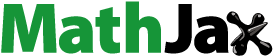
ABSTRACT
Sulphate (SO4) abundance in the earth’s crust contributes largely to industrial wastewater contamination lowering the pH, which exuberates the dissolution of metals forming acidic drainages. Biological sulphate reduction as a remediation process can be affected by factors such as pH, temperature and high sulphide concentrations. In this study, sulphate-reducing bacterial community enriched from mine wastewaters was applied in semi-automated bioreactors to assess the effects of these factors on microbial sulphate reduction capacities. Low pH (3.5) and temperature (10°C) were observed to promote the toxicity of sulphur-reduced species on the consortium while mesophilic temperature (25°C) and near neutral pH (6.2) were observed to induce optimum SO4 reduction attaining a maximum of 95% SO4 reduction. Obtained SO4 reduction dynamics data was then applied in formulating a unique non-competitive inhibition equation that models biogeochemical events during SO4 reduction under varied pH and temperature conditions and predicts the efficacy of a bioremediation system.
1. Introduction
Water as a natural resource needed by all life forms on the planet with extend of serving as a habit to wide range of life forms has been reported to be at risk of contamination globally [Citation1,Citation2]. With some parts of the world being deserts and others covered in ice, fresh surface water becomes a life-limiting factor [Citation3]. Over a decade ago, it was reported that from all water sources including oceans, there is approximately 0.016% of fresh surface water available for human consumption and daily use [Citation4,Citation5]. Most of the water is in the ocean but contains high concentrations of salt which is not readily usable by human while most fresh water is locked in ice of the polar regions of the planet [Citation6]. The rest of the fresh available water is scattered in rivers, ponds, streams, dams and in the subsurface of the earth as groundwater. Anthropogenic activities remain the leading cause of contamination in both developed, developing and primarily rural countries [Citation7,Citation8]. With increase in human population, comes demand of food, water and resources to sustain economies of many countries largely African countries. Farming and mining have been over a century been an economic cornerstone in most African countries which contribute to environmental pollution including water [Citation2]. The earth’s crust is rich in known elements present as compounds and as pure crude deposits in different depth hence the need to mine in extracting these minerals [Citation9]. During mining, explosives are used to crush rocks and make way for deeper access to those embedded deep underground. During this process, tailings are deposited around the mining sites which then exposes these crude minerals to conditions which makes some of them reactive as they become in contact with oxygen (O2), water (H2O) and other chemicals used in extraction of valuable targeted minerals [Citation10]. With sulphur (S0) as one of the most abundant elements, it does become exposed to H2O and O2 which result in SO42- and in favourable conditions, it reacts with hydrogen (H+) forming H2SO4 that is acidic and promote dissolution of other metals and elements that are hosted by subsurface rocks [Citation11]. In most gold mining facilities, S0 makes up most of the gold (Au) containing rocks together with other metal sulphite such as pyrite (FeS), sphalerite (ZnS), galena (PbS), chalcocite (Cu2S), Millerite (NiS), cinnabar (HgS), arsenopyrite (FeAsS) and chalcopyrite (CuFeS2). These compounds are often mined for the extraction of precious metals such as Au, silver (Ag), lead (Pb), iron (Fe), zinc (Zn) and copper (Cu) to be used commercially [Citation12–14]. To curb and combat the adverse effect of sulphate in the environment, reversal of the process is possible where S0 gets stripped from H2 and O2 and in the presence of dissolved metals, precipitation into their native forms can be forged [Citation15].
Sulphate reduction from SO42- to S0 can be carried out by either biological or chemical processes [Citation16,Citation17]. Under mesophilic conditions, this process is performed by a group of anaerobic microorganisms known as sulphate-reducing bacteria (SRB) classified according to their S0, O2 and carbon metabolisms. Sulphate reducers can reduce SO42- in either of the two pathways: dissimilatory or assimilatory growing as anaerobic, facultative anaerobic, autotrophic and/or heterotrophic bacteria [Citation16]. Based on the bioavailability of a suitable electron donor for the bacteria present in an optimized environmental condition for SO42- reduction, bacteria will use SO42- as an electron acceptor. With various electron donors usable by different groups of SRB, Liamleam and Annachhatre (2007) reviewed both organic and inorganic electron donors usable by SRB including but not limited to: acetate, lactate, ethanol, molasses and hydrogen [Citation18]. In the current decade where researchers are evaluating cost-effective methods in biotechnological processes, glycerol as one of the organic biodiesel wastes has been assessed to be valorized as an electron donor in bioremediation processes with success [Citation19]. With stoichiometric balancing of 1 mol of SO42- as electron acceptor, 0.67 mol of chemical oxygen demand (COD) or electron donor is required in optimized sulfidogenic condition for SRB to optimally reduce SO42- of which glycerol was observed to successfully be utilized by heterotrophic SRB [Citation18,Citation19].
Sulphate reducers have demonstrated wide tolerance to extreme conditions which destroy most bacteria such as pH below 4 and temperatures below 7°C and others above 60°C [Citation20]. Due to their tolerance to harsh environmental conditions, SRB have been applied with success in industrial wastewater treatments where influents contained high concentrations of SO42- and dissolved heavy metals [Citation21,Citation22]. Studies conducted by Kimura and co-workers (2005) reported growth and reduction of SO42- with heavy metal precipitation by pure SRB isolates at low pH between 3.8 and 4 [Citation23]. In this study, glycerol was used a carbon source with acetate produced as a secondary metabolite. The most vital factor considered in this study was maintaining the sulphate-reducing conditions which were anaerobic conditions in a closed system which further resulted in the precipitation of Zinc in a form of ZnS. In an open field where passive wastewater treatments are structure with SRB present as a consortium, fluctuations of physicochemical parameters are inevitable with some such as change in pH and temperature can exacerbate the toxicity of some reactive metabolites such H2S, organic compounds or metals that have potential to halt and inhibit SRB activity and growth.
Some transition metal ions are both toxic and essential for different bacterial groups including SRB which when present in required concentrations, microbial activities can be optimized to efficiently reduce contaminants in treatment systems [Citation10,Citation23]. Under unfavorable conditions such as extreme variation of pH and temperature, specific metabolic and biochemical mechanisms are prune to be affected and could hinder growth and activity of bacterial cells in a system hence their importance to be monitored and regulated. During such fluctuations, enzymes facilitating pathways such as SO42- reduction are affected in which most of their activation depends on the bioavailability of different transition metals such as Fe, Zn, Cu and Mn, among others [Citation10,Citation23]. Sulphate-reducing systems have been used efficiently as low-cost alternative to treat polluted water with high SO42- and transition metals concentration. Despite extensive studies conducted on these systems, a complete understanding of the synergistic effects of microbial inhibition factors on the survival mechanisms of SRB under dynamic growth is still missing. In order to clarify and add new information and scientific contribution to this subject, this paper reveals the behavior of SRB under the synergic exposure of varied pH and temperature conditions. A mathematical model formulated from the obtained kinetic data is presented and is usable for the prediction of the outcomes of a closed biological remediation system with SRB consortium applied as a biological entity.
2. Materials and methods
2.1. Site description and wastewater sampling
Three wastewater samples were collected from three mining sites in South Africa located in the Witwatersrand area depicted on the map below (). Site-1 and Site-3 are active mines with ongoing mining practice, and Site-2 is a reclaimed mining site with halted mining activities. Site-1 is an active open pit coal mine with daily extraction of coal and other valuable minerals from detonated rocks. The site is located in Mpumalanga province supplying coal to electricity generating enterprise in South Africa. Site-2 is a previously abandoned shaft coal mine that still generates mine drainage which made it a suitable study site for the rehabilitation of the site hence reclaimed by other companies. This site is located in the Eastern side of Johannesburg and contributed largely on both ground and surface water contamination. Site-3 is an active shaft gold mine situated in the Witwatersrand area which is still being mined daily. The mine is located in the North-West province of South Africa which is along the Witwatersrand basin which is known to be the largest gold deposits in the world with multiple gold mines accessing it in different locations [Citation25]. Names and precise locations of the mines cannot be revealed due to a non-disclosure agreement (NDA) that is in place. Water samples were collected according to the technique described by [Citation10] using sterile 25-L polyethylene drums. The following chemical parameters were measured on site: pH and total dissolved solids (TDS) using an ExStik®II probe; Model-EC500 (EXTECH Instruments). The samples were transported to the laboratory, dispensed into two 500-mL portions of sub-samples: one for hydro-chemical analysis and two for biological analysis and downstream batch experiments and then all were stored at 4°C until further analysis.
Figure 1. South African map indicating selected study sites. Site 1: active coal mine in Mpumalanga province; Site 2: reclaimed coal mine situated Eastern side of Johannesburg in Gauteng province and Site 3: active gold mine situated in the North West province of South Africa. (map adopted with modifications from [Citation24] (used with permission).
![Figure 1. South African map indicating selected study sites. Site 1: active coal mine in Mpumalanga province; Site 2: reclaimed coal mine situated Eastern side of Johannesburg in Gauteng province and Site 3: active gold mine situated in the North West province of South Africa. (map adopted with modifications from [Citation24] (used with permission).](/cms/asset/03432d92-4d91-43a4-afca-4783a5d75ef7/tcsb_a_2257388_f0001_oc.jpg)
2.2. Chemical wastewater analysis
At the laboratory, the three starting samples (site-1, −2 and −3) were further characterized for verification by determining pH, electrical conductivity (EC) and TDS using an ExStik®II probe Model EC500 (EXTECH-FLIR, Massachusetts, UK). Oxidation Reduction Potential (ORP) was determined using ExStik® probe Model REC300 (EXTECH-FLIR, Massachusetts, UK), and the measurements were corrected according to a standard H+ electrode. A portion of the three starting samples were filtered through a 0.22-µm filters using sterile syringes, acidified to a pH less than 2 with nitric acid (2% HNO3) and stored at 4°C until analysis. Elemental Ca, Mg, K, P, Na, Fe, Al, Mn, Ni and Zn concentrations were determined using an Inductively Coupled Plasma–Optical Emission Spectroscopy with Large Format, Programmable Array Detector (Prodigy High Dispersion ICP-L-PAD) at the Institute of Ground Water Studies (IGS), University of the Free State.
2.3. Microbial enrichments
The three mining wastewaters were individually used as inoculum (20% v/v) for enrichment of SRB using Postgate medium B (PSGM-B) with modifications that include the addition of glycerol rather than sodium lactate and adjusting the medium pH to 6.2 [Citation26,Citation27] in serum vials. All components of the medium were prepared in a 1 L volume with the following modifications: Seawater replaced with autoclaved tap water, carbon source: Sodium Lactate used for enrichments and later replaced with 5 mL/L of 60% glycerol and pH adjusted to 6.5 instead of 7–7.5 as it appears on the cited components. The 1 L medium was then aliquoted in 80-mL portions in 150-mL serum vials, sealed with a combination of Teflon-faced chlorobutyl stoppers and aluminium crimps (Bellco Glass, Vineland, N.J., U.S.) prior to autoclaving. Autoclaved media was then deoxygenated by purging O2-free N2 (Air Liquide, Alrode, S.A.) for 20 cycles with filter-sterilized Resazurin-HCl added as an O2 indicator during cultivations. Enrichments were conducted under anaerobic conditions for the acclimatization of anaerobic bacteria and elimination of most obligate aerobic bacteria through three passages from primary, secondary and tertiary subculturing. Each culture generation was maintained in a metabolic active state and viable through inoculating 10% of the growing culture into fresh medium every 20 days. After three generations of enrichments: primary (20 days), secondary (40 days) and tertiary (60 days), the three consortia were combined to obtain a single consortium of enriched SRB for downstream experiments. This consortium was kept viable by transferring the culture into fresh medium every 20 days throughout the experimental period.
2.3.1. Molecular analysis of the bacterial enrichment
The microbial community and diversity were determined by subjecting the culture to molecular analysis as done by [Citation10]. Genomic DNA was extracted from the culture using the Nucelos NucleoSpin® Soil kit (Macherey-Nagel, Germany) according to the manufacturer’s instructions. The quality (pure dsDNA concentration and ds/ssRNA contamination concentration) and integrity (DNA shearing) of the DNA were determined using a NanoDrop 3300 fluorospectrometer (Thermo Scientific, Waltham, MA, United States) and 1% agarose gel stained with ethidium bromide (EtBr), respectively. The extracted gDNA samples were subjected to Illumina MiSeq sequencing analysis at the Centre for Proteomic Genomic Research (CPGR), Cape Town, South Africa. The sequence libraries were prepared by amplifying approximately the 460 bp region located in the hyper-variable V3/4 region of the 16S rRNA gene with overhang Illumina adapter overhang nucleotide sequences [Citation28]. The obtained 16S rRNA gene sequence data were analyzed using QIIME v1.9.1 as described by [Citation29] with adopted modifications by [Citation30]. Analyses of the abundance tables were carried out using R v3.6.1 (www.r-project) (R Development Core Team, 2011) and the phyloseq package [Citation31].
2.4. Batch experiments
The sulphate-reduction activity under varied conditions were studied by batch experiments using SixFors bioreactors (INFORS AG CH-4103 Bottmingen/Switzerland). Each bioreactor was fitted with a hermetic system that controls pH, temperature, dissolved oxygen (DO), anti-foam injector and stirrer speed for optimal conditions during the experiments. For the batch experiments, the following parameters were monitored and determined as follows during dynamic experiments: Optical Density (OD600nm) measured using a spectrophotometer for monitoring bacterial growth. Sulphate (SO42-) concentrations were determined using a multiparameter portable colorimeter model DR900 (manufactured by HACH analytical) according to methods: 8051 USEPA SulfaVer 4 Method 2 to monitor their progressive SO42- reduction over time. Sulphide (H2S) concentrations were monitored to verify the biological SO42- reduction that yields H2S which dissolves in the media to either react with metals forming metal sulphides or eventually be released from the media as (H2S) gas. Sulphide concentrations were analysed using the methylene blue method according to the procedure described by [Citation32] with fresh reagents prepared every two weeks for accuracy of the method.
2.4.1. Microbial sulphate reduction tests
Sulphate reduction capabilities of the enriched anaerobic SRB consortium from the three sites were evaluated anaerobically in serum vials by inoculating the tertiary culture in PSGM-B medium containing increasing concentrations of sulphate (SO42- as Na2SO4, Merck): 20.82, 26.03, 31.23, 41.64 and 41.68 mmol/L in 200-mL serum vials (Wheaton, U.S.A.). Media preparations in the vials were done as previously described. The vials were incubated for 20 days, and SO4 concentrations were measured every third day using a DR900 spectrophotometer (Hach Analytical) according to the HACH method 8051 USEPA SulfaVer 4 Method. The evaluated culture was further maintained on Postgate medium and prepared in a 500 mL volume for the downstream experiments.
2.4.2. Evaluation of pH and temperature effects on sulphate reduction rates
The effects of pH and temperature on the sulphate reduction activity were evaluated by growing the consortium in PSGM-B with 10.42 mmol/L of SO42- in the following varied conditions: pH of 3.5 at 10°C and 25°C, and a pH of 6.2 at 10°C and 25°C. Each of the four bioreactors were stirred at 50 rpm and pH, OD, ORP, SO4 and total sulphide (ST = H2S/HS−/S2-) concentrations were determined daily for 20 days. During this experiment, pH was monitored in respective reactors using the pH electrodes incorporated in each reactor and maintained at pH 3.5 and 6 which were set levels by automated peristaltic pumps that titrated acid (0.5 M HCl) and base (0.5 M NaOH), respectively, when set thresholds were surpassed by pH margins on 1. The pH was monitored to adjusted and maintain the set pH of 3.5 and 6 in respective bioreactors as biochemical processes though the release of metabolites can alter the medium pH. Temperature was also monitored in each reactor by the temperature sensors and was maintained via an automated thermal jacket that adjusted the temperatures accordingly to keep the internal culture temperature at set 10°C and 25°C for the respective rectors. Dissolved O2 was monitored by an incooperated ORP probes for each reactor vessel and the Spurgers that bubbled O2-free N2 were activated when set 0% dissolved O2 status changed beyond 5%. The 0% dissolved O2 was maintained in order to keep the internal environment anaerobic and within sulfidogenic conditions. As the above internal maintenance was automated and samples from each reactor were collected daily through the sampling ports of each reactor for daily for further analysis of the following parameters: OD, ORP, SO42- and H2S. To monitor microbial growth, OD measurements were measured at 600 nm while ORP was measured immediately after sampling to monitor the reducing conditions within each reactor. As the reactor were operated to study SO42- reduction by the enriched SRB consortium, both SO42- and H2S concentrations were monitored to evaluate the effectivity of SO42-o reduction in the set conditions together with the concentration of produced H2S according to [Citation32]. All data obtained during the reactor operations were used to construct dynamic curves representing the fluctuations of each of the following parameters: pH, Temp, O2, DO, ORP, H2S and SO42- in each reactor.
2.5. Geochemical and mathematical modelling
The PHREEQC-2 geochemical speciation model [Citation33], in conjunction with the MINTEQ thermodynamic database [Citation34], was used. Zero, negative or positive SI values indicated that the solutions were in equilibrium, undersaturated and supersaturated, respectively, with respect to a solid phase of the samples. The synergic effects of temperature and pH were both integrated in a unique non-competitive inhibition equation, and the formulated mathematical model was tested to describe the biochemical reactions using the Lotka-Volterra equation [Citation35] also known as predator–prey equation proposed below.
3. Results
3.1. Hydro-chemical characterization of wastewater samples
The geochemical data recorded in revealed two types of mine drainages: acid mine drainage (AMD) and non-acidic mine drainage (NMD), based on the significant differences in pH and chemical composition of the wastewaters. The NMD collected at site-1 had pH over 7 and was found to contain low SO42- and metal concentrations as compared to the AMD samples collected from site-2 and site-3, whereh both had pH lower than 4 with elevated dissolved metals and SO42- concentrations.
Table 1. Physicochemical characteristics of mine drainages.
3.2. Phylogenetic characterization of the enriched consortium
According to the Illumina MiSeq sequencing results depicted in , the consortium predominantly consisted of Firmicutes (85% of the sequences) and Proteobacteria (11%), in which both phyla have been found to contain SRB [Citation36,Citation37].
3.3. Microbial sulphate reduction
The consortium was exposed to increasing sulphate concentrations (20.82, 26.03, 31.23, 41.64 and 41.68 mmol/L) to evaluate its sulphate-reducing capacity and to infer an inhibition effect of high sulphide concentrations on bacterial growth. Results revealed over 70% sulphate removal on average for experiments over a period of 20 days (). Sulphate was not completely reduced in all experiments even in the culture that contained the lowest SO42- concentration of 20.82 mmol/L.
Table 2. Sulphate reduction efficacy of SRB.
3.4. Adaptation dynamics of SRB under varied pH and temperature conditions
The consortium was exposed to pH of either 3.5 or 6.2 at 10 or 25°C. At an initial pH of 6.2 and a temperature of 25°C, 95% of the sulphate was reduced, with Eh dropping below −200 mV. During the experiment, the pH dropped to 4.61 and slowly increased to 6.17 indicating bacterial activity. The initial OD600nm of 0.14 increased to 0.75 indicating intense bacterial growth. Low quantities of H2S production were observed at the beginning of the experiment as SO4 reduction was minimal till after day 10 of incubation with a rapid yield of H2S (). According to H2S solubility at different temperatures diagram (), moderate H2S toxicity levels were expected due to higher temperature (25°C) reducing H2S toxicity and solubility, which then permitted efficient SRB activity.
Figure 3. Temporal evolution of pH, ORP (Eh), SO42- and S2- in pH and temperature experiments: a) conditions: pH = 6.2 and 25°C, b) conditions: pH = 6.2 and 10°C, c) conditions: pH = 3.5 and 25°C and d) conditions: pH = 3.5 and 10°C over 20 days period. e) reference graphs for biogeochemical solubility of hydrogen sulphide in water at different temperatures [Citation38] (used with permission) and f) Eh-pH diagram for the system S-O2-H2O at 25°C showing the fields of predominance of the aqueous species and of elemental sulphur (so) for ES(aq) = 10–3 mol/kg at aqueous/so boundaries [Citation39] (used with permission).
![Figure 3. Temporal evolution of pH, ORP (Eh), SO42- and S2- in pH and temperature experiments: a) conditions: pH = 6.2 and 25°C, b) conditions: pH = 6.2 and 10°C, c) conditions: pH = 3.5 and 25°C and d) conditions: pH = 3.5 and 10°C over 20 days period. e) reference graphs for biogeochemical solubility of hydrogen sulphide in water at different temperatures [Citation38] (used with permission) and f) Eh-pH diagram for the system S-O2-H2O at 25°C showing the fields of predominance of the aqueous species and of elemental sulphur (so) for ES(aq) = 10–3 mol/kg at aqueous/so boundaries [Citation39] (used with permission).](/cms/asset/26be401e-53ec-4df1-aeb1-2f5a9e543373/tcsb_a_2257388_f0003_b.gif)
The experiment with the following conditions: pH 6.2:10°C, pH 3.5:10°C and pH 3.5:25°C achieved 19%, 13% and 9% sulphate removal, respectively (), with an average Eh of −73 mV. At pH 6.2 and 10°C (), a rapid initial bacterial growth was observed from OD600 nm of 0.13 to 0.58. However, after this exponential growth, a stationery phase was maintained throughout the incubation period with a slight increase on the last day of the experiment. In the experiments with pH 3.5:10°C () and pH 3.5:25°C (), minimal bacterial growth was observed at the beginning of the experiments followed by a long stationary phase, which was overcome only on the last day with extreme effects of low pH in both experiments. Due to low Eh in both experiments with low pH (3.5), SO42- in a form of H SO42- dominated the system and exerted toxic effects on the growing bacteria hence low sulphate reduction (). Data collected from these experiments were used to formulate a non-competitive inhibition equation.
4. Discussion
4.1. Effect of pH and temperature on SRB consortium
From the physicochemical characteristics of the mine wastewaters recorded in , it was observed that pH in each in each sample became a factor in the dissolution of heavy metals in water. As expected in AMD characterized by low pH, there were higher concentrations of dissolved metals as compared to wastewater that had pH above neutral. The most prevalent heavy metal was Fe, known to be associated with AMD, and was observed to be 8.32 and 1.52 mmol/L in wastewater collected from Site-3 and Site-2, respectively, which had respective pHs of 2.9 and 3.4. This factor is supported by the same metal detected in low concentration of 0,0009 mmol/L in Site-1 wastewater that had a pH of 7.82. The same was observed with Zn and Ni in which both were detected in higher concentrations in acidic wastewaters [Citation10,Citation11]. The values of EC in both acid samples were beyond twofolds higher than in the neutral wastewater that had EC of 166. With the presented data, the two acidic sample represent typical AMD which according to the South African National Standards (SANS) limits for surface water, the wastewater should not be released into the environment prior to treatment [Citation10]. To further assess the level of contamination, the SANS limits have set maximum SO4− concentration at 2.6 mmol/L (250 mg/L) while Site-1 had 9,81, Site-2 had 12,56 and Site-3 had the highest concentration of 30,55 making it 12-folds higher than the acceptable limit. As an option of remediating SO42- from wastewater using bacteria, this paper provides insights on how bacteria can best be applied in controlled environments to mitigate the contamination.
Sulphate reduction as a complex process can be affected, depressed and hindered by changes in environmental parameters such as pH, temperature, some organic acids and sulphide species [Citation40,Citation41]. Most SRB grow optimally at pH values above 6 and temperatures between 25°C and 30°C [Citation42,Citation43], which are the conditions established in the first experiment of this study. Under these conditions, SO42-/H2S redox couples control the system, and the predominant reduced S0 compound was found to be H2S [Citation44]. Due to its structural similarities with H2O, it was hypothesized that aquaporins present in SRB may facilitate H2S transport across cell membranes similarly as water molecules. This would facilitate its binding capacity to the iron of cytochrome and other essential iron-containing compounds in the cell causing electron transport systems to cease activity [Citation45,Citation46].
Within the controlled stirred tank reactors, the enriched SRB consortium achieved over 95% of sulphate reduction in culture with initial sulphate concentration of 10.42 mmol/L of SO42- generating 3.15 mmol/L of H2S which was high enough to inhibit SRB activity (>3 mmol/L) [Citation44]. Nevertheless, this concentration did not seem to affect SRB activity but could have sufficiently been toxic for other (acidogenic and methanogenic) bacteria that coexist in the mixed population [Citation44,Citation47,Citation48]. Thus, it was favorable for the sulphate-reducing process to optimally occur within the sulfidogenic environment created by the reactor. At the beginning of the pH and temperature experiments, fluctuations in pH were observed with intense bacterial growth but low sulphate reduction activity. According to [Citation49], pH fluctuations and intense bacterial activity can be promoted by two phenomena: the saturation of CO2 in the medium that is generated when glycerol is catabolized (Equation 1) and the acidogenic process at low pH of 3.5 (Equation 2) which reveals a clear competition for carbon source that was observed in the first five days of the experiments. The competition for carbon source and the saturation of CO2 in the system promoted the later generation of bicarbonate that increased the pH of the system (Equation 3). Bertolino and co-workers (2014) [Citation27] reported that glycerol oxidation during sulphate reduction produce less alkalinity than lactate degradation (Equation 2 and 4) which justifies the pH values that could not exceed neutral values in these experiments when using glycerol as a carbon source at higher pH above 6. The H2S production was low at the beginning of the experiment which suggests that once the SRB started producing H2S, they out-compete other bacterial groups [Citation50]. This was supported by sudden rapid sulphate reduction rates after 10 days of the incubation in the culture grown in pH of 6.2 at 25°C.
Under high pH and low temperature (pH 6.2: 10°C), sulphide production from 19% sulphate reduction did not match the rapid bacterial growth observed in . ALthough the bacterial growth was minimal, its trend was similar to the batch experiment under higher parameter limit conditions (pH 6.2: 25°C) during the entire experiment. Lower temperature slowed down the metabolic activity of bacteria and increased the solubility of H2S which becomes toxic even at low concentrations [Citation51,Citation52]. Therefore, the alteration of bacterial activity after the rapid growth could be attributed to the toxicity of H2S present in solution despite its low concentration of 0.6 mmol/L.
Under low pH (3.5) conditions, the toxicity increased as a result of the bioavailability of organic acids and metals in solution which is later discussed. At low pH, carboxyl groups (volatile fatty acids) occur mainly in the undissociated form as organic acids. These toxic uncharged molecules can pass through the cell membrane and act as an uncoupler in the cytoplasm and, therefore, reduces SRB activity [Citation52]. The pH in the system dictates the speciation of organic compounds generated from glycerol oxidation by the mixed consortia. These volatile fatty acids under specific low pH would become protonated and toxic such as at pH 4, most of the acetate which is a product of glycerol oxidation, become acetic acid (pKa: 4.75). In its undissociated form, acetic acid (as many other small molecular weight acids) is toxic even at low concentration. The trend of the bacterial growth and the low sulphide produced in these experiments would be due to the inhibitory activity of biogenic compounds such as H2S and organic acids. According to the results, sulphate reduction activity and its prediction are regulated and depend on the synergistic effect of parameters such as pH and temperature which are crucial in such passive biosystems based on sulphate-reducing bacteria.
4.2. Formulation of temperature and pH effects on sulphate reduction into a model
In a passive biosystem that could be affected by environmental and seasonal oscillation of pH and temperature, the development of a mathematical model to simulate and predict the different biochemical reactions that occur in these systems became a complex task. Thus, to develop a generic and simple model able to estimate the dynamics of the biological sulphate-reducing activity was needed. With background of known mathematical models for biological sulphate reduction systems, a simulation of the synergic effect of temperature and pH was integrated in a unique non-competitive inhibition equation. The kinetics of microbial growth as monitored by optical density in this study was not considered in the model as the data did not match temporal evolution of the sulphate or sulphide as observed in the previous results due to the metal precipitates that interfered with OD of the microbial cells. The mathematical model considers describing the biochemical reaction with the Lotka-Volterra equation, which is also known as predator–prey equation. This was used to portray the dynamics of biological systems in which two species interact, one as a predator and other as prey. However, Alfred J. Lotka initially introduced this equation in the theory of autocatalytic chemical reactions in 1909 [Citation35]. In this section, we suggest a mathematical equation that could be used to portray this biochemical process in a closed system.
Let S[t] be the concentration of SO42- at time t and C[t] be a concentration of H2S at time t. While n is the number of days of the experiment. The H2S species predominated the system since the pH was 4 to 6. Therefore, it was selected and presented in the mathematical model as the sulphide reduced specie as follows:
Equation 5 represents the rate of change of S[t] as function of time:
where f (pH, T) is the contribution function of pH and temperature during the reaction. According to the experimental data, the influence of pH in the sulphate reduction rate was higher than the influence of the temperature, which was taken into consideration in this mathematical model. The function is generalized and can be constructed for any given reaction. In Equation 6, is the time delay due to acclimatization of bacteria for the sulphate reduction process to start. Equation 6 can therefore be reformulated into Equation 7 as follows:
With the integration of Equation 6 into 7, the determination of sulphate concentration (S[t]) at a set time can be archived by Equation 8 below where S[0] is the initial concentration of SO42- before the start of the reaction. According to the observed data, at the beginning of the reaction, there is no biogenic concentration of H2S from sulphate reduction of the initial concentrations, this implies C[0] = 0. The stoichiometric relation between H2S/SO42- is thus 1/3.
With consideration in a progressive reduction process, the end of the process would be indicated and proven by complete reduction of SO42- and that factor can be incorporated in the model using Equation 9 and Equation 10 where C[t] is represented.
Thus by solving Equations 9 and 10 using the classical method of ordinary differential equation, Equation 11 was obtained as the following solution for C[t] determination. From Equation 11, applying the initial and boundaries conditions of pH and temperature, the calculated yields Equation 12:
The final mathematical model of SO42- reduction to H2S is therefore suggested to be as shown in a complex model Equation 13 where all parameters are incorporated:
The above presented model was calibrated and validated using an experimental chemical data. However, minor deviations between experimental data and the mathematical model were observed as depicted in . According to [Citation16], one of the factors resulting in the self-oscillating dynamics observed in the trend of sulphate, sulphide, pH and ORP experiments was reported to be the bacterial competition for the same substrate. Nevertheless, data deviations do not affect the prediction and estimation of the trend of the main biochemical reactions in these biosystems as stoichiometric calculations support the model. Therefore, the described equation serves to easily predict the effectiveness of a bioreactor according to the variation of pH and temperature expected for each specific case which can be subsequently used for process optimization and control.
Figure 4. Proposed model with realistic temporal evolution of sulphate (SO42-) and sulphide (H2S) in a closed system.

In order to optimize and improve the accuracy of this model, more factors that may affect the effectivity of biogenic sulphate reduction including the repetition of the experiment m-times and evaluation of the trends of the m-sets of data should be performed. If the m-set of data have the same trend when different parameters are incorporated, then the model would predict and represent the actual activity of SRB in an in-situ bioremediation system. Otherwise, one will have to extract uncertainties from experimental data to obtain the real behavior of bacteria under varied conditions and factors including environmental conditions that changes spontaneously. The mathematical model proposed in this study is therefore a first millstone to formulating a model that serve to easily predict the sulphate-reducing activity in the biosystems for passive wastewater treatment.
5. Conclusions
This study showed that the efficiency of the sulphate-reducing process by sulphate-reduction bacteria is governed by environmental factors such as pH and temperature which require control and monitoring in a closed system. Specifically, it was confirmed that low pH exerts a higher inhibitory effect than low temperature with evidence that different SO species affect bacteria differently under varied environmental conditions. The synergistic effect of these two physicochemical parameters were observed to be controlling the speciation and solubility of inorganic compounds such as S0-reduced species (H2S/HS−) and organic acids such as acetic acid, which significantly decreased the sulphate reduction activity of the consortium. A unique non-competitive inhibition equation, integrating both the effect of temperature and pH, was formulated which can be used to predict the efficiency of bacteria enriched to be applied in a passive bioremediation system. This equation further facilitates the estimation of the effectiveness of sulphate-reducing biological systems under a range of pH and temperature which are known to affect all life forms also serving as growth-limiting factors in biological systems. Although the model fits well with the experimental data, it should be optimized further by additional experiments that encompasses other factors such as O2 concentration, specifically defined bacterial groups, metals associated with SO4 contamination in water, effects of synthesized metabolites such as acetic acids and different carbon sources applicable in bioremediation systems. Finally, it was possible to determine that optimizing environmental condition, such as pH and temperature through adjusting the physicochemical parameters using a stirred tank reactors, can induce the effectivity of a bioremediation systems. Application of the predictor model can be used to make decisions in planning and implementing bioremediation systems in pilot-scale experiments.
Author contributions
Conceptualization: E.v.H., J.C. and K.M.; Experimental work: K.M.; Data interpretation and analysis: K.M., E. vH., J.C., E.C.; Model formulation: A.A., J.C. and K.M.; Writing of the manuscript: K.M., J.C., E. vH., E.C., Z.K., M.M., J.U.; Project Administration: E.vH. Funding Acquisition: E.vH., and J.C. All authors have read and agreed to the published version of the manuscript.
Acknowledgments
The authors would like to acknowledge TIA for financial support and the involved departments at the University of the Free State (UFS), Central University of Technology, Free State (CUT), University of Kwa-Zulu Natal (UKZN), University of Pretoria (UP) where resources, infrastructure and equipment were used in conducting the experiments and preparing the manuscript. Authors finally acknowledge the three mining companies in South Africa for granting access to collect samples and the Institute of Groundwater Studies (IGS) at the UFS for assistance with chemical analysis of water samples.
Disclosure statement
No potential conflict of interest was reported by the author(s).
Data availability statement
Data used and presented in this study can be made available upon requests to the corresponding authors.
Additional information
Funding
References
- Santucci L, Carol E, Tanjal C. Industrial waste as a source of surface and groundwater pollution for more than half a century in a sector of the Río de la Plata coastal plain (Argentina). Chemosphere. 2018;206:727–464. doi: 10.1016/j.chemosphere.2018.05.084
- Moloantoa KM, Khetsha ZP, van Heerden E, et al. Nitrate water contamination from industrial activities and complete denitrification as a remediation option. Water. 2022;14(799):1–31. doi: 10.3390/w14050799
- Albrighta TP, Mutiibwa D, Gerson AR, et al. Mapping evaporative water loss in desert passerines reveals an expanding threat of lethal dehydration. PNAS. 2017;114(9):2282–2288. doi: 10.1073/pnas.1613625114
- Derakhshani R, Alipour M. Remediation of acid mine drainage by using tailings decant water as a neutralization agent in Sarcheshmeh copper mine. Res J Environ Sci. 2010;4(3):250–260. doi: 10.3923/rjes.2010.250.260
- Meyer JA, Casey NH. Exposure of potentially toxic trace elements in indigenous goats in the natural communal production system of the northern region of South Africa. South African J Animal Sci. 2004;34:219–222.
- Bhateria R, Jain D. Water quality assessment of lake water: a review. Sustain Water Resr Man. 2016;2(2):161–173. doi: 10.1007/s40899-015-0014-7
- Seckler D, Barker R, Amarasinghe U. Water scarcity in the twenty-first century. Int J Water Res Dev. 1999;15(1–2):29–42. doi: 10.1080/07900629948916
- Akhtar N, Shak MIS, Bhawani SA, et al. Various natural and anthropogenic factors responsible for water quality degradation: a review. Water. 2021;13(9):2660. doi: 10.3390/w13192660
- Hummer DR, Golden JJ, Hystad J, et al. Evidence for the oxidation of earth’s crust from the evolution of manganese minerals. Nat Commun. 2022;13(960):1–7. doi: 10.1038/s41467-022-28589-x
- Moloantoa KM, Khetsha ZP, Kana GEB, et al. Metagenomic assessment of nitrate-contaminated mine wastewaters and optimization of complete denitrification by indigenous enriched bacteria. Front Environ Sci. 2023;11:1–20. doi: 10.3389/fenvs.2023.1148872
- Dold B. Evolution of acid mine drainage formation in sulphidic mine tailings. Minerals. 2014;4(3):621–641. doi: 10.3390/min4030621
- Baker BJ, Banfield JF. Microbial communities in acid mine drainage. FEMS Microbiol Ecol. 2003;44(2):139–152. doi: 10.1016/S0168-6496(03)00028-X
- Baker BJ, Moser DP, MacGregor BJ, et al. Related assemblages of sulphate-reducing bacteria associated with ultra-deep gold mines of South Africa and deep basalt aquifers of Washington State. Environ Microbiol. 2003;5(4):267–277. doi: 10.1046/j.1462-2920.2003.00408.x
- Kalin M, Fyson A, Wheeler WN. The chemistry of conventional and alternative treatment systems for the neutralization of acid mine drainage. Sci Total Environ. 2006;366(2–3):395–408. doi: 10.1016/j.scitotenv.2005.11.015
- Matsumoto S, Ishimatsu H, Shimada H, et al. Characterization of mine waste and acid mine drainage prediction by simple testing methods in terms of the effects of sulfate-sulfur and carbonate minerals. Minerals. 2018;8(9):403. doi: 10.3390/min8090403
- Cassidy J, Lubberding HJ, Esposito G, et al. Automated biological sulphate reduction: a review on mathematical models, monitoring and bioprocess control. FEMS. 2015;33:1–31.
- Luptakova A, Kotulicova I, Macingova E, et al. Bacterial elimination of sulphates from mine waters. Chem Eng Trans. 2013;35:853–858.
- Liamleam W, Annachhatre AP. Electron donors for biological sulfate reduction. Biotechnol Adv. 2007;25(5):452–463. doi: 10.1016/j.biotechadv.2007.05.002
- Zhou X, Dorado AD, Gabriel X, et al. Mechanistic modelling of glycerol fermenting and sulfate-reducing process by granular sludge under sulfidogenic conditions. J Environ Chem Eng. 2022;10(3):1–12. doi: 10.1016/j.jece.2022.107937
- Kaksonen AH, Puhakka JA. Sulfate reduction based bioprocesses for the treatment of acid mine drainage and the recovery of metals. Eng Life Sci. 2007;7(6):541–564. doi: 10.1002/elsc.200720216
- Knoblauch C, Sahm K, Jørgensen BB. Psychrophilic sulfate-reducing bacteria isolated from permanently cold arctic marine sediments: description of desulfofrigus oceanense ge. nov., sp. nov., desulfofrigus fragile sp. nov., Desulfofaba gelida gen. nov., sp. nov., desulfotales psychrophilia gen. nov., sp. nov. And Desulfotalea artica sp. nov. Int J Bacteriol. 1999;49(4):1631–1643. doi: 10.1099/00207713-49-4-1631
- Sekiguchi Y, Muramatsu M, Imachi H, et al. Thermodesulfovibrio aggregans sp. nov. And thermodesulfovibrio thiophilus sp. nov., anaerobic, thermophilic, sulfate-reducing bacteria isolated from thermophilic methanogenic sludge, and emended description of the genus thermosulfovibrio. Int J Bacteriol. 2008;58(11):2541–2548. doi: 10.1099/ijs.0.2008/000893-0
- Kimura S, Hallberg KB, Johnson DB. Sulfidogenesis in low pH (3.8–4.2) media by a mixed population of acidophilic bacteria. Biodegradation. 2006;17(2):57–65. doi: 10.1007/s10532-005-3050-4
- McCarthy S. The impact of acid mine drainage in South Africa. South African J Sci. 2011;107(5/6):1–7. doi: 10.4102/sajs.v107i5/6.712
- van Eeden ES, Liefferink M, Durand JF. Legal issues concerning mine closure and social responsibility in the West Rand. TD: J Transdisciplinary Res Southern Africa. 2009;5(1):51–71. doi: 10.4102/td.v5i1.148
- Ghazy EA, Mahmound MG, Asker MS, et al. Cultivation and detection of sulfate reducing bacteria (SRB) in sea water. J Am Sci. 2011;7(2):604–608.
- Bertolino SM, Melgaco LA, Sá RG, et al. Comparing lactate and glycerol as a single-electron donor for sulfate reduction in fluidized bed reactors. Biodegradation. 2014;25(5):719–733. doi: 10.1007/s10532-014-9694-1
- Klindworth A, Pruesse E, Schweer T, et al. Evaluation of general 16S ribosomal RNA gene PCR primers for classical and next-generation sequencing based diversity studies. Nucleic Acids Res. 2013;41(1):e1–e1. doi: 10.1093/nar/gks808
- Caporaso JG, Kuczynski J, Stombaugh J, et al. QIIME allows analysis of high throughput community sequencing data. Nat Methods. 2010;7(5):335–336. doi: 10.1038/nmeth.f.303
- Cason ED, Mahlomaholo BJ, Taole MM, et al. Bacterial and fungal dynamics during the fermentation process of sesotho, a traditional beer of Southern Africa. Frontier In Microbiol. 2020;11(1451):1–14. doi: 10.3389/fmicb.2020.01451
- McMurdie PJ, Holmes S, Watson M. Phyloseq: an R package for reproducible interactive analysis and graphics of microbiome census data. PLoS One. 2013;8(4):1–11. doi: 10.1371/journal.pone.0061217
- Fonselius S, Dyrssen D, Yhlen B. Determination of hydrogen sulphide. In: Grasshoff K, Kremling K Ehrhardt M, editors. Methods of seawater analysis. Weinheim, New York, Chichester, Brisbane, Singapore: Wiley-VCHToronto; 2007. pp. 91–108. doi: 10.1002/9783527613984.ch5
- Parkhurst DL, Appelo CAJ. PHREEQC (version 3)-A computer program for speciation, batch-reaction, one-dimensional transport, and inverse geochemical calculations. Model Tech B. 2013;6:497. 99–4259.
- Nicholas T. Loux, David S. Brown, Claudia R. Chafin, Jerry D. Allison and Sayed M. Hassan. Chemical speciation and competitive cationic partitioning on a sandy aquifer material. Chemical Speciation & Bioavailability. 1989;1:3. 111–125. doi: 10.1080/09542299.1989.11771987
- Lotka AJ. Contribution to the theory of periodic reactions. J Phys Chem. 1909;14(3):271–274. doi: 10.1021/j150111a004
- Kushkevych I, Dordevic D, Vitezova M, et al. Environmental impact of sulfate-reducing bacteria, their role in intestinal bowel diseases, and possible control of bacteriophages. Appl Sci. 2021;11(2):735. doi: 10.3390/app11020735
- Nielsen PH, Raunkjær K, Hvitved-Jacobsen T. Sulfide production and wastewater quality in pressure mains. Water Sci Technol. 1998;37(1):97–104. doi: 10.2166/wst.1998.0024
- Langmuir D. Aqueous environmental chemistry. 1st ed. Upper Saddle River, N.J: Prentice Hall; 1997. p. 445.
- Moosa S, Nemati M, Harrison STL. A kinetic study on anaerobic reduction of sulphate, part II: incorporation of temperature effects in the kinetic model. Chem Eng Sci. 2005;60(13):3517–3524. doi: 10.1016/j.ces.2004.11.036
- Rzeczycka M, Blaszczyk M. Growth and activity of sulphate-reducing bacteria in media containing phosphogypsum and different sources of carbon. Polish J Environ Stud. 2005;14(6):891–895.
- Al-Zuhair S, El-Naas M, Al-Hassani H. Sulfate inhibition effect on sulfate reducing bacteria. J Biochem Tech. 2008;1(2):39–44.
- Sawicka JE, Jørgensen BB, Brüchert V. Temperature characteristics of bacterial sulfate reduction in continental shelf and slope sediments. Biogeosciences. 2012;9(8):3425–3435. doi: 10.5194/bg-9-3425-2012
- Okabe S, Nielsen PH, Characklis WG. Factors affecting microbial sulfate reduction by Desulfovibrio desulfuricans in continuous culture: limiting nutrients and sulfide concentration. Biotechnol Bioeng. 1992;40(6):725–734. doi: 10.1002/bit.260400612
- Shimada T. Factors affecting the microclimate pH in rat jejunum. J Physiol. 1987;392(1):113–127. doi: 10.1113/jphysiol.1987.sp016772
- Mathai JC, Missner A, Kugler P, et al. No facilitator required for membrane transport of hydrogen sulfide. Proc Natl Acad Sci. 2009;106(39):16633–16638. doi: 10.1073/pnas.0902952106
- Omil F, Lens P, Hulshoff Pol L, et al. Effect of upward velocity and sulphide concentration on volatile fatty acid degradation in a sulphidogenic granular sludge reactor. Process Biochem. 1996;31(7):699–710. doi: 10.1016/S0032-9592(96)00015-5
- Visser A, Hulshoff Pol LW, Lettinga G. Competition of methanogenic and sulfidogenic bacteria. Water Sci Technol. 1996;33(3):99–110. doi: 10.2166/wst.1996.0062
- Dinkel VG, Frechen FB, Dinkel AV, et al. Kinetics of anaerobic biodegradation of glycerol by sulfate-reducing bacteria. Appl. Biochem Microbiol. 2010;46(7):712–718. doi: 10.1134/S0003683810070069
- Maillacheruvu KY, Parkin GF, Peng CY, et al. Sulfide toxicity in anaerobic systems fed sulfate and various organics. Water Environ Res. 1993;65(2):100–109. doi: 10.2175/WER.65.2.2
- Lettinga G, Rebac S, Zeeman G. Challenge of psychrophilic anaerobic wastewater treatment. Trends Biotechnol. 2001;19(9):363–370. doi: 10.1016/S0167-7799(01)01701-2
- Auvinen H, Nevatalo LM, Kaksonen AH, et al. Low-temperature (9°C) AMD treatment in a sulfidogenic bioreactor dominated by a mesophilic desulfomicrobium species. Biotechnol Bioeng. 2009;104:740–751. doi: 10.1002/bit.22434
- Ghose TK, Wiken T. Inhibition of bacterial sulphate-reduction in presence of short chain fatty acids. Physiol Plant. 1995;8(1):116–135. doi: 10.1111/j.1399-3054.1955.tb08965.x