trapping solution is usually 20 mL of deionized water [Citation4,Citation13,Citation14]. Since high-temperature combustion of HOCs can yield halogen gas and halogen oxides, adding reducing agents to the trapping solution can help reduce them to IX. Currently, Na2S2O3 is a well-studied reducing agent and has been proven to be conducive to TOX detection [Citation4,Citation27]. In addition, acetic acid and sodium borate can be added to the trapping solution to determine IX using MC and IC, respectively [Citation4].
1.3. TOX measurement based on solid phase extraction
Solid phase extraction (SPE), which can tolerate high contents of inorganic ions and organics, has also been proposed to separate and enrich HOCs from water samples [Citation52]. The SPE extracted TOX is HOCs that can be adsorbed by the adsorbent and eluted by the solvent [Citation4], the eluate is then measured using IC or MC after converting it to IX by high-temperature combustion, or using ICP-MS after drying and redissolving it in ultrapure water [Citation21,Citation53–55]. The operational step is similar to the classical SPE process (), in which the water sample is first acidified to pH < 2 to protonate HOCs, the acidified water is passed through a pre-activated SPE cartridge. After rinsing the residual IX with acidified water or salt solution, SPE cartridge is eluted with an organic solvent to elute the adsorbed HOCs.
Due to the diversity and structural complexity of HOCs, the adsorbent and elution solvent play a crucial role in the SPE method for TOX determination. A study has evaluated the enrichment efficiency of different SPE adsorbents and their combinations for non-volatile TOX [Citation11], including HLB, ENV+, Lichrolut, StrataX, TELOS ENV, WAX, and MAX cartridges, as well as XAD-8/2, coconut charcoal activated carbon (CC)-HLB, HLB-CC, and Strata X-AW/Strata X-CW/ENV+ (in top-down order), the elution solvents were mainly methanol and methyl tert-butyl ether. The recoveries in terms of TOCl were found to be HLB-CC (68%) ≈ TELOS ENV (67%) > HLB (51%) ≈ Lichrolut (49%) ≈ StrataX (49%) ≈ ENV+ (48%) > mixed-bed (36%) ≈ WAX (34%) ≈ XAD-8/2 (33%) > MAX (27%) ≈ CC-HLB (27%) [Citation11]. The adsorbents of HLB-CC, HLB, TELOS ENV, Lichrolut, StrataX, and ENV+ are all polystyrene diethylene benzene or polydivinylbenzene polymers; hence, their recoveries (50–70%) were comparable [Citation11]. This study also indicated that a low pH was beneficial in improving TOX recoveries, but too low a pH tended to modify the adsorbent, hence affecting its sorption performance [Citation11]. Although XAD resins have been used extensively for extracting DBPs in the past and showed near 100% recoveries for model compounds such as 4-chlorophenol [Citation52], they are unsuitable for enriching some HOCs such as long-chain aliphatic HOCs and haloacetic acids (), resulting in the low recovery in terms of TOX [Citation1,Citation28]. In another study, XAD resins and adsorbents containing strong anion-exchange adsorbents and surface-modified styrene divinylbenzene adsorbents were used to separate and enrich (semi-)volatile and non-volatile HOCs [Citation32]. Both adsorbents showed limited recovery for (semi-)volatile HOCs; however, the polymeric adsorbent paired with methyl tert-butyl ether elution exhibited a better recovery, showing the impact of the elution solvent on the recovery of HOCs [Citation32]. The method was applied to separate and enrich DBPs from 10 L of disinfected waters, and recoveries of both TOCl and TOBr were found to be below 30%, primarily due to the loss of (semi-)volatile DBPs which accounted for approximately 30% of TOX. That is, the SPE method is more favorable for the extraction of HOCs with a relatively large logKow and low vapor pressure [Citation32], and not favorable for HOCs with high hydrophilicity and vapor pressure (). In addition to the SPE cartridge, SPE discs have also been used to enrich HOCs from water, and poly(styrene-diethylene-benzene)-modified discs provided high recoveries (82–99%) for most model HOCs, except for a few substances such as haloacetic acid and dieldrin [Citation1]. The study also compared the elution efficiency of HOCs with different solvents and found that 2-propyl alcohol was the best solvent under experimental conditions [Citation1]. Unfortunately, the method performance was only evaluated using 4-chlorophenol as an internal standard for the recovery rate (up to 102–107%), and the overall HOCs recovery was not evaluated.
SPE method has high recoveries for certain HOCs. To determine the total amount of contrast agent in municipal wastewater, Fono et al. employed a C18 cartridge to retain the non-polar iodinated organics and an Isolute ENV+ column to separate and enrich the polar X-ray contrast agent [Citation56]. The eluted contrast agent from the Isolute ENV+ column was subsequently converted to I– in a reaction system containing Cu (II) and hydrogen peroxide solution, and I– was measured by liquid chromatography with detection wavelength at 226 nm, yielding recoveries of 74.8%, 73.6%, and 66.2% for pantothenic acid, iodopropamine, and iodoamine, respectively [Citation56]. Perfluorinated compounds (PFCs) have attracted much attention due to their persistence and wide distribution in the environment, for which Miyake et al used a weak anion exchange cartridge (Oasis WAX) to separate PFCs from waters and converted the enriched PFCs to F–, yielding recoveries ranging from 81 to 109% for a broad spectrum of PFCs [Citation57]; Recently, Forster et al. used Strata cartridge (WAX/graphitized carbon black) to develop a new sensitive method to quantify TOF in industrial wastewater and river water, achieving higher overall recoveries (72–91%) for a larger mix of 39 PFCs [Citation58].
1.4. TOX measurement based on liquid-liquid extraction
Liquid-liquid extraction (LLE) is another commonly employed technique for TOX detection that relies on separating HOCs from water using an organic solvent or extractant [Citation4,Citation24]. Wegmann and Greve utilized petroleum ether to extract HOCs from surface water as early as 1977. The extract was concentrated to 1 mL with gentle nitrogen blowing before being converted to IX in a combustion furnace [Citation59]. Owing to the high concentration rate, this approach has a detection limit as low as 1 µg Cl/L [Citation59]. In order to achieve excellent separation between the solvent used for LLE extraction and water, mostly non-polar and weakly polar solvents are employed, popular solvents include n-hexane, n-pentane, ethyl ether, and methyl tert-butyl ether (MtBE) [Citation24]. Among them, MtBE is utilized extensively in the separation and extraction of HOCs due to its high volatility and extraction capacity [Citation24]. MtBE can extract HOCs by polar-polarity, hydrogen bond forces, and van der Waals forces. MtBE has a high solubility in water due to its ability to operate as both a hydrogen bond donor and acceptor, resulting in no substantial biphasic separation [Citation24]. By adjusting the pH of water samples, acidic, neutral, or basic HOCs can be extracted selectively [Citation24]. LLE necessitates the addition of an inert salt to enhance the ionic strength of water, hence enhancing the extraction efficiency of HOCs and allowing the separation of the organic and aqueous phases; the most widely employed salt is anhydrous sodium sulfate [Citation24]. Like SPE, LLE has a high recovery rate for HOCs with a relatively large logKow and low vapor pressure (). Overall, the TOX value measured based on LLE is smaller than that based on the AC adsorption method [Citation4].
1.5. TOX measurement based on subtraction method
The above three TOX measurement methods are based on separating and enriching HOCs from waters, but they usually face high cost, labor-intensive operation procedures, and/or low TOX recovery. Hence, researchers are developing new and simple TOX analysis methods. The core of these methods is the determination of TOX and the converted IX from water samples without enriching HOCs (). There are two main approaches to achieve this target; The first is the subtraction method, i.e. determining TOX based on the difference between the TX content and the IX content [Citation53,Citation60]; The second is to remove the IX from waters, then TOX is determined as the total amount of the retained HOCs and possibly trace IX [Citation61,Citation62].
Currently, the subtraction method is primarily used for detecting TOBr and TOI. For example, Yang et al. employed ICP-MS to determine total bromine and total iodine, and high-performance liquid chromatography coupled with ICP-MS to determine inorganic Br/I content [Citation53]. The difference between TI and IX was calculated as TOBr/TOI. It was found that the occurrence level of TOI in the Yangtze River increased with the direction of water flow [Citation53]. It has been reported that SPE eluent still contains a small amount of IX, and the subtraction approach may be used to eliminate the bias caused by the residual IX when measuring TOX [Citation2,Citation54,Citation55]. The subtraction method requires a high sensitivity and low detection limit for both TX and IX. In addition, the IX content should not be too high compared to TX; otherwise, this can lead to significant analytical biases [Citation47]. Therefore, if water samples contain a high content of IX, it is necessary to remove it before measuring the retained TOX [Citation4,Citation47,Citation61,Citation62].
1.6. TOX measurement based on removing IX
Given that the IX content is much higher than the TOX content in many environmental waters, several technologies have been developed for removing IX from water based on membrane separation and silver precipitation technologies. Membrane-based technologies are often used in seawater desalination and can thus be used to isolate IX from HOCs. The used membranes mainly involve ultrafiltration membrane, dialysis membrane, and ion-exchange membrane. The first two membranes for separating HOCs from IX mainly rely on the molecular retention function of the membrane. Dialysis membranes with 100 Da interception and ultrafiltration membranes with 500 Da interception have been used to separate HOCs from IX, and their respective TOX recoveries were 53.0% and 16.8%, suggesting that membranes’ interception molecular weight has a significant impact on retaining HOCs [Citation48]. However, membranes based on molecular interception cannot completely remove IX; therefore, the subtraction method is still required to obtain TOX. Since most HOCs differ substantially in molecular weight and charge from IX, electrodialysis method based on ion-exchange membranes has been employed in recent years to separate HOCs from IX in water samples [Citation61]. The method is reported to be able to remove 99% of IX in water and recover 80% of TOX in 2 h (). As the membrane technology can operate in a continuous injection mode and the solute is always in liquid form, it is expected that it will be automated in the future [Citation61]. Unfortunately, the method is not favorable for ionizable substances, hence the majority of the retained TOX are non-ionic.
Figure 4. Performance of electrodialysis separation methods on TOX measurement. (a) Influences of halide types (C0 = 10 mg/L) on IX removal with electrodialysis; (b) Effects of chloride concentrations with fixed chloral hydrate (50 µg/L) on the recovery of NTOX. Reprinted with permission from ref [Citation61]. Copyright 2018 Elsevier. NTOX represents non-ionic TOX. .
![Figure 4. Performance of electrodialysis separation methods on TOX measurement. (a) Influences of halide types (C0 = 10 mg/L) on IX removal with electrodialysis; (b) Effects of chloride concentrations with fixed chloral hydrate (50 µg/L) on the recovery of NTOX. Reprinted with permission from ref [Citation61]. Copyright 2018 Elsevier. NTOX represents non-ionic TOX. .](/cms/asset/a1289e71-41ed-4954-b87f-ce1f8a278287/tcsb_a_2203350_f0004_oc.jpg)
Due to the low solubility product constant of silver halides (AgCl: 1.8 × 10−10; AgBr: 5.3 × 10−13; AgI: 8.3 × 10−17), IX in water can be removed by the addition of Ag+ to water or passing through an Ag+ exchange cartridge. Based on this principle, Chen et al. proposed a new method for removing IX from waters by forming AgX precipitates between Ag2CO3 and IX, achieving good IX removal and TOX recovery [Citation47]. The residual Cl– and Br– were below 57.5 µg Cl/L and 42.7 µg Br/L, respectively, under the optimal conditions (molar concentration ratio of Ag+ to halide at 2:1, precipitates removed by filtration through a 0.22 µm filter membrane, the residual Ag+ removed by an H+ exchange cartridge), with 90% recovery of most HOCs achieved when concentrations of model HOCs varied in the range of 30.0–1000.0 µg/L. Although a previous study reported that IX concentration could be reduced to µg/L using Ag+ exchange cartridge, this study showed that the amount of Cl– removed by the Ag+ exchange cartridge method was limited. For example, a solution containing 10 mg/L Cl– exhibited a residual of 2.0 mg/L Cl– after passing through the Ag+ exchange cartridge [Citation47].
Due to the high cost of utilizing high sensitivity instruments such as ICP-MS and the incapacity of single quadrupole ICP-MS to detect trace Cl–, the HOCs retained after removal of IX need to be further converted to IX for IC detection. Vacuum ultraviolet mixed with ultraviolet (VUV-UV) and chemical oxidation method are the most important applicable conversion techniques now accessible. A low-pressure mercury vapor lamp is a common source of VUV radiation, emitting primarily 185 nm VUV and 254 nm UV with 10.0% and 90.0% of the output power, respectively [Citation63]. Without the addition of any reagent or catalyst, VUV photons can generate several free radicals through the homolytic cleavage and ionization of water, including oxidizing radicals such as hydroxyl radicals (·OH) and superoxide anions (O2–), as well as reducing radicals such as hydronium (e–aq) and hydrogen radicals (·H) [Citation64]. VUV can completely convert organic carbon and organic nitrogen into inorganic carbon and inorganic nitrogen. Considering that the carbon-halogen bond (C-X), except for C-F bond has weaker bond energy than C-C and C-N bonds, VUV can convert HOCs to IX by breaking the C-X bond. Bu et al. used VUV to photolyze 20 representative small molecule DBPs and some disinfection water samples [Citation62]. They found that as long as the irradiation dose was sufficient, the dehalogenation efficiency of all target compounds could reach>85.5%, regardless of the chemical structure, type, or halogenation degree of HOCs [Citation62]. Although the TOX measured based on the combination of this method with electrodialysis separation was 2.3–30.6% lower than TOX measured by the AC-based method, the two results were well correlated (R2>0.97, ) [Citation62]. After that, Chen et al. used VUV-UV photolysis to convert organic fluorine to F–, continued the conversion of organic fluorine to inorganic fluoride using VUV-UV photolysis, and the defluorination rates of 10 model fluorinated organic compounds in a tailored VUV-UV photoreactor ranged from 83.9 to 109.4% [Citation65]. Unlike other UV-based processes that favor alkaline conditions, this VUV process preferred either neutral or acidic conditions to defluorinate selected compounds. The exact mechanisms remain to be explored in the future. This indicates that VUV photodehalogenation process is accompanied by photooxidation processes and is susceptible to factors such as dissolved oxygen and pH [Citation62,Citation63].
Figure 5. Performance of UVU-UV conversion methods on TOX measurement. Comparison of organic chlorine (a) and organic bromine (b) between electrodialysis separation coupled with UVU-UV conversion method and conventional AOX methods for TOX analyses for disinfected waters. Reprinted with permission from ref [Citation62]. Copyright 2018 Elsevier. TW, SPW, and SW represent a tap water, a swimming pool water, and a synthetic water, respectively.
![Figure 5. Performance of UVU-UV conversion methods on TOX measurement. Comparison of organic chlorine (a) and organic bromine (b) between electrodialysis separation coupled with UVU-UV conversion method and conventional AOX methods for TOX analyses for disinfected waters. Reprinted with permission from ref [Citation62]. Copyright 2018 Elsevier. TW, SPW, and SW represent a tap water, a swimming pool water, and a synthetic water, respectively.](/cms/asset/a0fb0af6-020c-4acb-bd40-f2dd4fc28b43/tcsb_a_2203350_f0005_oc.jpg)
Because C-I bonds break more easily than other carbon-halogen bonds, the chemical oxidation of HOIs can be employed to release iodine. For example, X-ray contrast agents can release iodide in a CuCl2 and H2O2 system, and the conversion of three selected compounds was between 66% and 74% under optimal reaction conditions (pH adjusted to 8.2 by bicarbonate/carbonate buffer, reaction temperature and time of 80°C and 90 min, respectively) [Citation56]. Alkaline persulfate can completely oxidize I– and organoiodine to iodate, and one study used this chemical mechanism to release iodine from organoiodine chemicals. To obtain TI, the resultant iodate was reduced to I– by NaHSO3 and ascorbic acid, and then TOI was detected by HPLC-UV in the form of I– based on the subtraction method [Citation66].
1.7. Summary and outlook
Since it is almost impossible to identify and quantify all HOCs in environmental waters and evaluate their toxicity, and the currently identified HOCs cannot adequately account for adverse health effects caused by all HOCs, TOX as a bulk parameter is of great value in capturing both known and unknown HOCs that may cause health issues. Advances in analytical methods and instruments allow the separation of the different components of TOX (TOCl, TOBr and TOI), making TOX a promising alternative method for the analysis of individual HOCs. This review provides a brief overview of the fundamentals, critical operations and influencing factors of several TOX analysis methods and detection techniques. Currently, the AC adsorption-based TOX method is still the primary standard method for TOX analysis in waters; however, it is not routinely and widely used due to its labor-intensive operations, high costs, and many factors impacting the recovery of TOX. Several alternative methods are likewise burdened by labor-intensive analytical procedures, a lack of automation, and high costs. Therefore, the future necessitates the development of novel, simple, and cost-effective TOX technologies that enable routine TOX analysis in routine laboratories. New TOX analytical methods could focus on the synthesis of new adsorbents, the development of new separation and enrichment methods and conversion methods for HOCs, TOX measurement techniques with a high sensitivity and low MDL, the development of new automated pre-treatment equipment, online automatic monitoring systems, and field measurement systems based on sensor technology. At the same time, it is necessary to make full use of data on the occurrence and toxicity of HOCs, as well as big data algorithms, to construct effective models to elucidate the interaction among water quality, occurrences of HOCs and TOX, and toxicity in waters, which would aid in predicting the formation and toxicity of HOCs in waters, and promote the development of TOX substitution parameters.
Author’s contribution
All authors have read and agreed to the published version of the manuscript. Conceptualization, literature reviewing, writing and editing of the manuscript – Jing Wu
Conceptualization, literature reviewing, funding acquisition, writing and editing of the manuscript – Zhineng Hao, Mei He
Reviewing, editing, and revision of the final manuscript – Yunwen Wang, Xuexin Gong, Xiaoli Wang, Rusong Zhao, Jingfu Liu
Additional information
Funding
References
- Maya F, Estela JM, Cerdà V. Completely automated system for determining halogenated organic compounds by multisyringe flow injection analysis. Anal Chem. 2008;80(15):5799–11.
- Mendez-Diaz JD, Shimabuku KK, Ma J, et al. Sunlight-driven photochemical halogenation of dissolved organic matter in seawater: a natural abiotic source of organobromine and organoiodine. Environ Sci Technol. 2014;48(13):7418–7427.
- Ayala-Cabrera JF, Santos FJ, Moyano E. Recent advances in analytical methodologies based on mass spectrometry for the environmental analysis of halogenated organic contaminants. Trends Environ Anal Chem. 2021;30:e00122.
- Chen B, Bu Y, and Yang J, et al. Methods for total organic halogen (TOX) analysis in water: past, present and future. Chem Engg J. 2020;399:136137.
- Kristiana I, McDonald S, Joll CA. The forest or the trees: a critical review on the analysis of total organic halogen (TOX) in drinking waters and its utility as a water quality parameter. Environ Sci: Water Res Technol. 2020;6(9):2313–2330.
- Li XF, Mitch WA. Drinking water disinfection byproducts (DBPs) and human health effects: multidisciplinary challenges and opportunities. Environ Sci Technol. 2018;52(4):1681–1689.
- Richardson SD, Ternes TA. Water analysis: emerging contaminants and current issues. Anal Chem. 2022;94(1):382–416.
- Yang M, Zhang X, Liang Q, et al. Application of (LC/)MS/MS precursor ion scan for evaluating the occurrence, formation and control of polar halogenated DBPs in disinfected waters: a review. Water Res. 2019;158:322–337.
- Kinani A, Sa H, Bouchonnet S, et al. Formation and determination of organohalogen by-products in water – Part I. Discussing the parameters influencing the formation of organohalogen by-products and the relevance of estimating their concentration using the AOX (adsorbable organic halide) method. Trends Analyt Chem. 2016;85:273–280.
- Han J, Zhang X, Jiang J, et al. How much of the total organic halogen and developmental toxicity of chlorinated drinking water might be attributed to aromatic halogenated DBPs? Environ Sci Technol. 2021;55(9):5906–5916.
- Stalter D, Peters LI, O’Malley E, et al. Sample enrichment for bioanalytical asessment of disinfected drinking water: concentrating the polar, the volatiles, and the unknowns. Environ Sci Technol. 2016;50(12):6495–6505.
- Pan Y, Zhang X, Wagner ED, et al. Boiling of simulated tap water: effect on polar brominated disinfection byproducts, halogen speciation, and cytotoxicity. Environ Sci Technol. 2014;48(1):149–156.
- Hua GRD, Reckhow DA. Determination of TOCl, TOBr and TOI in drinking water by pyrolysis and off-line ion chromatography. Anal Bioanaly Chem. 2006;384(2):495–504.
- Kristiana I, McDonald S, Tan J, et al. Analysis of halogen-specific TOX revisited: method improvement and application. Talanta. 2015;139:104–110.
- Richardson SD, PLEWA M, WAGNER E, et al. Occurrence, genotoxicity, and carcinogenicity of regulated and emerging disinfection by-products in drinking water: a review and roadmap for research. Mutat ResRev Mutat Res. 2007;636(1–3):178–242.
- Eaton AD, Rice LSC, Greenberg AE. Standard methods for the examination of water and wastewater. Washington DC: American Public Health Association; 2005.
- Oleksy-Frenzel J, Wischnack S, Jekel M. Application of ion chromatography for the determination of the organic group parameters AOCl, AOBr, and AOI in water. Fresen J Anal Chem. 2000;366(1):89–94.
- Liu D, Chen G, Huo Z, et al. A study of bromine speciation in human serum and ambroxol determination in rat plasma by liquid chromatography–inductively coupled plasma mass spectrometry. Chromatographia. 2019;82(6):927–934.
- Sayess R, Reckhow DA. An improved method for total organic iodine in drinking water. Water Res. 2017;108:250–259.
- Pan Y, Zhang X. Total organic iodine measurement: a new approach with UPLC/ESI-MS for off-line iodide separation/detection. Water Res. 2013;47(1):163–172.
- Yang Y, Komaki Y, Kimura SY, et al. Toxic impact of bromide and iodide on drinking water disinfected with chlorine or chloramines. Environ Sci Technol. 2014;48(20):12362–12369.
- Nakano K. Ultra-low level determination of phosphorus, sulfur, silicon and chlorine using the agilent 8900 ICP-QQQ. Appl Note Semiconductor. 2016;5991–6852EN.
- Kimura SY, Zheng WN, Hipp T, et al. Total organic halogen (TOX) in human urine: a halogen-specific method for human exposure studies. J Environ Sci. 2017;58:285–295.
- Kinani A, Kinani S, Bouchonnet S. Formation and determination of organohalogen by-products in water – part II. Sample Preparat Techniq Anal Appr TrAc Trends Analy Chem. 2016;85:281–294.
- Langsa M, Allard S, Kristiana I, et al. Halogen-specific total organic halogen analysis: assessment by recovery of total bromine. J Environ Sci. 2017;58:340–348.
- Li Y, Zhang X, Krasner SW, et al. Penetration of polar brominated DBPs through the activated carbon columns during total organic bromine analysis. J Environ Monit. 2011;13(10):2851–2857.
- Kinani A, Salhi H, Bouchonnet S, et al. Determination of adsorbable organic halogens in surface water samples by combustion–microcoulometry versus combustion–ion chromatography titration. J Chromatogr A. 2018;1539:41–52.
- Glaze WH, Peyton GRPR, Rawley R. Total organic halogen as water quality para-meter: adsorption/microcoulometric method. Environ Sci Technol. 1977;11(7):685–690.
- Brandt G, Kettrup A. Determination of organic group parameters (AOCl, AOBr, AOs) in water by means of ion-chromatographic detection. Fresenius’ Zeitschrift für analytische Chemie. 1987;327(2):213–219.
- Reckhow DA, Hull C, Lehan E, et al. The determination of total organic halide in water: a comparative study of two instruments. Int J Environ Anal Chem. 1990;38(1):1–7.
- Symons JM, Xia R, Diehl AC, et al. The influence of operational variables on the formation of dissolved organic halogen during chloramination. Water Disinfect Natural Organ Matter. 1996;649:78–104.
- Lau SS, Forster AL, Richardson SD, et al. Disinfection byproduct recovery during extraction and concentration in preparation for chemical analyses or toxicity assays. Environ Sci Technol. 2021;55(20):14136–14145.
- Gioia MLD, Leggio A, Pera AL, et al. SPE-GC-MS analysis of chloroform in drinking water. Chromatographia. 2004;60(5–6):319–322.
- Nikolaou AD. Application of different analytical methods for determination of volatile chlorination by-products in drinking water. Talanta. 2002;56(4):717–726.
- Alexandrou LD, Spencer MJS, Morrison PD, et al. Micro versus macro solid phase extraction for monitoring water contaminants: a preliminary study using trihalomethanes. Sci Total Environ. 2015;512-513:210–214.
- Golfinopoulos SK, Nikolaou AD. Disinfection by-products and volatile organic compounds in the water supply system in Athens, Greece. J Environ Sci Health. 2001;36(4):483–499.
- Echigo S, Zhang X, Minear RA, et al. Differentiation of total organic brominated and chlorinated compounds in total organic halide measurement: a new approach with an ion-chromatographic technique. Natural Organ Matter Disinfect By-Prod. 2000;761 :330–342 .
- Sun YX, Gu P. Determination of haloacetic acids in hospital effluent after chlorination by ion chromatography. J Environ Sci. 2007;19(7):885–891.
- Kristiansen NK, Aune KT, Frøshaug M, et al. Determination of halogenated acetic acids in chlorinated sea water and drinking water produced offshore. Water Res. 1996;30(9):2155–2159.
- Xie Y, Zheng DAR, He M. Bromine and iodine species in drinking water supply system along the Changjiang River in China: occurrence and transformation. Water Res. 2021;202:117401.
- Sadia WP, Pauzi A. SPE-GC-MS for the determination of halogenated acetic acids in drinking water. Chromatographia. 2009;69(11–12):1447–1451.
- Liao X, Allen JM, Granger CO, et al. How well does XAD resin extraction recover halogenated disinfection byproducts for comprehensive identification and toxicity testing? J Environ Sci. 2022;117:264–275.
- Jung SW, Choi YW. Simultaneous fluorimetric determination of on-line preconcentrated HANs, DCAD and TCAD by using RPLC with a postcolumn derivatization system. Bull Korean Chem Soc. 2013;34(6):1783–1790.
- Ashworth DJ, Zheng W, Yates SR. Determining breakthrough of the soil fumigant chloropicrin from 120 mg XAD-4 sorbent tubes. Atmos Environ. 2008;42(21):5483–5488.
- Jekel MR, Roberts PV. Total organic halogen as a parameter for the characterization of reclaimed waters: measurement, occurrence, formation, and removal. Environ Sci Technol. 1980;14(8):970–975.
- Han J, Zhang X, Liu J, et al. Characterization of halogenated DBPs and identification of new DBPs trihalomethanols in chlorine dioxide treated drinking water with multiple extractions. J Environ Sci. 2017;58:83–92.
- Yang J, Tang Y, Pan H, et al. Precipitating halides by silver carbonate: a facile pretreatment method to enable total organic halogen analysis in water. Sep Purif Technol. 2023;305:122461.
- Li Y, Zhang X, Shang C. Effect of reductive property of activated carbon on total organic halogen analysis. Environ Sci Technol. 2010;44(6):2105–2111.
- Li Y, Zhang X, Shang C, et al. Evaluation and improvement of total organic bromine analysis with respect to reductive property of activated carbon. Water Res. 2011;45(3):1229–1237.
- Liu C, Ersan MS, Plewa MJ, et al. Formation of regulated and unregulated disinfection byproducts during chlorination of algal organic matter extracted from freshwater and marine algae. Water Res. 2018;142:313–324.
- Ivanova P, Stratiev D, Pavlova A. Verification of a method for microcoulometric determination of adsorbable organic halide pollutants in natural, drinking, waste, and treated waters. J AOAC Int. 2006;89(3):735–739.
- Borsdorf H, Fiedler P, Wilke D, et al. A simplified analytical procedure for the determination of organically bound halogens in salt-containing water samples. Acta hydrochimica et hydrobiologica. 2003;31(1):19–24.
- Yang X, Zheng Q, He M, et al. Bromine and iodine species in drinking water supply system along the Changjiang River in China: occurrence and transformation. Water Res. 2021;202:117401.
- Hao Z, Shi F, Cao D, et al. Freezing-induced bromate reduction by dissolved organic matter and the formation of organobromine compounds. Environ Sci Technol. 2020;54(3):1668–1676.
- Wang J, Hao Z, Shi F, et al. Characterization of brominated disinfection byproducts formed during the chlorination of aquaculture seawater. Environ Sci Technol. 2018;52(10):5662–5670.
- Fono LJ, Sedlak DL. A simple method for the measurement of organic iodine in wastewater and surface water. Water Res. 2007;41(7):1580–1586.
- Miyake Y, Yamashita N, Rostkowski P, et al. Determination of trace levels of total fluorine in water using combustion ion chromatography for fluorine: a mass balance approach to determine individual perfluorinated chemicals in water. J Chromatogr A. 2007;1143(1–2):98–104.
- Forster ALB, Zhang Y, Westerman DC, et al. Improved total organic fluorine methods for more comprehensive measurement of PFAS in industrial wastewater, river water, and air. Water Res. 2023;235:119859.
- Wegman RCC, Greve PA. The microcoulometric determination of extractable organic halogen in surface water; Application to surface waters of the Netherlands. Sci Total Environ. 1977;7(3):235–245.
- Gilfedder BS, Petri M, Wessels M, et al. Bromine species fluxes from lake constance’s catchment, and a preliminary lake mass balance. Geochim Cosmochim Acta. 2011;75(12):3385–3401.
- Zhang Y, Bu Y, Han J, et al. A facile and green pretreatment method for nonionic total organic halogen (NTOX) analysis in water – step I. Using electrodialysis to separate NTOX and halides. Water Res. 2018;145:631–639.
- Bu Y, Song M, Han J, et al. A facile and green pretreatment method for nonionic total organic halogen (NTOX) analysis in water – step II. Using photolysis to convert NTOX completely into halides. Water Res. 2018;145:579–587.
- Pan H, Huang Y, Li J, et al. Coexisting oxidation and reduction of chloroacetaldehydes in water by UV/VUV irradiation. Water Res. 2022;214:118192.
- Buxton GV, Greenstock CL, Helman WP, et al. Critical review of rate constants for reactions of hydrated electrons, hydrogen atoms and hydroxyl radicals (·OH/·O−)in aqueous solution. J Phys Chem Ref Data. 1988;17(2):513–886.
- Li J, Li B, Pan H, et al. Total organic fluorine (TOF) analysis by completely converting TOF into fluoride with vacuum ultraviolet. J Hazard Mater. 2022;429:128389.
- Du J, Kim K, Min DW, et al. Freeze–thaw cycle-enhanced transformation of iodide to organoiodine compounds in the presence of natural organic matter and Fe (III). Environ Sci Technol. 2022;56(2):1007–1016.