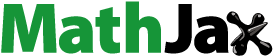
ABSTRACT
Natural and anthropogenic event information helped to simulate the physical–chemical behavior of hydrogen sulfide (H2S) in a deep oil spill and a hypothetical deep-ocean hydrothermal discharge. This research led to the development of the Lagrangian model of the discharge plumes in both cases and also analyzed the profiles of the H2S concentration at different depths taking into account its dissolution in ocean water and oceanographic conditions, such as thermohaline stratification, current fields, as well as the characteristics of the spilled hydrocarbon and the hydrothermal. The results revealed a first approximation in the identification of the dynamic behavior of the concentration profiles of H2S as a function of the oceanographic conditions. Hydrogen sulfide concentrations vary between natural and anthropogenic discharges at various depths, leading to a numerical model for applying in chemical oceanography.
1. Introduction
Natural events incorporate also many chemical compounds in the oceans, which are present in different forms and/or concentrations. As a result of the natural regulation of the physical, chemical, biological and geological equilibrium, there are responses to the natural and anthropogenic sources that disturb our oceans [Citation1,Citation2].
Sulfur is one of those compounds that enters the ocean by different routes. It is the sixth most abundant element on Earth [Citation3]. The most common oxidation states of sulfur range from −2 to + 6, which determines its high reactivity and allows a wide range of marine microorganisms to incorporate it into their cellular components and use it for energy transformations [Citation4,Citation5]. This ability to utilize sulfur as an energy source demonstrates the versatility and adaptability of marine microorganisms in environments where sulfur is present in different chemical forms and oxidation states [Citation6].
Sulfur is present in various chemical compounds, such as pyrite and other inorganic compounds like sulfur dioxide
and hydrogen sulfide
. It can also exist as sulfite ions (
) and hydrosulfide ions
in aqueous solutions.
Sulfur is present in natural gas, and crude oil in the form of hydrogen sulfide forming various compounds: thiols, mercaptans, sulfides, polysulfides, etc. or as elemental sulfur. Crude oil containing noticeable amounts of hydrogen sulfide or other reactive sulfur compounds is ´sour´; and those with lower sulfur content are ´sweet´.
Two factors help fix salinity in the ocean. The ratio of the elements such as hydrogen, chloride and sulfur in the volatile emissions from the interior of the earth, and the tectonic factors that influence the division of sulfur and chlorine between the ocean and the sediments [Citation7]. Seawater’s average sulfate ion () concentration is near 30
. The response time of
dissolved in the sea is 20 million years [Citation7].
Chemical pollution resulting from human activities exemplifies the detrimental effects of human influence on the environment, including the oceans. Certain oceanic activities can generate bulky amounts of pollutants that can disrupt natural cycles. When analyzing anthropogenic contamination resulting from deep oil spills, it is crucial to consider not only organic components but also other chemical compounds present in the spill, such as H2S. Some exceptions to this rule are ”West Texas” crudes (which are always ´sour´ regardless of their H2S content) and highly sulfurous Arabian crudes (which are not ´sour´ because their sulfur compounds are not very reactive).
Assessing the anthropogenic impact of these types of pollutants in deep oil spill conditions necessitates the collection of field data from a historical perspective. In addition to that, a multidisciplinary scientific approach is required for accurate interpretation.
The focus of this research is to compare the anomalies in the marine environment caused by diluted H2S originating from anthropogenic and natural sources. It is understood that natural sources o H2S would not be considered contaminants and can serve as a baseline for evaluating the impact of substances that cannot be detected through remote sensing. This analysis will contribute to a comprehensive understanding of the potential contamination and aid in identifying and mitigating the impacts of anthropogenic pollutants on the marine ecosystem. By comparing the dissolution behavior of H2S from different sources, this study aims to shed light on the concentration variations of this substance under deep-ocean environmental conditions. It takes into account various oceanographic factors, including stratification, slope gradients, thermohaline fields and physical characteristics of the discharge sources, to understand their influence on the dissolution process. Consequently, this research contributes to the development of numerical models that facilitates the assessment of potential pollution sources and their fate under specific oceanographic conditions [Citation8].
By incorporating the principles of physical and chemical oceanography into numerical models, researchers can gain insights into the dissolution dynamics of substances like H2S in seawater [Citation9,Citation10]. This knowledge contributes to a better understanding of the behavior of pollutants in marine environments, facilitating the assessment of potential ecological and health impacts associated with natural and anthropogenic discharges [Citation11,Citation12].
The representation of the transport phenomena in the ocean adjusted to the space-time scale in which the Deepwater Horizon (DWH) spill took place in June 2010, with a geographical position of the platform of N,
W [Citation13].
depicts the locations of the anthropogenic source and the hypothetical natural source. The square represents the domain research for both discharges. Additionally, the bathymetry of the domain where hydrodynamic and dissolution modeling took place is illustrated. The model domain has a resolution of
and is centered on the DWH spill site. The study conducted by Reddy et al. [Citation14] on the behavior of the soluble portion was incorporated into this research. According to the methodology employed, the potential chemical reactions involving H2S and the formation of methane hydrates were not taken into account [Citation13].
This research included the modeling of the anthropogenic and natural discharges with a plume of variable density closed a more realistic response to the physical distribution of the spills and their compounds.
A state-of-the-art review carried out found there are no publications about deep-ocean release activities by H2S contamination.
2. Methods
The present research work utilizes deterministic investigation to solve numerical methods applied to hydrodynamic and physicochemical processes. This research methodology is partly suitable for the case study due to several reasons. First, it allows for the simulation of hydrodynamic conditions using already validated models, which simplifies the process. Second, it proves to be an efficient research method compared to in situ measurements, especially in challenging scenarios where significant investments would be required. Additionally, the hydrodynamic model demonstrates adaptability to various conditions. Lastly, this approach enables us to expand our capabilities in solving complex and realistic problems [Citation15].
In this manuscript, a hydrodynamic model was used to simulate the Eulerian fields of the dissolution in the water column of natural and anthropogenic hydrogen sulfide.
Both the natural and anthropogenic discharges exhibit stages driven by both inertia and buoyancy in the mixing process. The duration and extent of each stage depend on the specific chemical characteristics of the discharge as well as environmental parameters such as temperature, salinity and currents [Citation11]. These factors play a crucial role in determining the time and spatial distribution of the mixture, ultimately influencing the dispersion and behavior of the dissolved substances in the seawater [Citation16].
In this sense, they corresponded to the trajectories of the Lagrangian plumes of the natural discharge of a deep hydrothermal and the accidental spill of oil from a blowout. In other words, the work analyzed how the concentration of the sulfur ion in a volume of ocean water changes in two different cases, keeping constant the conditions of the affected area, such as thermohaline stratification, current fields, sea-level topography and the characteristics of the spilled fluid. To simulate the behavior of solubilized hydrogen sulfide in both cases, it was necessary to know the Lagrangian behavior of the 3D plume path for both the hydrothermal and the spilled oil.
EquationEquation (1)(1)
(1) of hydrogen sulfide partial ionization can generally be summarized as
EquationEquation (1)(1)
(1) describes the partial ionization of hydrogen sulfide. It represents the equilibrium between hydrogen sulfide (H2S) and its dissociated forms, the hydrogen ion (
) and the sulfide ion (
). This equilibrium equation illustrates the dynamic interplay between H2S/day and its ions in aqueous environments, particularly in the ocean. Understanding this partial ionization process is essential for studying the distribution, transport and behavior of hydrogen sulfide in marine ecosystems.
Measurements for different flow regimes of deep-ocean hydrothermal, including diffuse at low and high temperatures (black smoker hydrothermal fluids) showed temperatures up to C and velocities ranging from 3.9 to 35.5 cm/s. Fluid discharge estimates for the hydrothermal plants ranged from 3.5 to
[Citation17].
The sulfur content of crude oil varies from to
; it is pure sulfur, hydrogen sulfide (H2S) and organic sulfur compounds. The components in liquid oils that contain nitrogen, sulfur and oxygen are known as NSO compounds [Citation18]. The sulfur content of the Fields in Mexico (Isthmus) has volumetric concentrations of 1.6% [Citation19].
A deep-ocean black smoker hydrothermal vent emits particle-laden fluids. The particles are mainly fine-grained sulfide minerals that form when hot hydrothermal fluids mix with nearly frozen minerals. These minerals solidify as they get cold, forming chimney-like structures https://oceanservice.noaa.gov/facts/vents.html.
In both Lagrangian models, each particle in the plume was defined in a spherical shape, characterized by its location in 3D motion. Recent developments display a methodology for calculating oil droplet size distributions [Citation20]. Studies have shown a comparison between computational and experimental distribution for droplet size [Citation21].
Yapa et al. [Citation22] stated that typical droplet sizes, determined through experimental methods, exhibit a predominant distribution with diameters of 2, 3 and 4 mm. However, the presence of chemical dispersants alters this distribution, leading to a prevailing diameter range of 600, 700 and 800 µm.
In order to build of the initial conditions of potential temperature (T) in Celsius degrees and salinity (S) in Practical Salinity Units in the space and time domain, an adjustment was made to the ECOMSED code to enter the depth levels [Citation15].
represents the surface temperature field (°C) on 1 May 2010, provided by Copernicus [Citation23]. The data collected between 1 and 6 May 2010 reveal that the temperature variable shows minimal temporal variability across different depths. This suggests that there were no significant changes in temperature during this time period, indicating a stable thermal environment.
represents the salinity field (Practical Salinity Units) on 1 May 2010 also provided by Copernicus [Citation23]. Similar to the temperature data, the salinity measurements taken between 1 and 6 May 2010 exhibit low temporal variability in different depth conditions. This indicates a relatively constant salinity distribution during that period.
represent the current fields (m/s)) on 1 May 2010, provided by Copernicus [Citation23].
Assuming that the plume dynamics do not include the physical–chemical processes associated with gas hydrate formation and dissolution [Citation24]. in Appendix A, shows the characteristics of hydrocarbons introduced into the 3D hydrodynamic model [Citation15].
The cell volumes in the Eulerian calculation grid defined fixed control volumes around each drop of the Lagrangian plume. The flow rate discharge in each model (natural and anthropogenic) are defined as constants; other parameters were calculated as variables since they change according to the diameter of the droplets. For example, the mass transfer coefficient had to be calculated for each condition to be analyzed [Citation25].
A hydrothermal flow temperature (T) at the discharge of 300.85°C and pressure (P) at 130 bars was assumed. With these conditions of T and P, the state of the mixture according to the triple point of the water would be a liquid state [Citation25].
The reviewed literature allowed us to find references for both calculated and sampled densities of fluids from hydrothermal sources. Thus, reference values of density lower than that of the ambient bottom water,
at
C and 2,000 m deep, are found. Black smokers exhibit the highest density contrast (approximately
–
), resulting in floating hydrothermal plumes that rise well above the sea floor [Citation26,Citation27].
Concentrations of hydrogen sulfide in stable samples from deep hydrothermal (black smoke) discharges in The Indian Ocean at 2,450 m depth are on the order of 4.0 mM [Citation28]. in Appendix A, summaries data references for hydrothermal simulations [Citation27].
In the present modeling, as a first approximation, the solubility constant of H2S at different standard oceanographic levels is fixed at 1.013 bar and , with a value of 4.67
[Citation29].
Studies on particle size distribution in deep hydrothermal discharges have not shown diameters in a solid state more than 100 µm. The distribution of slow-settling particles, which in the end, are those of interest for this research, has an average size between 1 and 10 µm [Citation30]. Due to the wetting effect of the aqueous solution on the solid particles, an increase in diameter of up to 10 times the particle diameter was considered.
Non-hydrocarbon petroleum components include organic derivatives of nitrogen, oxygen, sulfur and the metals: nickel and vanadium [Citation31]. A proportion of these impurities will remain in the water column after a deep spill.
Total sulfur in crude oil can vary from for light oil to approximately
for heavy oil. However, the sulfur content of crude oil will also depend on the geographical regions of extraction due to the geochemical formation conditions [Citation30].
A sample of the crude oil from the DWH at the time of the spill had a sulfur content of [Citation14].
The rate of change of hydrogen sulfide mass within the droplets, resulting from its dissolution in the water column, is computed by the following equation proposed by Zheng et al. [Citation32]:
EquationEquation (2)(2)
(2) describes the dynamics of the hydrogen sulfide mass with respect to time. It involves parameters such as the mass transfer coefficient (K), the solubility of hydrogen sulfide (S) and the concentration of dissolved hydrogen sulfide in the environment (
). By utilizing EquationEquation (2)
(2)
(2) , we can gain insights into the dissolution process and its impact on the behavior of hydrogen sulfide in the water column.
Herein, t is time (s); n is the number of moles of a solubilized component; K is the mass transfer coefficient for hydrogen sulfide (); A is the total surface area of each of the drops in the liquid phase
; S is the solubility of hydrogen sulfide
and
is the concentration of dissolved hydrogen sulfide in the environment
. Then, it was assumed that
in (2) is negligible
S).
In the context of both anthropogenic and natural discharge conditions, the effective internal coefficient, commonly employed in heat transfer analysis of solid spheres [Citation25], can be defined as
This coefficient, derived from heat transfer analysis, extends its applicability to characterize the mass transfer process. It represents the ratio between the diffusion coefficient inside the droplet () and the droplet diameter (
), scaled by a factor of 10. Incorporating this coefficient provides valuable insights into the complex interplay between heat and mass transfer phenomena associated with the discharge, facilitating a comprehensive analysis of the system’s behavior.
The transport of substances in oceanic environments is influenced by various factors, including currents, turbulent diffusion processes and the phase change of pollutants, such as solubility. These factors are incorporated into the following transport Equationequation (4)(4)
(4) :
This comprehensive transport equation captures the dynamic interplay of advection, diffusion and internal sources, providing a valuable framework for studying the distribution and behavior of pollutants in oceanic environments. In this equation, C represents the concentration of the transported pollutant, varying with time (t) and space coordinates (x, y, z), where U, V and W denote the respective components of current velocity. is the vertical diffusivity. The term Q represents the internal source resulting from the dilution of hydrogen sulfide components
, while
represents the horizontal diffusion terms. By considering advection, diffusion and internal sources, this equation accounts for the complex processes involved in the transport of substances and allows for a comprehensive analysis of their distribution and behavior in the oceanic environment.
In the given case, Q is the accumulation rate of hydrogen sulfide from the Lagrangian feathers. In the given scenario, Q corresponds to the rate of accumulation of hydrogen sulfide from the Lagrangian feathers.
The open boundary conditions of the model are defined as C = 0, considering that they are separate from the source of origin. The initial condition is known as C(t = 0) = 0 (t = 0 corresponds to the beginning of the deep spill). At the sea and bottom surfaces, the fluxes of hydrogen sulfide are to be zero. If this condition is fully applicable, then the zero flux at the sea surface is close in proximity due to the possibility of evaporation of the substance. The necessary parameters for (2) and (3) are defined in in Appendix A.
The final validation of the concentration fields obtained from hydrogen sulfide was not conducted due to the lack of detailed in situ observations on this diluted chemical compound. This comparative approach allows us to assess the potential impacts and behavior of H2S in different environmental settings. Numerical methods are the most viable approach to conduct this evaluation, particularly considering that these contaminants cannot be detected using remote sensors.
3. Results
in Appendix A, provides an overview of H2Sconcentrations at various depths and distances obtained from the hydrodynamic model. The data represent concentrations at depths ranging from 1,065 to 1,200 m, as well as two different distances from the spill source, namely 2.3 and 16.5 km, for both anthropogenic and natural H2S sources.
represents the profiles of concentration and depths hydrogen sulfide at hypothetical natural hydrothermal and anthropogenic discharges in a study domain in the Gulf of Mexico and with the hydrodynamic conditions of 1 May 2010 with a time domain of 2.0/4.0 days.
Figure 6. Profiles of concentration and depths hydrogen sulfide at hypothetical natural hydrothermal and anthropogenic discharge.

The model-computed hydrogen sulfide concentrations exhibited a slight spatial variation between natural and anthropogenic discharges. Given the source of discharges is in the center of the domain, there was no significant upward spatial variation observed in the hydrogen sulfide profile at different distances from the source and at different depths.
The hydrogen sulfide profile at 2.3 km SW of DWH shows a concentration change at a depth of 1,065 m with more input of the H2S from the anthropogenic discharge compared to the natural discharge. At this depth, the range of concentrations in the anthropogenic discharge is greater than the range of these concerning the natural discharge, which has a concentration of
vs
.
The hydrogen sulfide profile at 16.5 km SW of the DWH shows a slight concentration change at a depth of 1,065 m, with a higher input of from the anthropogenic discharge compared to the natural discharge. At this depth, the range of concentrations in the anthropogenic discharge is greater than that in the natural discharge, which has a concentration of
compared to
.
The hydrogen sulfide profile at 2.3 km SW of DWH shows a concentration change at a depth of 1,200 m with more input of the H2S from the natural discharge compared to the anthropogenic discharge. At this depth, the range of concentrations in the anthropogenic discharge is lower than the range of these concerning the natural discharge, which has a concentration of
vs
.
The hydrogen sulfide profile at 16.5 km SW of DWH shows a concentration change at a depth of 1,200 m with more input of the H2S from the natural discharge compared to the anthropogenic discharge. At this depth, the range of concentrations in the anthropogenic discharge is lower than the range of these concerning the natural discharge, which has a concentration of
vs
. (left) illustrates the concentration profiles and depths of Hydrogen sulfide at the hypothetical natural hydrothermal and anthropogenic discharge at a depth of 2.3 km. The color green represents a positive anomaly, indicating that the concentration of H2S in the anthropogenic discharge exceeds that of the natural discharge, serving as a baseline comparison. However, as the anthropogenic discharge ascends, a transition occurs from a positive anomaly to a negative anomaly. This means that the concentration of H2S surpasses the concentration of the natural discharge at those depths, indicating an alteration in the prevailing conditions. (right) displays the concentration profiles and depths of hydrogen sulfide at the hypothetical natural hydrothermal and anthropogenic discharge at a depth of 16.5 km. Similar to the previous scenario, the color green denotes a positive anomaly, indicating that the concentration of H2S in the anthropogenic discharge is higher than that of the natural discharge, serving as a baseline reference. However, in this case, the observed trend shows a smaller impact on the magnitude of the concentration anomalies. Although the anthropogenic discharge still exhibits a shift from positive to negative anomalies as it ascends, the deviation from the natural discharge concentration is less pronounced compared to the 2.3 km depth scenario.
Figure 7. Concentration anomalies of hydrogen sulfide at hypothetical natural hydrothermal and anthropogenic discharge: 2.3 km depth (left) and 16.5 km depth (right).

In both scenarios, the concentration profiles have a similar behavior. The H2S concentration at depth conditions the natural discharge is higher than anthropogenic discharges, and during the ascent the balance is reversed, with the H2S concentration in anthropogenic discharge higher than for natural discharges.
The profiles at 1,200 and 1,065 m of depth show an appreciable difference between both types of discharges with a greater area of impact for anthropogenic from natural spills at 1,200 m, and a greater area of impact for natural from anthropogenic spills at 1,065 m. Therefore, the exclusive fact of decreasing droplet size and increasing water contact surface (increasing droplet count) not results in the increment possibility of hydrogen sulfide solubility.
The numerical model presents the same order of magnitude of the resulting concentration for both natural and anthropogenic discharges, and this model is effectively sensitive to the distribution of droplet sizes.
4. Discussion
This study has highlighted the importance of considering chemical pollution produced by human activities, such as hydrocarbon emissions, in the marine environment. In addition to organic components, it is crucial to take into account other chemical compounds present in oil spills, such as . Assessing the anthropogenic impact of these pollutants in deep oil spill conditions requires field data and a multidisciplinary scientific approach for accurate interpretation.
The main objective of this research was to compare the anomalies caused by potential contamination in the marine environment due to the presence of diluted H2S from anthropogenic sources and compare them to natural sources. It has been demonstrated that natural sources of H2S are not considered contaminants and can serve as a baseline for evaluating the impact of substances that cannot be detected through remote sensing. This analysis will contribute to a more comprehensive understanding of potential contamination and aid in identifying and mitigating the impacts of anthropogenic pollutants on the marine ecosystem.
The difference between hydrogen sulfide contamination on a deep anthropogenic discharge in comparison with a natural spills is well known. The application of the Eulerian model allowed quantifying the variation of diluted hydrogen sulfide in the water column for both anthropogenic and natural spills.
The Eulerian model for these conditions considered measurable variables, such as thermohaline stratification, the initial composition of the hydrocarbon in the oil spill, and the hydrothermal, as well as other physical characteristics of the environment to simulate the behavior of the plumes.
The hydrodynamic model adapted to the simulation of the behavior of the soluble components in the case of the 2010 DWH. The modeling found that hydrogen sulfide contamination in a deep anthropogenic spill does always not exceed hydrogen sulfide contamination in a natural discharge, supposing the spillage occurred in an equal location of the source at the seabed.
The change in the concentration of solubilized hydrogen sulfide in the water column showed that although the value of an anthropogenic discharge does not always exceed the range of values of a natural spill, this contaminant may not be negligible. Given the degree of toxicity of these compounds, it is recommended to include in the environmental evaluations’ application of this type of tool for the licenses, before the offshore activities of the exploitation of natural resources.
The developed model can be a starting point to provide information from the perspective of chemical oceanography, useful to locate potential areas for exploration of minerals and energy that can be provided by the underwater hydrothermal vents, and facilitate environmental assessments of such projects.
In summary, this study underscores the need to consider chemical pollution in oceanic ecosystems and emphasizes the importance of proper management to minimize the negative effects of human activities on the oceans. An integrated and multidisciplinary approach is required to address these challenges and ensure the long-term preservation and health of our oceans.
The findings of this study suggest that hydrogen sulfide concentrations, in principle, do not impose a constraint on the viability of anthropogenic activities at greater depths. Nevertheless, it is crucial to evaluate the impact of these contaminants at individual sites using numerical models, which can serve as accessible tools for comprehensive analysis and assessment.
Consent for publication
All authors agree to publish this research (including any individual details and images) in Environmental Pollutants and Bioavailability.
Contributions
All authors provided support and contributions to the conception and design of this article. Sample collection and data collection were conducted by Andrés Rodríguez. Manuscript writing was completed by Andrés Rodríguez, and constructive comments from Jaíro Cubillos and Serguei Lonin guided the research process. All authors read and approved the final manuscript.
Disclosure statement
Author 1 conceived the idea, collected the data, developed the workflow, performed the assessment and did the background research. Authors 2 and 3 supervised the research and provided critical feedback.
Data availability statement
The datasets supporting the relevant research findings in this article are available from the corresponding author (Andrés Rodríguez) through reasonable request.
Correction Statement
This article has been corrected with minor changes. These changes do not impact the academic content of the article.
References
- Ray D, Sreenivas B, Prakash LS, et al. Fe and S-isotope compositions of hydrothermal deposits from Kings Triple Junction, Lau Basin, southwest Pacific Ocean. Marine Chem. 2021;230:103929. doi: 10.1016/j.marchem.2021.103929
- Milesi V, Shock E, Ely T, et al. Forward geochemical modeling as a guiding tool during exploration of Sea Cliff hydrothermal field, Gorda Ridge. Planet Space Sci. 2020;197:105151. doi: 10.1016/j.pss.2020.105151
- CNRS. New form of sulfur discovered in geological fluids. ScienceDaily. 2011.
- Saint-Macary A, Barr NG, Armstrong EA, et al. The influence of ocean acidification and warming on DMSP & DMS in New Zealand Coastal Water. Atmosphere. 2021;12(2):181. doi: 10.3390/atmos12020181
- Amend JP, Edwards KJ, Lyons TW. Sulfur biogeochemistry: past and present. Geol Soc Am. 2004.
- Ann Moran M, Durham BP. Sulfur metabolites in the pelagic ocean. Nature Rev Microbiol. 2019;17(11):665–363. doi: 10.1038/s41579-019-0250-1
- Laakso T, Anna Waldeck FM, Johnston D. Volcanic controls on seawater sulfate over the past 120 million years. Proc Natl Acad Sci, USA. 2020;117(35):21118–21124. doi: 10.1073/pnas.1921308117
- Rodríguez A, Chang D, Calzada A, et al. Probabilistic modeling of oil spills at the exclusive economic Zone of Cuba using Petromar-3D model. J Geosci Environ Prot. 2021;9(6):21–34. doi: 10.4236/gep.2021.96002
- Rodríguez RA, Lonin SA. Influencia de los dispersantes en la contaminación por btex en un derrame profundo de hidrocarburos. Bol Cient CIOH. 2016;34(34):3–12. doi: 10.26640/22159045.423
- Ryerson TB, Camilli R, Kessler JD, et al. Chemical data quantify Deepwater Horizon hydrocarbon flow rate and environmental distribution. Proc Natl Acad Sci, USA. 2012;109(50):20246–20253. doi: 10.1073/pnas.1110564109
- Bracco A, Paris CB, Esbaugh AJ, et al. Transport, fate and impacts of the deep plume of petroleum hydrocarbons formed during the Macondo blowout. Front Mar Sci. 2020;7(542147):1–22. doi: 10.3389/fmars.2020.542147
- Shigenaka G. Chapter 27 - Effects of Oil in the Environment. Boston: Gulf Professional Publishing; 2011. doi: 10.1016/B978-1-85617-943-0.10027-9
- Zhou Z, Guo L, Shiller AM, et al. Characterization of oil components from the Deepwater Horizon oil spill in the Gulf of Mexico using fluorescence EEM and PARAFAC Techniques. Mar Chem. 2013;148:10–21. doi: 10.1016/j.marchem.2012.10.003
- Reddy CM, Arey JS, Seewald JS, et al. Composition and fate of gas and oil released to the water column during the Deepwater Horizon oil spill. Proc Natl Acad Sci, USA. 2012;109(50):20229–20234. doi: 10.1073/pnas.1101242108
- Blumberg AF. A primer for ECOMSED, version 1.3, users manual, HydroQual. HydroQual, Inc.; 2002.
- Uchida T, Nagamine I, Yabe I, et al. Dissolution process observation of methane bubbles in the deep ocean simulator facility. Energies. 2020;13(3938):2–11. doi: 10.3390/en13153938
- Wankel SD, Germanovich LN, Lilley MD, et al. Influence of subsurface biosphere on geochemical fluxes from diffuse hydrothermal fluids. Nat Geosci. 2011;4(7):461–468. doi: 10.1038/ngeo1183
- Drozd GT, Worton DR, Aeppli C, et al. Modeling comprehensive chemical composition of weathered oil following a marine spill to predict ozone and potential secondary aerosol formation and constrain transport pathways. J Geophys Res Oceans. 2015;120(11):7300–7315. doi: 10.1002/2015JC011093
- Roussak O, Gesser HD. Applied chemistry: a textbook for engineers and technologists. Springer US: SpringerLink: Bücher; 2012.
- Nissanka ID, Yapa PD. Calculation of oil droplet size distribution in ocean oil spills: A review. Mar Pollut Bull. 2018;135:723–734. doi: 10.1016/j.marpolbul.2018.07.048
- Ming L, Garrett C. The relationship between oil droplet size and upper ocean turbulence. Mar Pollut Bull. 1998;36(12):961–970. doi: 10.1016/S0025-326X(98)00096-4
- Yapa PD, Wimalaratne MR, Dissanayake AL, et al. How does oil and gas behave when released in deepwater? J Hydro-Environ Res. 2012;6(4):275–285. doi: 10.1016/j.jher.2012.05.002
- Copernicus Marine Service. Global ocean physics reanalysis. 2022.
- Chen Y. Development of an oil spill model adaptable to exposure and submergence conversion of tidal flats: A case study in the Changjiang estuary. Mar Pollut Bull. 2021;171:112715. doi: 10.1016/j.marpolbul.2021.112715
- Warren LM, Harriot P, Cleveland Smith J. Operaciones unitarias en ingeniería química. Vol. 7. Mexico: McGraw Hill Interamericana; 2005.
- Kretschmar U, McBride D. The metallogeny of lode gold deposits: a syngenetic perspective. Geosci Canada.2015;43(4):291–3
- Von Damm KL. Seafloor hydrothermal activity: Black smoker chemistry and chimneys. Annu Rev Earth Planet Sci. 1990;18(1):173–204. doi: 10.1146/annurev.ea.18.050190.001133
- Gamo T, Chiba H, Yamanaka T, et al. Chemical characteristics of newly discovered black smoker fluids and associated hydrothermal plumes at the Rodriguez Triple Junction, Central Indian Ridge. Earth Planet Sci Lett. 2001;193(3–4):371–379. doi: 10.1016/S0012-821X(01)00511-8
- Ecured. [cited 2022 05 6]. Available from https://www.ecured.cu.
- Estapa M, Breier J, German C. Particle dynamics in the rising plume at piccard hydrothermal field, mid-Cayman rise. Geochem Geophys Geosyst. 2015;16(8):2762–2774. doi: 10.1002/2015GC005831
- Bjørlykke K, Mondol N. Knut Bjørlykke (ed) 2015 petroleum geoscience. From Sedimentary Environ Rock Phys. 2017;10
- Zheng L, Yapa PD, Chen F. A model for simulating deepwater oil and gas blowouts - part I: Theory and model formulation. J Hydraul Res. 2010 February;41(4):339–351. doi: 10.1080/00221680309499980
Appendix A
Table A1. Concentration of H2S at different depths and distances.
Table A2. Summary of data references for hydrothermal simulations [Citation28].
Table A3. Parameters used in the simulations.
Table A4. Properties of the fluids for the two discharges (anthropogenic and natural).