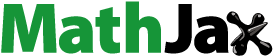
ABSTRACT
Heavy metals contamination in soil from copper mine areas is a serious and widespread problem in China. In this study, the content of Tl, Hg, Cu, Cd, Ni, Pb and As and probabilistic human health risks were investigated in a typical copper mining city. Copper showed the highest average content of 184.29 mg/kg, followed by Pb, As, Ni, Tl, Cd and Hg. Approximately 54.2% of study sites were moderately contaminated by Cu, Cd, As and Tl. Among the heavy metals, Hg, Cu and Cd showed relatively high mobility and medium risk in the environment based on the chemical speciation. In particular, Tl was mainly present as Tl(I) in soil with more biovailability and solubility. Moreover, the probabilistic human health risk assessment using Monte-Carlo approach revealed that heavy metals in soil can cause non-carcinogenic risks (adults: 0.03%, children: 48.45%) and carcinogenic risks (adults: 41.79%; children 61.98%). The soil oral ingestion is the main exposure pathway for human exposed to heavy metals. Our findings indicate that the copper mining activities can cause high level of heavy metals in soils, and corresponding health risks. Meanwhile, these results could provide important information and theoretical support the development of precise soil pollution management strategies in copper mining areas.
1. Introduction
Thallium (Tl) is a non-essential and widely dispersed trace element in environment [Citation1]. Due to the high potential toxicity of Tl, it has been listed as a priority pollutant by the U.S. Environmental Protection Agency. The level of Tl in the natural environment is relatively low, the background level of Tl in the earth’s crust, soil, and water is 0.75 mg/kg [Citation2], 0.01–3.00 mg/kg [Citation3], and 0.001–0.25 μg/L [Citation4], respectively. However, due to the high bioavailability of Tl in soil, it can be easily accumulated in plant, and may cause potential harm to humans [Citation5]. In addition, the high content of Tl was also reported in animals (sheep, rabbits, horses, etc.) through the consumption of leaves from Tl contaminated areas [Citation6]. Serious endemic thallotoxicosis, such as hair loss, body-aches, reduced vision and blindness, has occurred due to the high level of Tl in water, soil, and food crops from Guizhou Province, China [Citation7]. Due to its adverse health effect, the Hygiene Ministry of China issued the maximum urinary Tl concentration for the public is 5 μg/L. Furthermore, a four-year study indicated that even the urinary Tl concentration was lower than 5 μg/L for children after treatment, their liver and renal functions did not completely recover [Citation8,Citation9].
Globally, the annual input of Tl was 5000 tons derived from industries and coal combustion [Citation10]. Meanwhile, Tl and Tl-based alloys have been widely used in recent decades for technologically advanced industries [Citation11]. The emerging Tl pollution has attracted more and more public and environmental attention, especially in China [Citation12]. With the development of analytical techniques in recent years, the pollution caused by Tl in soil has been numerously documented. In general, the content of Tl was shown at low content in environment, whereas ferrous and non-ferrous smelting activities and industry processes can increase its content in environment [Citation13]. Some previous studies found that the high level of Tl was recorded in soils from Tl-rich mineralized area [Citation14], Tl-rich pyrite mineralized zone [Citation15], Carlin-type gold deposit area [Citation16], steel-making area [Citation17], etc. More than 10 poisoning events involving Tl have occurred in China since 1997 [Citation14].
As an important mineral resource, copper (Cu) is an essential non-ferrous metal and widely used in electronics, construction industry, transportation, and so on [Citation18]. China is the largest copper consumer in the world, with an annual consumption being 13.89 million tons in 2021. It is estimated that the cumulative demand for copper in China will reach 360 million tons from 2022 to 2050 [Citation19]. Therefore, copper is also listed in the catalog of strategic key minerals in China. Tongling city, the most important copper product area, has a long history of Cu mining activities for more than 3000 years [Citation20]. Due to its long mining history and various kinds of non-ferrous metal mines, this city is known as the ‘ancient Chinese copper capital’ and ‘modern Chinese copper base’ in China [Citation21,Citation22]. It produced approximately 1.1 million tons of electrolytic copper annually. Therefore, this area is believed to be representative Cu mining districts in eastern China [Citation23]. Unfortunately, some heavy metals can be accumulated in the soil near copper mines due to the metallurgical process [Citation24,Citation25]. Previous studies demonstrated that the presence of heavy metals in soil can cause potential human health risk for local residents [Citation26–28]. Furthermore, soil pollution by heavy metals is considered to be the biggest challenge to sustainable development [Citation29]. Out of 17 sustainable development goals (SDGs) set by United Nations in 2015, eight issues are directly or indirectly relevant to soil pollution [Citation30].
Previous studies have mentioned that Tl is a mildly chalcophile element [Citation31,Citation32], and can be incorporated into the pyrite structure as a substitution, which leads to a relatively high level of Tl in pyrite [Citation33]. Currently, a considerable count of publications documented Tl pollution in soils [Citation34], vegetables [Citation35], teas [Citation36], plants [Citation37], surface water and sediments [Citation38]. However, the content of Tl and its human health risk remains poorly studied in this representative Cu mining district. Therefore, the main objectives of this study were (1) to investigate the levels of Tl and other heavy metals in the soils near copper smelting plants in Tongling city and (2) to evaluate the health risk of human exposure to multiple heavy metals in soil.
2. Materials and methods
2.1. Study area and sample collection
Tongling (117°42′00″-118°10′6″, 30°45′12″-31°07′56″) is a typical medium-sized industrial city and belongs to the Yangtze River basin, located in the central-south of Anhui province. This region belongs to a mild climate, and the mean annual temperature is 16°C, the average annual precipitation is 1930 mm, the average humidity is 83%, as well the frost-free period is approximately 230 days per year. The mineral deposits of investigated area are polymetallic (iron-copper-gold-sulfide) deposits, and the metal minerals mainly contain magnetite, hematite, chalcopyrite, pyrite, siderite [Citation39]. In addition, the soils in study area are mainly brown red soil (Ferralosols).
In this study, the abandoned industrial area was selected, and the levels of heavy metals in soils were measured. Totally, 211 soil samples from 65 sites were collected (), and each soil sample was commixed by five subsamples. All the samples were stored in clean aluminum and then returned to the laboratory for naturally air dried and ground to 100 mesh. The processed soil samples were sealed in polyethylene plastic container and store at 4°C for future chemical analysis [Citation40].
2.2. Measurement of metal concentrations
The soil sample digestion was performed using a microwave-assisted acid-digestion procedure following the national standard method HJ 832–2017 [Citation41]. In brief, approximately 100 mg sieved soil was weighed in a Teflon digestion tube followed by an addition of 11 mL acid mixture digestion systems (6 mL HNO3 +3 mL HCl +2 mL HF) [Citation42]. The concentrations of heavy metals were measured using an inductively coupled plasma emission spectrometer (ICAP6300, Thermo Fisher Scientific, U.S.A.). In addition, the blank, standard reference material (Geochemical Standard Reference Soil of China, GB W07443) was used to control and calibrate the analysis procedure. All samples were analyzed in triplicate and the relative error of the duplicate samples was <5% on average, and the heavy metals recovery rate of the reference material ranged from 96% to 102%.
2.3. Measurement of metal speciation
The processed soil samples were extracted using the European Community Bureau of Reference (BCR) sequential extraction procedure according to our previous studies [Citation42,Citation43]. After extraction, the heavy metals can be described as four fractions (i.e. acid-soluble fraction, reducible fraction, oxidizable fraction, and residual fraction). The detailed BCR procedure for heavy metals in soils was shown in Table S1. The concentrations of heavy metals in all steps were determined by ICP-OES. Additionally, the recovery percentages of all the heavy metals were ranged from 92% to 115%. The chemical oxidation states of heavy metals in soil samples were examined using X-ray Photoelectron Spectroscopy (XPS, Thermo Fischer, ESCALAB Xi+, U.S.A.), which was equipped with Al Kα X-ray source (1486.6 eV). The binding energies were referenced to the C1s signal at 284.6 eV.
2.4. Risk assessment
2.4.1. Index of geo-accumulation
The geo-accumulation index (Igeo) has been widely used to assess heavy metals pollution in soils or sediments since the late 1960s [Citation44], which is calculated using the following equation:
Where, n represents the certain metal in soils, Cn and Bn are the heavy metal contents in collected soil samples and the geochemical background values of soils, respectively. 1.5 represents the constant factor due to lithospheric effects [Citation45]. On the basis of this index, seven grades of Igeo can be divided to present different contamination levels, which was shown in Table S2.
2.4.2. Exposure assessment
To describe the quantitative health risk of exposure to heavy metals in soils, the assessment model proposed by the USEPA was selected. In this study, the carcinogenic and noncarcinogenic risk from heavy metals for adults and children in study area was assessed under three exposure pathways (ingestion, inhalation and dermal absorption) [Citation46]. The average daily intake (ADD, mg/kg/day) of heavy metals was calculated according to the following formulae:
Where ADDingestion, ADDinhalation and ADDdermal are the average daily doses through ingestion, inhalation and dermal contact, respectively, in mg/(kg∙day). Csoil is content of heavy metals in the soil (mg/kg); IngR is the soil ingestion rate (mg/day); InhR is the air inhalation rate (m3/day); EF and ED are the exposure frequency (day/year), and exposure duration (year), respectively; BW shows the body weight (kg); The term AT represents the average exposure time (day). SA is dermal surface area (cm2/day), and AF is the soil adherence factor (mg/(cm2∙day)). ABS demonstrates dermal fraction absorbed from the soil (unitless). PEF represents the particulate emission factor of heavy metals (m3/kg). The assumed values for health risk assessment are given in Table S3.
2.4.3. Human health risk assessment
The carcinogenic risk index (TCR) and the noncarcinogenic risk index (HI) were calculated [Citation47].
where i presents one heavy metal in soil; j indicates the three exposure pathways for soil; HIsoil is the sum of the hazard quotient of various heavy metals through different exposure pathway (unitless); TCRsoil represents the total carcinogenic risk through the three exposure pathways (unitless); CRi is the carcinogenic risk of heavy metal i (unitless); HQi is the hazard quotient of heavy metal i (unitless); RfDi is the reference oral dose available for heavy metal i (mg/(kg∙day)); SFi is the cancer slope factor for heavy metal i (mg/(kg∙day)) . The toxicity parameters for heavy metals were shown in Table S4.
The criteria for risk are as follows: no significant health risk (CR or TCR < 10−6); acceptable/tolerable (10−6 < CR or TCR < 10−4); unacceptable (CR or TCR > 10−4). Humans may tolerate the potentially toxic effects if the HQ or HI value exceeds 1.0.
2.4.4. Probabilistic risk modeling and sensitivity analysis
To reduce the uncertainty of the health risk of elements, a Monte Carlo simulation method was conducted to assess the probabilistic risk using the Oracle Crystal Ball program (version 11.1, Oracle, U.S.A.) loaded in Microsoft Excel [Citation48]. Furthermore, the sensitivity analysis was conducted to estimate the key factors that influenced the results of risk. The parameter distribution types used in this study were listed in Table S3. In this study, the THQ and CR were repeatedly and randomly repeated 10,000 times to obtain more creditable outputs and sensitivity index.
2.5. Data and statistical analyses
The arithmetic mean, range, and standard deviation were used to analyze the content of heavy metals in soils. Spearman’s correlation was used to explore sources of heavy metals. The Origin Pro 2022 (Origin Lab Corporation, Northampton, U.S.A.) was used to create the figures in this study.
3. Results and discussion
3.1. Contents of heavy metals in soils
The descriptive statistics of contents for heavy metals are shown in , including the items of percentage, median and mean. Comparing the contents of heavy metals with the national background values of China, all the heavy metals in soils exceeded the corresponding background values in the study area. This suggests that heavy metals in the soil were heavily influenced by pollution from mining activities. The range of heavy metals in the soils was: 31.00–562.00 mg/kg for Cu, 10.4–408.00 mg/kg for As, 0.60–9.7 mg/kg for Tl, 0.18–0.61 mg/kg for Hg, 0.28–5.44 mg/kg for Cd, 33.00–298.00 mg/kg for Pb, 26.00–197.00 mg/kg for Ni. The maximum contents of all heavy metals except Hg were higher than their corresponding recommended risk screening values in China (GB15618–2018). The mean value of content for heavy metals in soils showed a trend of Cu>Pb>As>Ni>Tl>Cd>Hg.
Table 1. Summary statistics for the content of heavy metals in soils (unit: mg/kg).
Particularly, as shown in Table S5, the level of Tl in soils was higher than the geochemical background value of soils from the Yangtze river – Huaihe river basin of Anhui province (0.60 mg/kg), indicating that Tl was likely introduced into soils through some anthropogenic effects. Previous studies indicated that human activities (i.e. mining and agricultural activities) are the main factors affecting the behaviors of Tl in the soil environment [Citation14]. Besides, the mean Tl content in this study exceeds that of vegetable soils, forest soils and grasslands from previous research [Citation49–51]. Thallium contents in this study were lower than the soil samples collected from Spain and China reported by Cortada et al., Jiang et al. and Xiao et al. [Citation16,Citation52]. Since no environmental standards for Tl in soil are established in China, Xiao et al. suggested the maximum content of Tl in arable soils should be regulated at 1 mg/kg [Citation14]. In this study, only 11 of 211 samples, the content of Tl was less than 1 mg/kg.
To further understand the pollution level of heavy metals, the geo-accumulation index (Igeo) was calculated, and the result was shown in . Cd had the highest Igeo value (2.30), followed by Cu (2.01), Hg (1.71), Pb (1.48), As (0.95), Tl (0.59), and Ni (0.54). According to the classification of Igeo, approximately 54.2% of soils were moderately contaminated, and nearly 4.1% of soils were extremely contaminated by Cd. This result is highly consistent with those reported data in Tongling city [Citation28].
The correlation analysis was used to analyze the inter-relationship between the contents of elements in this study. And the results are plotted in . High positive linear relationships were found between Pb and Cd, Tl and Pb, Tl and Cd. The high correlations suggested that these elements might have similar sources or common pollution pathways.
3.2. Speciation of heavy metals in soils
The chemical speciation of heavy metals can control their bioavailability, mobility, reactivity and toxicity in soils. The chemical speciation can be divided into four categories, the acid soluble/exchangeable fraction (F1), reducible fraction (F2), oxidizable fraction (F3) and residual fraction (F4). And the F1 and F2 can be considered the mobile fractions in the environment. The results of the BCR and risk assessment code (RAC) of heavy metals in soils are presented in . According to , As, Ni and Cu were more concentrated in the residual fraction (F4), with the percentage was higher than 50%, these heavy metals were likely immobilized and will not pose severe hazards in the environment. Furthermore, the high value of Cu in oxidizable fraction (F3) soils was due to the organic matter in soils, as Cu showed a strong affinity with humic substances in soils [Citation53]. In contrast, the mobilization of heavy metals, such as Cd, Tl, Pb and Hg was high, due to their high values of ∑(F1+F2+F3), these fractions were regarded as directly bioavailable and potentially bioavailable [Citation42]. In addition, Hg was found more in the exchangeable fraction (F1), with a percentage of 28.3%, which may cause negative effects because this fraction is bound with carbonates that can readily release to the environment [Citation54].
The environment implication of heavy metals was also calculated by risk assessment codes (RAC). In this study, based on the result of , the RAC of heavy metals showed a trend: Hg>Cu>Cd>Ni>Tl>Pb>As. The Hg, Cu and Cd presented medium risk because their RAC values were higher that 10%. Low risk (<10%) was observed in soils for Ni, Tl, Pb and As.
To investigate the chemical states of heavy metals, the soil sample was characterized by XPS. The results of XPS analysis are shown in , some intense peaks in wide-scan spectra can be attributed to O1s, C2s, Si2p and Fe2p. According to the results of XPS (), Tl main occurs as weakly hydrated monovalent cation Tl(I) in soils [Citation55], with a percentage of approximately 71.3%. The Tl(I) is the common speciation of Tl in the environment, and can be more bioavailable because of its high solubility. In contrast, the toxicity of Tl(III) is much less than Tl(I) [Citation56]. This result was in good agreement with the study of Tl-As rich soils from Yunnan, China [Citation56].
3.3. Human health risk assessment based on Monte Carlo simulation
3.3.1. Potential non-carcinogenic risk
The Monte Carlo simulation results of non-carcinogenic risk through soil exposure to heavy metals are shown in and . The median value of HI for children and adults were all <1, with the trend of As>Pb>Tl>Cu>Hg>Ni>Cd. Arsenic showed the highest contribution to the total non-carcinogenic risk by comparing the largest HQ value, this result was consistent with the research on the global copper mines, which found that the As accounted for more than 70% of HI value for eight heavy metals [Citation27]. Meanwhile, the mean value of HI for adults was 0.23 with 95% CI of 0.08 to 0.25, and the mean value for children (HIchildren) was 1.09 with 95% CI of 0.70 to 1.34. Compared with the guideline value of HI, the HI value for adult was much lower than 1.0, indicating no potential noncariogenic risk. Meanwhile, approximately 48.45% of the HI value for children surpassed 1.0, indicating non-negligible potential non-carcinogenic risk (). Moreover, the non-cariogenic risk was mainly from soil ingestion, which accounted for 99.23% and 82.93% of the total risk for children and adults, respectively. This meant that oral ingestion was the major exposure pathway in this study. These results may be due to the hand-to-mouth behavior of children, thus children were more vulnerable to non-carcinogenic risks than adults [Citation27]. Furthermore, the HI values were >10 folds as high as agricultural soil reported by Wang et al. [Citation57]. Hence, the potential non-carcinogenic risk for soils in this study should pay closer attention to heavy metals exposure for children, especially to As.
Figure 7. The probabilities distribution of carcinogenic risk (a) and non-carcinogenic (b) risks for local residents (the black short dot line presents the risk boundary, TCR = 1.0E–4, HI = 1).

Table 2. Summary statistics of health risk based on Monte Carlo simulation.
Additionally, the probability distribution of HQ value for each heavy metal was shown in Fig. S1, all the heavy metal, except for As, with negligible non-carcinogenic risk (HQ < 1). And As with 0.01% and 26.56% probability had a higher HQ value than 1.0 for adults and children, respectively, which indicated the potential non-carcinogenic risk for local residents.
3.3.2. Potential carcinogenic risk
The potential carcinogenic risk (CR) of heavy metals for local residents is shown in . It was worth noticing that the CR of adults was higher than that of children, which was consistent with the results of HI. With regard to the exposure pathway, oral ingestion accounted for 93.18% and 95.68% of the TCR value for adults and children, respectively. The mean value of TCR for adults and children were 1.21E–4 and 1.24E–4, respectively, which was higher than the acceptable range (1.00E–6 to 1.00E–4) recommended by USEPA. The probability of carcinogenic risk (CR) for all heavy metals for adults and children was 41.79% and 61.98%, respectively. Furthermore, the CR value for Cu showed the highest percentage (60.06%) of CR, followed by As (19.67%), Ni (19.55%) and Cd (0.73%), indicating that Cu and As was the major carcinogenic factor in this study (). In addition, the CR values of Pb and Cd were within the acceptable threshold that indicating no cancer risk for both adults and children.
3.3.3. Sensitivity analysis
The sensitivity analysis of the impacts of parameters on the risk was performed with Crystal Ball software. The result was shown in . In general, the value of the total noncarcinogenic risk (HI) and total carcinogenic risk (TCR) was found positively sensitive to the content of heavy metals, exposure frequency (EF), the soil ingestion rate (IngR) and the air inhalation rate (InhR), while negatively sensitive to body weight (BW). For the HI, the content of Pb in soil showed the most notable contribution to the risk, with proportions of 48.64% and 51.38% for adults and children, respectively. Regarding the TCR, the content of Cu was significantly responsible for cancer risk estimation, accounting for 55.60% and 65.83% for adults and children, respectively. The results implied that heavy metals in the soil were the most sensitive factor for both noncarcinogenic and carcinogenic risk, and effective controlling measures were required to decrease Pb, As and Cu content to effectively reduce the health risk to local residents.
4. Conclusions and recommendations
The content, chemical speciation, human health risk of Tl and other heavy metals in soil from copper mining area were studied in this study. The results demonstrated that the content of all heavy metals exceeded the background value of Tongling city. Cadmium showed the highest geo-accumulation (Igeo) index, followed by Cu, Hg, Pb, As, Tl and Ni. Furthermore, approximately 54.2% of soils were moderately contaminated, based on the classification of Igeo. The chemical speciation demonstrated that the Hg, Cu and Cd had more bioavailable fractions, which can readily be released to the environment. In addition, Tl predominantly occurred in the form of Tl(I) in soil. The health risk assessment revealed that the non-carcinogenic risk and carcinogenic risk for children were higher than that for adults, the probability of risk for children was approximately 48.45% and 61.98% for non-carcinogenic risk and carcinogenic risk, respectively. Furthermore, based on the Monte Carlo sensitivity analysis, the health risk was most sensitive to heavy metal content, especially Cu, As and Pb. Furthermore, this study recommended that some soil management strategies should be implemented to protect public and environmental health. Further, comprehensive studies are needed to determine the sources of heavy metals in soil from this area.
Authors contributions
Kefei Sun, Hui Li and Zixuan Jiang: investigation, methodology, writing-original draft. Yunhua Zhang: resources, supervision, writing – review & editing, fund acquisition. Yanhong Shi: project administration. Jiale Gao, Jiaqi Shi and Yan Fang: investigation. Ting Fang and Jie Kong: writing – review & editing. Zixuan Jiang, Kongyong Ouyang and Ningyuan Zhang: sample collection. Xinju Dong: writing – review & editing.
Supplemental Material
Download MS Word (1.8 MB)Disclosure statement
No potential conflict of interest was reported by the authors.
Data availability statement
The data that support the findings of this study are available from the corresponding author, upon reasonable request.
Supplementary material
Supplemental data for this article can be accessed online at https://doi.org/10.1080/26395940.2023.2250912
Additional information
Funding
References
- Cruz-Hernández Y, Ruiz-García M, Villalobos M, et al. Fractionation and mobility of thallium in areas impacted by mining-metallurgical activities: Identification of a water-soluble Tl(I) fraction. Environ Pollut. 2018;237:154–408. doi: 10.1016/j.envpol.2018.02.031
- Taylor SR, McLennan SM. The continental crust: its composition and evolution. Palo Alto, CA: United States: Blackwell Scientific Pub; 1985.
- Chaney RL. The heavy elements: chemistry. Environ Impact, And Health Eff J Environ Qual. 1991;20(4):876–876. doi: 10.2134/jeq1991.00472425002000040028x
- Banks D, Reimann C, Røyset O, et al. Natural concentrations of major and trace elements in some Norwegian bedrock groundwaters. Appl Geochem. 1995;10(1):1–16. doi: 10.1016/0883-2927(94)00046-9
- Luo Z, Kayiranga A, Uwiringiyimana E, et al. Thallium contamination in agricultural soils and associated potential remediation via biochar utilization. Biochar. 2020;2(1):33–46. doi: 10.1007/s42773-020-00042-6
- Jia Y, Xiao T, Zhou G, et al. Thallium at the interface of soil and green cabbage (Brassica oleracea L. var. capitata L.): soil–plant transfer and influencing factors. Sci Total Environ. 2013;450-451:140–147. doi: 10.1016/j.scitotenv.2013.02.008
- Li S, Xiao T, Zheng B. Medical geology of arsenic, selenium and thallium in China. Sci Total Environ. 2012;421-422:31–40. doi: 10.1016/j.scitotenv.2011.02.040
- Duan W, Wang Y, Li Z, et al. Thallium exposure at low concentration leads to early damage on multiple organs in children: a case study followed-up for four years. Environ Pollut. 2020;258:113319. doi: 10.1016/j.envpol.2019.113319
- Tong J, Liang C, Wu X, et al. Prenatal serum thallium exposure and cognitive development among preschool-aged children: a prospective cohort study in China. Environ Pollut. 2022;293:118545. doi: 10.1016/j.envpol.2021.118545
- Kazantzis G. Thallium in the environment and health effects. Environ Geochem Health. 2000;22(4):275–280. doi: 10.1023/A:1006791514080
- Cyubahiro E, Luo Z, Wang H, et al. Effect of montmorillonite biochar composite amendment on thallium bioavailability in contaminated agricultural soils and its mitigated health risk. Environ Sci Pollut Res. 2023;30(16):47882–47891. doi: 10.1007/s11356-023-25668-4
- Peter ALJ, Viraraghavan T. Thallium: a review of public health and environmental concerns. Environ Int. 2005;31(4):493–501. doi: 10.1016/j.envint.2004.09.003
- Wang J, Zhou Y, Dong X, et al. Temporal sedimentary record of thallium pollution in an urban lake: an emerging thallium pollution source from copper metallurgy. Chemosphere. 2020;242:125172. doi: 10.1016/j.chemosphere.2019.125172
- Xiao T, Yang F, Li S, et al. Thallium pollution in China: A geo-environmental perspective. Sci Total Environ. 2012;421-422:51–58. doi: 10.1016/j.scitotenv.2011.04.008
- Zhou T, Fan Y, Yuan F, et al. A preliminary investigation and evaluation of the thallium environmental impacts of the unmined Xiangquan thallium-only deposit in Hexian, China. Environ Geol. 2008;54(1):131–145. doi: 10.1007/s00254-007-0800-0
- Xiao T, Guha J, Boyle D, et al. Environmental concerns related to high thallium levels in soils and thallium uptake by plants in southwest Guizhou, China. Sci Total Environ. 2004;318(1–3):223–244. doi: 10.1016/S0048-9697(03)00448-0
- Liu J, Wang J, Chen Y, et al. Thallium dispersal and contamination in surface sediments from South China and its source identification. Environ Pollut. 2016;213:878–887. doi: 10.1016/j.envpol.2016.03.023
- Renaud KM, Manley R, Nassar NT. A comparison of copper use in China and India as a proxy for their economic development. Resour Policy. 2023;80:103195. doi: 10.1016/j.resourpol.2022.103195
- Dong D, Tukker A, Van der Voet E. Modeling copper demand in China up to 2050: a business-as-usual scenario based on dynamic stock and flow analysis. J Ind Ecol. 2019;23(6):1363–1380. doi: 10.1111/jiec.12926
- Wang R, Zhang J, Sun H, et al. Effect of different vegetation on copper accumulation of copper-mine abandoned land in tongling, China. J Environ Manag. 2021;286:112227. doi: 10.1016/j.jenvman.2021.112227
- Zhao K, Hu R, Wan X, et al. Heavy metal spatial distribution, sources and ecological risks in farmland soils from three areas in the Yangtze river basin in Anhui. Arabian J Geosci. 2022;15(12):1154. doi: 10.1007/s12517-022-09976-6
- Liu W, Hu T, Mao Y, et al. The mechanistic investigation of geochemical fractionation, bioavailability and release kinetic of heavy metals in contaminated soil of a typical copper-smelter. Environ Pollut. 2022;306:119391. doi: 10.1016/j.envpol.2022.119391
- Xie J, Ge L, Qian L, et al. Trace element characteristics of pyrite in Dongguashan Cu (Au) deposit, Tongling region China. Solid Earth Sci. 2020;5(4):233–246. doi: 10.1016/j.sesci.2020.09.002
- Covre WP, Ramos SJ, Pereira WVDS, et al. Impact of copper mining wastes in the Amazon: properties and risks to environment and human health. J Hazard Mater. 2022;421:126688. doi: 10.1016/j.jhazmat.2021.126688
- Shi J, Zhao D, Ren F, et al. Spatiotemporal variation of soil heavy metals in China: The pollution status and risk assessment. Sci Total Environ. 2023;871:161768. doi: 10.1016/j.scitotenv.2023.161768
- Jiang X, Liu W, Xu H, et al. Characterizations of heavy metal contamination, microbial community, and resistance genes in a tailing of the largest copper mine in China. Environ Pollut. 2021;280:116947. doi: 10.1016/j.envpol.2021.116947
- Chen L, Zhou M, Wang J, et al. A global meta-analysis of heavy metal(loid)s pollution in soils near copper mines: evaluation of pollution level and probabilistic health risks. Sci Total Environ. 2022;835:155441. doi: 10.1016/j.scitotenv.2022.155441
- Shen Z, Xu D, Li L, et al. Ecological and health risks of heavy metal on farmland soils of mining areas around Tongling city, Anhui, China. Environ Sci Pollut Res. 2019;26(15):15698–15709. doi: 10.1007/s11356-019-04463-0
- Sonowal S, NAVA AR, JOSHI SJ, et al. Biosurfactant-assisted phytoremediation of potentially toxic elements in soil: green technology for meeting the United Nations sustainable development goals. PEDOSPHERE. 2022;32(1):198–210. doi: 10.1016/S1002-0160(21)60067-X
- Hou D, O’Connor D, Igalavithana AD, et al. Metal contamination and bioremediation of agricultural soils for food safety and sustainability. Nature Rev Earth Environ. 2020;1(7):366–381. doi: 10.1038/s43017-020-0061-y
- Nielsen SG, Shimizu N, Lee C-TA, et al. Chalcophile behavior of thallium during MORB melting and implications for the sulfur content of the mantle. Geochem Geophys Geosyst. 2014;15(12):4905–4919. doi: 10.1002/2014GC005536
- D’Orazio M, Campanella B, Bramanti E, et al. Thallium pollution in water, soils and plants from a past-mining site of Tuscany: sources, transfer processes and toxicity. J Geochem Explor. 2020;209:106434. doi: 10.1016/j.gexplo.2019.106434
- Xie J, Tang D, Qian L, et al. Geochemistry of sulfide minerals from skarn Cu (Au) deposits in the Fenghuangshan ore field, Tongling, eastern China: Insights into ore-forming process. Ore Geol Rev. 2020;122:103537. doi: 10.1016/j.oregeorev.2020.103537
- Ning Z, Liu E, Yao D, et al. Contamination, oral bioaccessibility and human health risk assessment of thallium and other metal(loid)s in farmland soils around a historic TlHg mining area. Sci Total Environ. 2021;758:143577. doi: 10.1016/j.scitotenv.2020.143577
- Liu J, Wei X, Zhou Y, et al. Thallium contamination, health risk assessment and source apportionment in common vegetables. Sci Total Environ. 2020;703:135547. doi: 10.1016/j.scitotenv.2019.135547
- Kowalczyk E, Givelet L, Amlund H, et al. Risk assessment of rare earth elements, antimony, barium, boron, lithium, tellurium, thallium and vanadium in teas. EFSA J. 2022;20:e200410. doi: 10.2903/j.efsa.2022.e200410
- Wei X, Zhou Y, Tsang DCW, et al. Hyperaccumulation and transport mechanism of thallium and arsenic in brake ferns (Pteris vittata L.): a case study from mining area. J Hazard Mater. 2020;388:121756. doi: 10.1016/j.jhazmat.2019.121756
- Sun F, Tao Y, Liao H, et al. Pollution levels and risk assessment of thallium in Chinese surface water and sediments. Sci Total Environ. 2022;851:158363. doi: 10.1016/j.scitotenv.2022.158363
- Wang J, Su J, Li Z, et al. Source apportionment of heavy metal and their health risks in soil-dustfall-plant system nearby a typical non-ferrous metal mining area of Tongling, eastern China. Environ Pollut. 2019;254:113089. doi: 10.1016/j.envpol.2019.113089
- Dai S, Li H, Yang Z, et al. Effects of biochar amendments on speciation and bioavailability of heavy metals in coal-mine-contaminated soil. Hum Ecol Risk Assess Int J. 2018;24(7):1887–1900. doi: 10.1080/10807039.2018.1429250
- MME. Soil and sediment - digestion of Total metal elements - microwave assisted acid digestion method. China Environment Publishing Group; 2017.
- Li H, Xu W, Dai M, et al. Assessing heavy metal pollution in paddy soil from coal mining area, Anhui, China. Environ Monit Assess. 2019;191(8):518. doi: 10.1007/s10661-019-7659-x
- Min X, Ge T, Li H, et al. Combining impregnation and co-pyrolysis to reduce the environmental risk of biochar derived from sewage sludge. Chemosphere. 2022;290:133371. doi: 10.1016/j.chemosphere.2021.133371
- Muller G. Index of geoaccumulation in sediments of the Rhine river. Geojournal. 1969;2:108–118.
- Liu G, Shi Y, Guo G, et al. Soil pollution characteristics and systemic environmental risk assessment of a large-scale arsenic slag contaminated site. J Clean Prod. 2020;251:119721. doi: 10.1016/j.jclepro.2019.119721
- Ayejoto DA, Agbasi JC, Egbueri JC, et al. Assessment of oral and dermal health risk exposures associated with contaminated water resources: an update in Ojoto area, southeast Nigeria. Int J Environ Anal Chem. 2022;1–21.
- Ozturk A, Arici OK. Carcinogenic-potential ecological risk assessment of soils and wheat in the eastern region of Konya (Turkey). Environ Sci Pollut Res. 2021;28(12):15471–15484. doi: 10.1007/s11356-020-11697-w
- Zhou H, Ge T, Li H, et al. A multi-medium analysis of human health risk of toxic elements in rice-crayfish System: a case study from middle reach of Yangtze river, China. Foods. 2022;11(8):1160. doi: 10.3390/foods11081160
- Liu J, Luo X, Wang J, et al. Thallium contamination in arable soils and vegetables around a steel plant—A newly-found significant source of Tl pollution in South China. Environ Pollut. 2017;224:445–453. doi: 10.1016/j.envpol.2017.02.025
- Karbowska B. Presence of thallium in the environment: sources of contaminations, distribution and monitoring methods. Environ Monit Assess. 2016;188(11):640. doi: 10.1007/s10661-016-5647-y
- Vaněk A, Chrastný V, Mihaljevič M, et al. Lithogenic thallium behavior in soils with different land use. J Geochem Explor. 2009;102(1):7–12. doi: 10.1016/j.gexplo.2008.10.004
- Jiang F, Ren B, Hursthouse A, et al. Distribution, source identification, and ecological-health risks of potentially toxic elements (PTEs) in soil of thallium mine area (southwestern Guizhou, China). Environ Sci Pollut Res. 2019;26(16):16556–16567. doi: 10.1007/s11356-019-04997-3
- Ashayeri SY, Keshavarzi B, Moore F, et al. Risk assessment, geochemical speciation, and source apportionment of heavy metals in sediments of an urban river draining into a coastal wetland. Mar Pollut Bull. 2023;186:114389. doi: 10.1016/j.marpolbul.2022.114389
- Palansooriya KN, Shaheen SM, Chen SS, et al. Soil amendments for immobilization of potentially toxic elements in contaminated soils: a critical review. Environ Int. 2020;134:105046. doi: 10.1016/j.envint.2019.105046
- Voegelin A, Pfenninger N, Petrikis J, et al. Thallium speciation and extractability in a thallium- and arsenic-rich soil developed from mineralized carbonate rock. Environ Sci Technol. 2015;49(9):5390–5398. doi: 10.1021/acs.est.5b00629
- Wei X, Wang J, She J, et al. Thallium geochemical fractionation and migration in Tl-As rich soils: the key controls. Sci Total Environ. 2021;784:146995. doi: 10.1016/j.scitotenv.2021.146995
- Wang C-C, Zhang Q-C, Kang S-G, et al. Heavy metal(loid)s in agricultural soil from main grain production regions of China: bioaccessibility and health risks to humans. Sci Total Environ. 2023;858:159819. doi: 10.1016/j.scitotenv.2022.159819