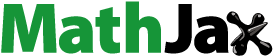
ABSTRACT
UiO-66 (Zr-MOF) metal-organic framework was successfully synthesized by the solvothermal method and characterized using FTIR, PXRD and TGA. Its ability to remove Malathion, 2,4-Dichlorophenoxyacetic acid (2,4-D) and a mixture of the two from an aqueous solution was studied. Effects of different experimental parameters (pH, adsorbent dose, contact time, speed of agitation and initial pesticide concentrations) were studied. Adsorption isotherm study revealed that the equilibrium data well fitted the Freundlich, Langmuir and Jovanovic isotherm models. Similarly, the kinetics study showed that the pseudo-first-order model gave the best fitting. Thermodynamic study indicated that the adsorption process was spontaneous and exothermic. To evaluate the matrix effect, tap water samples were spiked with standard solutions of the analytes and recoveries in the range of 70–120% were obtained. Based on the findings of the present study, UiO-66 (Zr-MOF) could be taken as a promising adsorbent for the removal of pesticides from aqueous samples
1 Introduction
Pesticides when introduced into the environment through spraying on crops, some portion resides in the area where applied and/or transported to various environmental compartments [Citation1,Citation2]. They may also reach streams or groundwater through leaching and adsorbed by plants and eatable vegetables, which are the main sources of pesticide exposure in living organisms. Among the different pesticides, malathion and 2,4-dichlorophenoxyacetic acid (2,4-D) are abundantly used in agricultural, industrial and public health fields [Citation3,Citation4].
Malathion, diethyl 2-[(dimethoxyphosphorothioyl)sulfanyl]butanedioate, is a man-made, broad spectrum and highly toxic organophosphorus insecticide that is commonly used to kill insects, ants, aphids, bagworms, beetles, cotton leaf worm, mosquito, lice and the like by inhibiting cholinesterase enzyme [Citation5]. It is believed that malathion is an agent in causing DNA abnormalities at all doses. There are also reports that it can cause ‘deletions’ in one section of the chromosome, thereby inducing mutations in human beings [Citation6]. More importantly, it should also be noted that malathion can persist in the human body for at least two generations [Citation4]. Literature reports showed higher concentrations of malathion in various water sources. A study done on rivers and surface waters in the Cienega area of Jalisco, Mexico, indicated that the concentrations of Malathion were found to vary between 311.76 and 717.34 μg L−1 in rivers and 771.49–863.49 μg L−1 in surface waters [Citation7]. A similar study in Jimma Zone, Ethiopia, indicated that the concentrations of malathion in river water, spring, tap water, well water and distribution reservoir were found to be 7.658, 7.252, 7.713, 13.947 and 12.165 μg L−1, respectively [Citation8].
Similarly, 2,4-D is a chlorinated phenoxy herbicide, which has been widely used for postemergence control of annual and perennial broad-leaved weeds in cereal croplands, pastures, forests and many other crops because of its low cost and good selectivity. However, it exhibits serious ecological effects [Citation9]. In addition to its toxic effects on birds, beneficial insects, soil annelids and non-target plants, it also negatively impacts aquatic life, affecting algae, small invertebrates, amphibians and fishes, especially in their juvenile stages. Since this compound exhibits high water solubility and mobility, its continuous use may cause soil percolation and groundwater contamination [Citation9,Citation10]. Studies show that different water sources have concentrations of 2,4-D that are the results of anthropogenic sources. The mean concentrations of 2,4-D in river water, spring, tap water, well water and distribution reservoir were found to be 2.666, 2.690, 2.270, 2.939 and 2.354 μg L−1, respectively, in Jimma Zone, Ethiopia[Citation8].
The wide range of pesticides used makes it extremely difficult to produce a single method for their removal from an aqueous system that applies universally. However, several authors have reported a variety of cost-effective and locally available adsorbents such as bagasse fly ash [Citation11], oil shale [Citation12], date palm stones [Citation13] and clay [Citation14,Citation15] for the removal of different types of pesticides from aqueous solutions. Nevertheless, there are some disadvantages associated with these types of adsorbents such as being incompatible with column operation because of their non-granulometric nature that results in chocking of industrial columns, poor adsorption of pesticides at low levels and high regeneration cost. There are also reports of other adsorbents such as activated carbon, chitosan, resin and bentonite [Citation9,Citation15]. However, these adsorbents also have some drawbacks including low adsorption capacity and the requirement of high equilibrium sorbate concentrations that makes them environmentally unfriendly [Citation16,Citation17].
Other techniques such as reverse osmosis, nanofiltration and advanced oxidation processes have some disadvantages due to the formation of undesirable oxidation byproducts and membrane fouling [Citation18]. On the other hand, ion-exchange techniques are eco-friendly and economical, but their low thermal and chemical stability limit their application [Citation19,Citation20].
According to the literature reports, metal-organic frameworks (MOFs) are being used in various applications including adsorption [Citation21], separation [Citation22], catalysis [Citation23] and sensing [Citation24]. They are composed of organic linkers and inorganic nodes and have unusual and exceptional features such as accessible cages, tunnels, high porosity and modifiable pores. One additional advantage of crystalline MOFs is the regular array of well-defined pores, which can achieve ultrahigh porosity and surface area [Citation25,Citation26].
Zr-MOF has gained a lot of attention since it exhibits exceptionally high chemical and thermal stability due to Zr-carboxylate’s strong bond [Citation27]. This has opened a new direction in the application of UiO-66 (Zr-MOF) for adsorptive removal of pollutants from aqueous solutions. In addition to the scarce efforts on the synthesis of this MOF, there are no reports on the utilization of UiO-66 (Zr-MOF) for the adsorptive removal of pesticides including 2,4-D and malathion from environmental water. Therefore, the main purpose of this study was to synthesize and investigate the sorption properties of UiO-66 (Zr-MOF) for 2,4-D and malathion from an aqueous solution.
2 Materials and methods
2.1 Chemicals and reagents
The chemicals and reagents used in this study were all analytical grade and include zirconium oxychloride octahydrate (ZrOCl2.8 H2O, 98%, Avra, India), sodium hydroxide (NaOH, 98%, BDH, England), hydrochloric acid (HCl, 37%, Blulux, India), 2,4-dichloro-phenoxy acetic acid (2,4-D, 97%, Blulux, India), N,N-dimethylformamide (DMF, 99.9%, Loba Chemie, India), methanol (99.9%, Loba Chemie, India), benzene-1,4-dicarboxylic (BDC)/terephthalic acid (98%, Sigma Aldrich, Germany), malathion (50% EC, China) and acetonitrile (99.9%, Park Scientific, UK). Deionized water was prepared in our laboratory.
2.2 Synthesis and characterization of UiO-66 (Zr-MOF)
For the preparation of UiO-66 (Zr-MOF), the solvothermal method reported by Gomes Silva et al. [Citation28] was followed with minor modifications. In brief, 3.900 g of ZrOCl2.8 H2O and 2.001 g of benzene 1,4-dicarboxylic acid were dissolved in 50 mL of DMF and stirred for 30 min on a magnetic stirrer separately. The metal salt solution was added to the organic linker solution slowly and stirred for 24 hr. The reaction continued for additional 24 h in an oven at 120°C and a white precipitate was finally formed. It was then centrifuged at 2500 rpm for 30 min and washed with DMF and methanol several times for solvent exchange and to remove other impurities. The obtained solid material Zr6O4(OH)4[C6H4(CO2)2]12 was dried in open air at room temperature.
The crystallinity and thermal stability of the synthesized UiO-66 (Zr-MOF) were characterized with Powder X-ray diffraction (XRD, Philips Analytical, PW-3040) using Cu-Kα radiation and Thermo gravimetric analyzer (TGA, Shimadzu DTG-60H, South Korea), respectively. The functional group analysis was performed with FT-IR Spectrometer (PerkinElmer, England). Similarly, the surface morphology and elemental composition of the MOF were studied using SEM-EDX (HITACHI Tabletop Microscope TM-1000, Japan).
2.3 Preparation of stock solution and batch adsorption experiments
The stock standard solutions of Malathion and 2,4-D (1000 mg L−1) were prepared in acetonitrile. The respective intermediate and working standards were freshly prepared from the stock solutions by diluting a portion of the stock standard solution with deionized water. Mixture standard solutions containing both analytes were prepared by mixing an equal ratio (v/v) of an intermediate standard solution (100 mg L−1) of each.
The adsorption of malathion and 2,4-D on UiO-66 (Zr-MOF) was studied by batch mode of adsorption. Accordingly, 30 mL of the standard solution containing 5 mg/L of each analyte and a mixture of the two was mixed separately with 0.15 g of the prepared adsorbent under continuous mixing with a magnetic stirrer at room temperature until complete adsorption was assumed to occur. Experimental parameters were optimized by varying one parameter at a time, keeping the others constant, including pH (2–9), contact time (30–180 min), initial concentration (5–50 mg L−1), adsorbent dose (0.04–0.4 g) and agitation speed (50–250 rpm). After equilibrium, the test samples were filtered and the concentration of non-adsorbed analytes in the filtrate was determined using a UV–Vis spectrophotometer at λ values of 294.5 and 291.5 for Malathion and 2,4-D, respectively. Then, the adsorption efficiency of UiO-66 (Zr-MOF) was calculated using equation 1.
where Co and Cf are the initial and final adsorbate concentrations, respectively, in the solution (mg L−1).
2.4 Adsorption isotherm and kinetics study
The adsorption isotherms were investigated by varying the initial concentration of malathion, 2,4-D and the mixture from 5 to 50 mg L−1 while keeping other experimental parameters at their optimum values. To each separate flask, 25 mL of the different initial concentrations of the analytes were mixed with 0.1 g of the synthesized adsorbent. Then, using the adsorption data obtained, different isotherm models including Langmuir, Freundlich and Jovanovic were tested. Similarly, the kinetics of the sorption process was studied by varying the contact time: 30, 60, 90, 120, 150 and 180 min while keeping all other parameters at their optimum values. At the end of the adsorption process, all samples were filtered and analyzed for their respective final concentrations (unadsorbed) using a UV–Vis spectrophotometer. For the kinetics study, Pseudo-first order, Pseudo-second-order and intraparticle diffusion kinetics models were tested and the percent of adsorption was calculated using the following expression.
Percent of adsorption (%) = (2)
where Co and Ce are the initial and equilibrium concentrations of the adsorbate, respectively.
2.5 Thermodynamic studies
To investigate the effect of temperature on the sorption process, all predetermined and optimized values of other parameters (pH, dosage, contact time, speed of agitation and initial concentration) were used and the temperature was varied as 298°C, 308°C, 318°C, 328°C and 338°C. Then, the thermodynamic parameters such as G (free energy change),
H (enthalpy change) and
S (entropy change) were calculated from the temperature effect.
2.6 The pH of point of zero charge (pHPZC)
The pHPZC of UiO-66 (Zr-MOF) was determined by adding 50 mL of NaCl (0.01 M) to 0.5 g of UiO-66 (Zr-MOF), and then the pH was adjusted. After shaking for 48 h, the pH of each flask was measured and the graph was plotted that was used to determine pHPZC from the graph of pHi vs pHf [Citation29].
2.7 Desorption and recyclability
Desorption test was carried out by adding 0.1 g of the adsorbent to 50 mL of the two analytes with an initial concentration of 5 mg/L. The agitation rate and pH were kept constant throughout the desorption experiment. Then, the saturated solutions were centrifuged at 3500 rpm for 20 min and the supernatant was carefully removed. Following this, the residual portions were washed with 10 mL of methanol, acetonitrile, distilled water, 0.1 M NaOH and 0.1 M HCl separately four times each to obtain good desorption yields. The solutions were shaken for 60 min, filtered and analyzed with a UV–Vis spectrophotometer.
For reusability, the used UiO-66 (Zr-MOF) was washed four times with 10 mL of the solvent of choice and air dried and tested for its adsorption efficiency. The regeneration process was performed for 3 cycles.
2.8 Real sample analysis
The applicability of the synthesized MOF was evaluated by collecting a real water sample from two sampling locations, Bate and Haramaya University’s Main Campus. The water samples were treated with an optimized procedure, and the adsorption capacity of the synthesized adsorbent in the presence of matrix effect was determined with a UV–Vis spectrophotometer after filtration.
3 Results and discussion
3.1 Characterization of UiO-66 (Zr-MOF) adsorbent
3.1.1 SEM-EDX study of the adsorbent
Scanning electron microscope image of the synthesized MOF was taken to investigate the morphology of the material. As can be seen from , the adsorbent has an aggregate non-homogenous surface particle size distribution. It is not really possible to propose the morphology of the material as either cubic, tetrahedral or other from the SEM images. This difficulty is due to lower magnification.
3.1.2 FTIR study of the adsorbent
The FTIR spectrum of UiO-66 was used to study the available functional groups on the surface of the adsorbent material (). The broad peaks around 3419 cm−1 show the presence of an OH group at the external surface of the adsorbent. The carboxylate group of the ligand, BDC, has two strongly coupled C−O bonds, which give rise to two sharp peaks, a symmetric C−O stretching band at 1398 cm−1 and an asymmetric C−O stretching band at 1586 cm−1. In addition to the peak existing at 1586 cm−1, the small bands at1504 cm−1 could be assigned to the C=C of the benzene ring of the ligand in the structure. The characteristic peak at 1660 cm−1 can be assigned to the stretching vibrations of C=O in the carboxylic acid present in BDC, and this may also indicate the coordinate bonding of the metal to the organic fraction of terephthalic acid. The peaks at 660 and 748 cm1 are attributed to –O stretching with Zr-(OC). The results from the spectrum are in line with similar reports in the literature [Citation30–32].
Figure 2. FTIR spectra of UiO-66; (a) before adsorption, (b) after adsorption of Malathion and (c) after adsorption of 2,4-D.

There is a slight peak shift around 3400 cm−1 and an increment of the peak intensity was observed after the adsorption of malathion and 2,4-D on the surface of the adsorbent. However, a clear peak spectrum change was not observed on the spectrum of UiO-66 (Zr) before and after the adsorption of both pesticides (). This may imply that surface adsorption is the main adsorption mechanism for both pesticides on the UiO-66 (Zr-MOF). Therefore, the removal mechanism of both pollutants was assumed to be physical adsorption.
3.1.3 PXRD analysis
Powder X-ray diffraction (XRD) is a nondestructive analytical technique in solid state chemistry, and it has been applied for the fingerprint characterization of crystals and for the determination of their structures [Citation33]. The diffraction pattern () of the synthesized material was acquired with Powder X-ray diffraction (XRD, Philips Analytical, PW-3040) equipped with the graphite monochromatized (Ni-filtered) Cu Kα radiation (λ = 1.5406 Å) in 2θ angles ranging from 5°C to 90°C with a step size of 0.05°C and scanning rate of 2°Cper min. It was used to figure out the structural information, crystallinity and phase identification of the material.
As shown in the XRD pattern, the most probable diffraction peaks for the synthesized material were found below a scattering angle (2θ) of 10 degrees, confirming that it is a MOF in good agreement with [Citation1,Citation34]. Intensive peaks appearing at small 2θ angles are characteristics of porous materials, which possess abundant pores or cavities. This may be due to the inverse relation of 2θ and the porosity of the adsorbent [Citation35]. The major peaks observed at 7.46 and 8.12 degrees indicated the MOF character with a symmetric cubic structure and high crystallinity.
3.1.4 TGA analysis
The thermal stability and structural integrity of UiO-66 (Zr-MOF) before and after adsorption of malathion and 2,4-D were further studied by thermogravimetric analysis. For the pristine UiO-66 (Zr-MOF), the thermogravimetric analysis curve () showed two weight loss steps between 25°C and 600°C. The first weight loss of 26.5% below 300°C may be attributed to the release of guest H2O, methanol and surface adsorbed DMF molecules. Upon further heating, a weight loss of 43% between 450°C and 600°C may correspond to the thermal decomposition of the organic linker. The residual weight after heating of UiO-66 (Zr) was 30% at 800℃, which is in good agreement with the theoretical value (25.7%). The final product after 600°C obtained was ZrO2 as can be confirmed from the PXRD above which indicated that there were cubic ZrO2 (JCPDS: 96-152-1754).
Figure 4. TGA of UiO-66 (Zr) before adsorption (a), after adsorption of Malathion (b) and after adsorption of 2,4-D (c).

After adsorption of Malathion and 2,4-D, the weight loss below 200°C increased 29% for Malathion () and 28% for 2,4-D (). This confirms that the adsorption of these pesticides occurred on the surface of UiO-66 (Zr). The stability of UiO-66 (Zr) before and after adsorption of Malathion and 2,4-D is not changed. After heating from 500 to 600℃, a thermal decomposition of the organic linker was observed in all cases.
3.1.5 Determination of pH of point of zero charge (pHPZC)
In a given adsorption process, the chemical properties of the adsorbent play a significant role in determining the surface interactions [Citation36]. One way of understanding this property is by investigating the pHPZC of the adsorbent. It is an essential characteristic that indicates the pH at which the adsorbent does have a neutral surface charge. The adsorbent surface has a net positive surface charge at pH <pHPZC and a net negative surface charge at pH > pHPZC [Citation37]. In the present study, it was observed that the pHPZC of UiO-66 (Zr-MOF) was found to be 4.96 5 () indicating that it has a positively charged surface below its pHPZC where anions could get adsorbed. This indicates that both 2,4-D and Malathion are adsorbed below the pHPZC of UiO-66. This is due to the weak electrostatic attraction between the positive surface of the adsorbent (UiO-66) and anions of 2,4-D and malathion, which favors the accumulation of these pesticides on the surface of the adsorbent.
3.2 Optimization of experimental conditions
3.2.1 The effect of pH
The pH of a solution is an important parameter to be optimized for maximum removal efficiency [Citation38]; because it determines the surface charge of the adsorbent and the degree of ionization of the adsorbate [Citation39]. This means any change in pH leads to variation in the surface properties of the adsorbent and the degree of ionization [Citation18]. In this study, its effect on the adsorption of malathion, 2,4-D and mixture onto UiO-66 was carried out in the pH range from 2 to 9 by keeping other parameters constant.
The high percentage removal of malathion, 2,4-D and the mixture was favored in acidic conditions (). The sorption of malathion and the mixture increased from 76.7% and 70.3% to 99.3% and 88.9% as the pH increased from 2 to 4 and readily decreased to 17.0% and 40.0% from pH 4 to 9. The maximum sorption for both adsorbates was observed at pH 4, which may be due to the electrostatic attraction between anions of the pesticides and the surface of the adsorbent. Since the pHPZC of UiO-66 was nearly at 5, the surface of the adsorbent is positively charged below pHPZC, and, hence, the negatively charged adsorbates (malathion, pKa = −6.8) are attracted to ward the surface of UiO-66. A similar study also shows the favorability of malathion adsorption in acidic media [Citation4].
Figure 6. Effect of experimental parameters on the removal of Malathion, 2,4-D and Mixture by UiO-66 (Zr-MOF): pH (a), adsorbent dose (b), contact time (c), agitation speed (d) and initial pesticide concentration (e).

In the case of adsorption of 2,4-D, the removal efficiency was found to decrease from 99.1% to 31.9% as the pH increased from 3 to 9. At a low pH value, the adsorption removal increased to its highest value, whereas at a high pH value, the removal percentage was intensely decreased. The decrease in adsorption capacity at high pH is explained by the electrostatic repulsion between the surface of adsorbents and the 2,4-D anions because the pKa value of 2,4-D is around 2.7–2.8. Therefore, the favorable adsorption of 2,4-D over UiO-66, especially at a pH of 3, is explained by the electrostatic interaction between the anions of 2,4-D and the positively charged surface of UiO-66. Similar results have been reported in the literature [Citation3].
As the initial pH of the solution increased, the positive charge on the surface decreased and the number of negatively charged sites increased. The negatively charged surface site on the UiO-66 (Zr-MOF) was not favorable for the adsorption of the anionic pesticides due to electrostatic repulsion. However, a slight constant adsorption of 2,4-D was observed at pH 5–6 as can be seen from which might be explained with π−π stacking (between the benzene rings of 2,4-D and UiO-66) on the porous UiO-66, because both the adsorbate and the adsorbent have benzene rings [Citation3]. Therefore, pH values of 3 for 2,4-D and 4 for malathion and the mixture solution were considered the optimum values and were used in the subsequent experiments.
3.2.2 Effect of adsorbent dose
The effect of the adsorbent dose on the removal of malathion, 2,4-D and the mixture using UiO-66 (Zr-MOF) was studied by varying the adsorbent amount in the range of 0.04 to 0.4 g by 0.02 factor at an optimum pH of 4, 3 and 4, respectively, by keeping other parameters (initial concentration, 5 mg/L; contact time, 60 min and agitation speed, 150 rpm) constant. The results shown in suggest that the increase in the dose of adsorbents results in an increase in adsorption efficiency due to the fact that the sorption sites remain unsaturated during the sorption as well as the number of sites available for sorption site increases by increasing the adsorbent dose. However, further increase after 0.1 g did not increase the adsorption, which might be due to the interference between the binding sites of adsorbent doses. The maximum adsorption efficiency of malathion, 2,4-D and the mixture on the UiO-66 (Zr) were 98.0%, 97.1% and 95.1%, respectively, at 0.1 g dose. Hence, the optimal adsorbent dose was considered to be 0.1 g, and this value was used in subsequent experiments.
3.2.3 Effect of contact time
Contact time is a key factor in designing a treatment process with minimum time and maximum efficiency. In the present study, its effect on the removal of malathion, 2,4-D and mixture by UiO-66 (Zr-MOF) was carried out by varying it from 30 to 180 min (). The removals of these pesticides were rapid in the first 30–60 minutes and decreased after 60 min. It was observed that instant adsorption of malathion, 2,4-D and the mixture was achieved within 60 min having adsorption efficiency of 99.6%, 98.2% and 96.3%, respectively. It was noted that the adsorption increased rapidly in the initial stages and then became slow at later stages until the equilibrium was attained. This could be due to the fact that initially all adsorbent sites were vacant, and, hence, the adsorption was high. Later, due to the decrease in the available adsorption sites on the adsorbent surface as well as the decrease in the adsorbate concentration, the adsorption became slow. Similar results have been reported in the literature on the removal of 2,4-D by Cu-Fe-layered double hydroxide nanoparticles [Citation39] and Malathion by Cross-linked polystyrene based strongly basic anion exchange resin De-Acidite FF-IP [Citation40]. Consequently, the optimum contact time for this study was found to be 60 min and was used in the subsequent experiments.
3.2.4 Effect of agitation speed
The influence of agitation speed on the adsorption of malathion, 2,4-D and mixture on UiO-66 (Zr-MOF) from the aqueous solution was studied by varying agitation speed from 50 to 250 rpm by keeping optimum conditions for pH, adsorbent amount and contact time and using the initial concentration constant (5 mg/L). It can be inferred from that the adsorption efficiency increased as the agitation speed increased from 50 to 100 rpm for malathion, 50 to 125 rpm for 2,4-D and 50 to 150 rpm for the mixture with maximum adsorption of 97.6%, 98.1% and 96.7%, respectively. By increasing the agitation speed, the rate of diffusion of the analyte molecules from the bulk liquid to the liquid boundary layer surrounding the adsorbent particle becomes higher because of an enhancement of the turbulence and a decrease in the thickness of the liquid boundary layer [Citation41].
3.2.5 Effect of initial concentration
The effect of initial malathion, 2,4-D and the mixture concentrations on the adsorption phenomena onto the UiO-66 (Zr-MOF) was performed by varying the initial concentrations from 1 to 50 mg/L while other parameters were kept at their optimum values and the results are presented in . The percentage removal of the pesticides was found to decrease with an increase in sorbate concentrations up to a certain concentration and remained almost constant with further increases in concentration indicating the saturation of sorption sites [Citation42]. The percentage removal of malathion, 2,4-D and the mixture decreased from 98.5% to 93.1%, 97.6% to 92.2% and 92.4% to 87.9%, respectively, and then became almost constant as their concentration increased from 20 mg/L to 50 mg/L. The high adsorption rate at the low initial concentration of the pesticides was due to the abundance of free binding sites of the adsorbent. However, at higher concentrations, most of the adsorption sites could be occupied by the adsorbate ions and the available sites for adsorption would become fewer. Therefore, 5 mg/L was considered an optimum initial concentration of the analytes.
3.3 Adsorption isotherms study
The study of adsorption isotherms is essential in ascertaining how solutes interact with adsorbents and understanding the feasibility of different adsorption processes in adsorbing a particular adsorbate. Such a study is also useful in investigating the distribution of adsorbate molecules between liquid and solid phases when the state of equilibrium is achieved [Citation43]. The adsorption isotherms correlate the equilibrium adsorption data with different mathematical models to describe how the adsorbate interacts with adsorbents and is critical in optimizing the use of a given adsorbent [Citation44]. In the present study, an adsorption isotherm study was conducted by varying the initial concentrations of malathion, 2,4-D and the mixture at room temperature by keeping other parameters at optimum conditions and three isotherm models (i.e. Langmuir, Freundlich and Jovanovic) were employed.
The Langmuir isotherm assumes that adsorption occurs at a specific homogeneous site within the adsorbent and that there is no significant interaction among the adsorbed species. In this model, the adsorption rate is proportional to the number of free sites on the adsorbent and fluid concentration [Citation45]. It can be expressed by the following non-linear expression [Citation46].
where Ce is the equilibrium concentration of the solution (mg/L), qe is the quantity of analyte adsorbed, Qo is the maximum adsorption capacity (mg/g) and KL is the sorption equilibrium constant (L/mg), indicating the sorbent affinity and energy of adsorption.
The non-linear plots of the above expression suggest the applicability of the Langmuir isotherm, as indicated by values of R2 and χ2 (). On the other hand, the Freundlich isotherm used for isothermal adsorption is a special case of heterogeneous surface energy in which the energy term varies as a function of surface coverage strictly due to the variation of the sorption [Citation46]. The non-linear expression for this model is given as follows [Citation45]:
Table 1. Comparison of the three adsorption isotherm models for adsorption of Malathion, 2,4-D and their Mixture on UiO-66 (Zr-MOF).
where KF and n are Freundlich constants representing the adsorption capacity and the energy of adsorption, respectively. The values of KF and n obtained from the experimental results are tabulated in . Values of 1/n indicate the favorability of adsorption. As the value of n is greater than unity for all adsorbents, it demonstrates a favorable adsorption condition [Citation4]. The third adsorption isotherm model evaluated in the present study is the Jovanovic isotherm model, which assumes monolayer adsorption without lateral interaction [Citation46]. The mathematical expression of this model is given below in equation 4.
The various values calculated from the respective mathematical expressions of Langmuir, Freundlich and Jovanovic isotherm models for the adsorptive removal of malathion, 2,4-D and mixture of the two using UiO-66 (Zr-MOF) are given in .
The applicability of the three isotherm models to describe the adsorptive removal of malathion, 2,4-D and a mixture of the two was evaluated by using two common parameters (i.e. coefficient of determination (R2) and chi-square (χ2)). The various parameters calculated using the three isotherm models are presented in . Based on the findings of the present study, the adsorptive removal of malathion is best described by the Freundlich isotherm model, which is evidenced by the comparatively higher value of R2 and the smallest value of χ2. The lower value of χ2 in the case of the Freundlich model indicates the less deviation of this isotherm from the experimental data [Citation46]. With similar justification, the adsorption of 2,4-D is best described by the Langmuir model. In the case of the mixture, the highest value of R2 and the smallest value of χ2 were obtained for the Jovanovic isotherm model.
To confirm the favorability of the adsorption process, the separation factor (RL) was determined for each analyte from the Langmuir model and was found to be 0.23, 0.42 and 0.31 for malathion, 2,4-D and the mixture, respectively. The values are between 0 and 1, which confirm that the adsorption process is favorable. Similarly, the values of 1/n for the Freundlich isotherm model indicate a favorable adsorption process. Therefore, with all the differences in the values of R2 and χ2 for the three isotherm models given in , one can conclude that all the three isotherm models can be used to describe the adsorption process of malathion, 2,4-D and the mixture onto the synthesized UiO-66 (Zr-MOF). A similar phenomenon was also reported for the removal of Malathion and 2,4-D [Citation18,Citation47].
3.4 Adsorption kinetics
Kinetics study is essential in the adsorption process that gives an idea of adsorbate uptake rate and efficiency of adsorption. The mechanism of adsorption, on the other hand, depends on the physical and chemical characteristics of the adsorbent as well as the mass transfer process. It provides an understanding of the adsorption rate and the controlling mechanism of the process [Citation48,Citation49]. In the present study, the kinetics profiles of malathion, 2,4-D and mixture removal were generated for the UiO-66 (Zr-MOF) by assessing the equilibrium time to test the suitability of the three common kinetic models, pseudo-first order, pseudo-second order and intraparticle diffusion. The pseudo-first order kinetics may be expressed as follows [Citation4]:
where qt are the amount of adsorbate per unit mass of adsorbent at time t and K1 is the pseudo-first order rate constant. The other common kinetics model is the pseudo-second order that is expressed as follows [Citation46]:
where K2 is the rate constant for adsorption, qe (mg/g) is the amount of adsorbate adsorbed at equilibrium and qt (mg/g) is the amount of adsorbent adsorbed at time t.
Similarly, the intraparticle diffusion model was also applied to describe the adsorption kinetics of the present study that is represented by the following expression:
Values of the different parameters described in the abovementioned three kinetics models including χ2 and coefficient of determination (R2) are presented in .
Table 2. Values of the different parameters of the three kinetics models.
As presented in , the values of R2 and χ2 calculated from the pseudo-first order kinetic model fitted better than the pseudo-second and the intraparticle diffusion kinetics models. This suggests that the adsorption process of malathion, 2,4-D and the mixture of the two onto the synthesized UiO-66 (Zr-MOF) follows first-order kinetics, indicating that the adsorption rate relies on the concentration of adsorbate in the medium rather than the availability of adsorption sites.
3.5 Adsorption thermodynamics
Temperature has an impact on the adsorption capacity to some extent. It is well known that a temperature change alters the adsorption equilibrium in a specific way determined by the exothermic or endothermic nature of the process. In the present study, the effect of temperature was studied in the range 298–338 K to determine the thermodynamic feasibility of the adsorption process. Thermodynamic parameters related to the adsorption process including standard free energy change (G
), standard enthalpy change (
H
) and standard entropy change (
S
) were determined from the slope and intercept in the Van’t Hoff plot of lnKc versus 1/T for the optimized initial concentration of pesticide using the expression given in equations 8–10 [Citation15].
Where:
(8)
G
S
(9)
G
-RT
(10)
where Kc is the thermodynamic equilibrium constant, qe is the equilibrium adsorption capacity of the adsorbent at different temperatures, Ce is the equilibrium concentration of adsorbate in the solution, T (K) is the absolute temperature and R (8.314 J mol−1 K−1) is the universal gas constant. The calculated thermodynamic parameters from the plot of lnKc versus 1/T are shown in .
Table 3. Thermodynamic parameters for 2,4-D and malathion adsorption onto UiO-66 (Zr).
Thermodynamically spontaneous and nonspontaneous processes are indicated by negative and positive G
changes, respectively. Therefore, as summarized in , the negative values of
G
at all temperatures studied indicated that the adsorption processes were spontaneous and feasible. For both 2,4-D and malathion, the values of
H
were negative, indicating the exothermic nature of the process, which further explains the fact that the adsorption efficiency decreased with the increase in temperature. For 2,4-D, the exothermic adsorption may be due to a weaker interaction between the pre-adsorbed water and the adsorbent than the interaction between 2,4-D (actually the anionic or deprotonated form) and the adsorbent. This may show a relatively strong electrostatic interaction between the 2,4-D anions and the positive surface of UiO-66 (Zr-MOF) adsorbent relatively at low temperatures. Similar results were reported for adsorption of 2,4-D on MIL-53 (MIL stands for Material of Institute Lavoisier) (Cr-BDC) and banana stalk activated carbon [Citation3,Citation15].
The thermodynamic calculation for malathion was also negative, which reveals that the mechanism of malathion adsorption from the aqueous solution was feasible and spontaneous. The negative value of H
indicates an exothermic adsorption process with a strong adsorbate-adsorbent electrostatic attraction [Citation4]. The magnitude of
H
may also give an idea about the type of sorption (physical or chemical) and generally, the
H
value ranges from 0 to 40 KJ/mol for physisorption and 40–120 KJ/mol for chemisorption mechanisms [Citation50]. In the present investigation, the
H
values were found to be −17.23 and −19.51 KJ/mol for 2,4-D and malathion, respectively, and this indicates that physisorption is the major adsorption mechanism. The physisorption mechanism inferred is supported by the findings from the isotherm study that indicated multilayer adsorption. Additionally, the negative value of ∆S implies less randomness at the solid/solution interfaces during the adsorption process.
3.6 Desorption and reusability
To keep the processing cost as low as possible, it is very important to carry out desorption and regeneration studies. The desorption study of malathion and 2,4-D was carried out by the batch processes using different desorbing reagents (methanol, acetonitrile, distilled water, 0.1 M NaOH and 0.1 M HCl). The results of desorption obtained by methanol, acetonitrile, distilled water, 0.1 M NaOH and 0.1 M HCl solutions are shown in . Relatively, the best result was achieved by acetonitrile with desorption of 75% and 85% of malathion and 2,4-D, respectively. This outcome could be due to the high solubility of both adsorbates in the reagent. The desorption efficiency of the analytes in other solvents was found to be 69% and 78% in methanol, 65% and 71% in deionized water, 53% and 56% in 0.1 M NaOH and 46% and 45% in 0.1 M HCl for malathion and 2,4-D, respectively. Even though desorption of adsorbate from the surface of the adsorbent was possible, high desorption efficiency was not possible in these solvents compared to acetonitrile. This might be due to the strong electrostatic attraction between the surface of the adsorbent and adsorbates.
To keep the adsorption process more economical, it is advantageous to regenerate the adsorbent. Since commercial applications of adsorptive removal rely on the reusability of an adsorbent, adsorption will surely be improved greatly if the reusability of the adsorbents is confirmed [Citation3]. In this study, the reusability of the UiO-66 (Zr-MOF) was estimated after washing the used MOF with acetonitrile. The results of malathion and 2,4-D sorption using original and regenerated UiO-66 (Zr-MOF) are shown in . Accordingly, UiO-66 retained about 70% adsorption capacity after three runs. This implies that the spent adsorbent (UiO-66) can be regenerated and reused. The percent desorption decreased from 80% to 68% and 85% to 72% for malathion and 2,4-D, respectively, for the second and third cycles. This reveals that UiO-66 can be regenerated and reused multiple times, as suggested by Lin et al. [Citation51].
3.7 Real sample analysis
To ensure the applicability of the developed method for the removal of pesticides from real samples, tap water samples from Bate Kebele and Haramaya University Main Campus were treated with UiO-66 (Zr) at optimized conditions. In the assayed water samples, the target analytes (Malathion and 2,4-D) were not detected. Therefore, the samples were spiked with a known standard solution to evaluate the adsorption performance of UiO-66 (Zr) in the presence of matrix effect. The results listed in show the recovery values for the adsorption of malathion and 2,4-D in the two tap water samples. The obtained recovery values were in the ranges of 78–107% and 90–111% at 5 and 10 mg/L spiking levels for malathion and 2,4-D, respectively. The results met the criteria for the matrix effect (70–120%) according to the guidance document on analytical quality control and validation procedures for pesticide analysis in water [Citation52]. Based on the recovery values, it can be said that the matrix effect of the water samples on the adsorption of malathion and 2 4-D onto UiO-66 (Zr) was not significant.
Table 4. Adsorption efficiency of UiO-66 (Zr) for the two analytes in real water samples.
4 Conclusion
Zirconium-based metal-organic framework (UiO-66) was successfully prepared by the solvothermal method using DMF as an organic linker at 120°C and characterized by FTIR, PXRD and TGA for functional groups, phase identification and thermal stability, respectively. The performance of UiO-66 (Zr) for the adsorptive removal of malathion, 2,4-D and their mixtures was studied in batch mode by optimizing different experimental parameters. Adsorption isotherm, kinetics and thermodynamics investigations were also done. Based on the results obtained, it can be concluded that UiO-66 (Zr) was found to be promising and, therefore, be taken as an alternative method in the literature for the adsorptive removal of malathion, 2,4-D and their mixture from an aqueous solution. The authors would like to recommend future research undertakings on these organic-inorganic hybrid solid materials with infinite, uniform framework structures built from organic linkers and inorganic metal (or metal containing cluster) nodes for the adsorptive removal of other pesticide residues from aqueous samples.
Acknowledgments
The authors are very much grateful for the support of the Department of Chemistry, College of Natural and Computational Sciences, Haramaya University, Ethiopia.
Disclosure statement
No potential conflict of interest was reported by the author(s).
Data availability statement
No additional data have been used to support the findings of this study.
References
- Zhang KD, Tsai FC, Ma N, et al. Adsorption behavior of high stable Zr-based MOFs for the removal of acid organic dye from water. Materials. 2017;10(2):10. doi: 10.3390/ma10020205.
- Teju E, Tadesse B, Megersa N. Salting-out-assisted liquid–liquid extraction for the preconcentration and quantitative determination of eight herbicide residues simultaneously in different water samples with high-performance liquid chromatography. Sep Sci Technol. 2021;56(4):719–293. doi: 10.1080/01496395.2016.1276596.
- Jung BK, Hasan Z, Jhung SH. Adsorptive removal of 2,4-dichlorophenoxyacetic acid (2,4-D) from water with a metal–organic framework. Chem Eng J. 2013;234:99–105. doi: 10.1016/j.cej.2013.08.110
- Kumar P, Singh H, Kapur M, et al. Comparative study of malathion removal from aqueous solution by agricultural and commercial adsorbents. Water Proc Eng. 2014;3:67–73. doi: 10.1016/j.jwpe.2014.05.010
- Venugopal N, Sumalatha B. Spectrophotometric determination of malathion in environmental samples. E-J Chem. 2012;9(2):857–862. doi: 10.1155/2012/390517.
- Nair AS, Pradeep T. Extraction of chlorpyrifos and malathion from water by metal nanoparticles. J Nanosci Nanotechnol. 2007;7(6):1871–1877. doi: 10.1166/jnn.2007.733.
- Silva-Madera RJ, Salazar-Flores J, Peregrina-Lucano AA, et al. Pesticide contamination in drinking and surface water in the Cienega, Jalisco, Mexico. Water Air Soil Pollut. 2021;232(2):43. doi: 10.1007/s11270-021-04990-y.
- Mekonen S, Argaw R, Simanesew A, et al. Pesticide residues in drinking water and associated risk to consumers in Ethiopia. Chemosphere. 2016;162:252–260. doi: 10.1016/j.chemosphere.2016.07.096
- Dehghani M, Nasseri S, Karamimanesh M. Removal of 2,4-Dichlorophenolyxacetic acid (2,4-D) herbicide in the aqueous phase using modified granular activated carbon. J Environ Health Sci Eng. 2014;12(1):28–35. doi: 10.1186/2052-336X-12-28.
- Sandoval-Carrasco CA, Ahuatzi-Chacón D, Galíndez-Mayer J, et al. Biodegradation of a mixture of the herbicides ametryn, and 2,4-dichlorophenoxyacetic acid (2,4-D) in a compartmentalized biofilm reactor. Biores Technol. 2013;145:33–36. doi: 10.1016/j.biortech.2013.02.068
- Gupta VK, Jain CK, Ali I, et al. Removal of lindane and malathion from wastewater using bagasse fly ash—a sugar industry waste. Water Res. 2002;36(10):2483–2490. doi: 10.1016/S0043-1354(01)00474-2.
- Al-Qodah Z, Shawaqfeh AT, Lafi WK. Adsorption of pesticides from aqueous solutions using oil shale ash. Desalination. 2007;208(1–3):294–305. doi: 10.1016/j.desal.2006.06.019.
- Hameed BH, Salman JM, Ahmad AL. Adsorption isotherm and kinetic modeling of 2,4-D pesticide on activated carbon derived from date stones. J Hazard Mater. 2009;163(1):121–126. doi: 10.1016/j.jhazmat.2008.06.069.
- Pal OR, Vanjara AK. Removal of malathion and butachlor from aqueous solution by clays and organoclays. Sep Purif Technol. 2001;24(1–2):167–172. doi: 10.1016/S1383-5866(00)00226-4.
- Salman JM, Njoku VO, Hameed BH. Adsorption of pesticides from aqueous solution onto banana stalk activated carbon. Chem Eng J. 2011;174(1):41–48. doi: 10.1016/j.cej.2011.08.026.
- El Harmoudi H, El Gaini L, Daoudi E, et al. Removal of 2,4-D from aqueous solutions by adsorption processes using two biopolymers: chitin and chitosan and their optical properties. Opt Mater. 2014;36(9):1471–1477. doi: 10.1016/j.optmat.2014.03.040
- Kearns JP, Wellborn LS, Summers RS, et al. 2,4-D adsorption to biochars: effect of preparation conditions on equilibrium adsorption capacity and comparison with commercial activated carbon literature data. Water Res. 2014;62:20–28. doi: 10.1016/j.watres.2014.05.023
- Naushad M, Alothman ZA, Khan MR. Removal of malathion from aqueous solution using De-Acidite FF-IP resin and determination by UPLC–MS/MS: equilibrium, kinetics and thermodynamics studies. Talanta. 2013;115:15–23. doi: 10.1016/j.talanta.2013.04.015
- Zhang S, Zeng M, Li J, et al. Porous magnetic carbon sheets from biomass as an adsorbent for the fast removal of organic pollutants from aqueous solution. J Mater Chem A. 2014;2(12):4391–4397. doi: 10.1039/C3TA14604A.
- Chen T, Zhang C, Qin Y, et al. Preparation of Cationic MOFs with mobile anions by anion stripping to remove 2,4-D from water. Materials. 2017;10(8):10. doi: 10.3390/ma10080879.
- Rostami S, Nakhaei Pour A, Salimi A, et al. Hydrogen adsorption in metal- organic frameworks (MOFs): effects of adsorbent architecture. Int J Hydrogen Energy. 2018;43(14):7072–7080. doi: 10.1016/j.ijhydene.2018.02.160.
- Zhang J, Chen Z. Metal-organic frameworks as stationary phase for application in chromatographic separation. J Chromatogr A. 2017;1530:1–18. doi: 10.1016/j.chroma.2017.10.065
- Zhang Y, Yang X, Zhou H-C. Synthesis of MOFs for heterogeneous catalysis via linker design. Polyhedron. 2018;154:189–201. doi: 10.1016/j.poly.2018.07.021
- Li C, Huang J, Zhu H, et al. Dual-emitting fluorescence of Eu/Zr-MOF for ratiometric sensing formaldehyde. Sensors And Actuat B Chem. 2017;253:275–282. doi: 10.1016/j.snb.2017.06.064
- Farha OK, Özgür Yazaydın A, Eryazici I, et al. De Novo synthesis of a metal–organic framework material featuring ultrahigh surface area and gas storage capacities. Nat Chem. 2010;2(11):944–948. doi: 10.1038/nchem.834.
- Furukawa H, Ko N, Go YB, et al. Ultrahigh Porosity in metal-organic frameworks. Science. 2010;329(5990):424–428. doi: 10.1126/science.1192160.
- He Y, Tang YP, Ma D, et al. UiO-66 incorporated thin-film nanocomposite membranes for efficient selenium and arsenic removal. J Membr Sci. 2017;541:262–270. doi: 10.1016/j.memsci.2017.06.061
- Gomes Silva C, Luz I, Llabres i Xamena FX, et al. Water stable Zr-Benzenedicarboxylate Metal-Organic frameworks as Photocatalysts for hydrogen generation. Chem: Eur J. 2010;16(36):11133–11138. doi: 10.1002/chem.200903526.
- Jing H-P, Wang C-C, Zhang Y-W, et al. Photocatalytic degradation of methylene blue in ZIF-8. RSC Adv. 2014;4(97):54454–54462. doi: 10.1039/C4RA08820D
- Valenzano L, Civalleri B, Chavan S, et al. Disclosing the complex structure of UiO-66 metal organic framework: a synergic combination of experiment and theory. Chem Mater. 2011;23(7):1700–1718. doi: 10.1021/cm1022882.
- Azhar MR, Abid HR, Sun H, et al. One-pot synthesis of binary metal organic frameworks (HKUST-1 and UiO-66) for enhanced adsorptive removal of water contaminants. J Colloid Interface Sci. 2017;490:685–694. doi: 10.1016/j.jcis.2016.11.100
- Ma D, Peh SB, Han G, et al. Thin-Film Nanocomposite (TFN) membranes incorporated with super-Hydrophilic Metal–Organic Framework (MOF) UiO-66: toward enhancement of water flux and salt rejection. ACS Appl Mater Inter. 2017;9(8):7523–7534. doi: 10.1021/acsami.6b14223
- Bunaciu AA, Udriştioiu EG, Aboul-Enein HY. X-ray diffraction: instrumentation and applications. Crit Rev Anal Chem. 2015;45(4):289–299. doi: 10.1080/10408347.2014.949616.
- Kim S-N, Lee Y-R, Hong S-H, et al. Pilot-scale synthesis of a zirconium-benzenedicarboxylate UiO-66 for CO2 adsorption and catalysis. CatalToday. 2015;245:54–60. doi: 10.1016/j.cattod.2014.05.041
- Li J-R, Sculley J, Zhou H-C. Metal–Organic Frameworks for separations. Chem Rev. 2012;112(2):869–932. doi: 10.1021/cr200190s.
- Bedadeep D, Shahnaz T, Manu Sankar V, et al. Organic polymer doped graphene-based composite for the effective elimination of diclofenac: a detailed study with phytotoxic assessments. J Environ Chem Eng. 2023;11(1):109223. doi: 10.1016/j.jece.2022.109223
- Azhar MR, Abid HR, Periasamy V, et al. Adsorptive removal of antibiotic sulfonamide by UiO-66 and ZIF-67 for wastewater treatment. J Colloid Interface Sci. 2017;500:88–95. doi: 10.1016/j.jcis.2017.04.001
- Priyan, V. V., Kumar N, Rajendran HK, et al. Sequestration and toxicological assessment of emerging contaminants with polypyrrole modified carboxymethyl cellulose (CMC/PPY): case of ibuprofen pharmaceutical drug. Int j biol macromol. 2022;221:547–557. doi: 10.1016/j.ijbiomac.2022.09.046
- Nejati K, Davary S, Saati M. Study of 2,4-dichlorophenoxyacetic acid (2,4-D) removal by Cu-Fe-layered double hydroxide from aqueous solution. Appl Surface Sci. 2013;280:67–73. doi: 10.1016/j.apsusc.2013.04.086
- Dehghani MH, Niasar ZS, Mehrnia MR, et al. Optimizing the removal of organophosphorus pesticide malathion from water using multi-walled carbon nanotubes. Chem Eng J. 2017;310:22–32. doi: 10.1016/j.cej.2016.10.057
- Kuśmierek K, Świątkowski A. The influence of different agitation techniques on the adsorption kinetics of 4-chlorophenol on granular activated carbon, reaction kinetics. Reac Kinet Mech Cat. 2015;116(1):261–271. doi: 10.1007/s11144-015-0889-1.
- Alka T, Anita B. Effective removal of pesticide (dichlorvos) by adsorption onto super paramagnetic poly (styrene-co-acrylic acid) hydrogel from water. Int Res J Environ Sci. 2014;3:41–46.
- Wanjeri VWO, Sheppard CJ, Prinsloo ARE, et al. Isotherm and kinetic investigations on the adsorption of organophosphorus pesticides on graphene oxide based silica coated magnetic nanoparticles functionalized with 2-phenylethylamine. J Environ Chem Eng. 2018;6(1):1333–1346. doi: 10.1016/j.jece.2018.01.064
- Bakhtiary S, Shirvani M, Shariatmadari H. Adsorption–desorption behavior of 2,4-D on NCP-modified bentonite and zeolite: implications for slow-release herbicide formulations. Chemosphere. 2013;90(2):699–705. doi: 10.1016/j.chemosphere.2012.09.052.
- Chen C, Zhang M, Guan Q, et al. Kinetic and thermodynamic studies on the adsorption of xylenol orange onto MIL-101(Cr. Chem Eng J. 2012;183:60–67. doi: 10.1016/j.cej.2011.12.021
- Chandrasekar R, Rajendran HK, Priyan V, et al. Valorization of sawdust by mineral acid assisted hydrothermal carbonization for the adsorptive removal of bisphenol A: a greener approach. Chemosphere. 2022;303:135171. doi: 10.1016/j.chemosphere.2022.135171
- Evy Alice Abigail M, Chidambaram R. Rice husk as a low cost nanosorbent for 2,4-dichlorophenoxyacetic acid removal from aqueous solutions. Ecol Eng. 2016;92:97–105. doi: 10.1016/j.ecoleng.2016.03.020
- Muthukumaran C, Sivakumar VM, Thirumarimurugan M. Adsorption isotherms and kinetic studies of crystal violet dye removal from aqueous solution using surfactant modified magnetic nanoadsorbent. J Taiwan Inst Chem Eng. 2016;63:354–362. doi: 10.1016/j.jtice.2016.03.034
- Patra C, Narayanasamy S. Polypyrrole complexation on biomass-derived powdered carbon for adsorptive elimination of emerging pharmaceutical contaminant Sulfamethoxazole: a comprehensive insight. J Clean Prod. 2022;370:133565. doi: 10.1016/j.jclepro.2022.133565
- Fairooz NE, Jwad ZA, Zahra M. Adsorption isotherms and thermodynamic data for removal pesticides from aqueous solution on pomegranate peel surface. Am J Appl Chem. 2015;3(4):147–152. doi: 10.11648/j.ajac.20150304.11.
- Lin K-YA, Chen S-Y, Jochems AP. Zirconium-based metal organic frameworks: highly selective adsorbents for removal of phosphate from water and urine. Materials Chemistry & Physics. 2015;160:168–176. doi: 10.1016/j.matchemphys.2015.04.021
- Pizzutti IR, Dias JV, Kok AD, et al. Pesticide residues method validation by UPLC-MS/MS for accreditation purposes. J Braz Chem Soc. 2016;27:1165–1176. doi: 10.5935/0103-5053.20160012