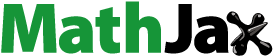
ABSTRACT
Discrimination of the catalytic ability of heterogeneous biochar components is often challenging. Herein, a sewage sludge-derived biochar (SDBC) was prepared to activate peroxydisulfate (PDS) for tetracycline (TC) degradation. To verify the contribution of different carbon components, SDBC was bleached with NaClO2 and CH3COOH to remove noncondensed aromatic carbon (NAC) contained in biochar, which was confirmed by 13C Nuclear Magnetic Resonance. The batch degradation experiment revealed that NAC removal decreased TC degradation by SDBC from 84.1% to 33.2% within 2 h, indicating its significant role in PDS activation. The quenching and electron paramagnetic resonance experiments suggested a very minor contribution of radical pathway in TC degradation. Instead, the electron transfer pathway predominated TC degradation mechanism as inferred by electrochemical tests. This is likely ascribed to formation of a biochar-PDS metastable complex, facilitating electron transfer from tetracycline-like compounds. An X-ray photoelectron spectroscopy confirmed that the percent of graphitic N in SDBC decreased after the degradation reaction, which suggested graphitic N is an important active site in biochar. Besides, acid-washed SDBC did not change TC degradation behavior excluding significant contribution of minerals in SDBC to PDS activation. Thus, the roles of biochar components in catalyzing PDS were quantified for the first time, proving insight for selection and manipulation of biochar in catalyzing PDS in environmental application.
GRAPHICAL ABSTRACT

Highlights
Noncondensed aromatic carbon in biochar is critical for activating peroxydisulfate.
Direct electron transfer is the primary pathway for biochar to degrade tetracycline.
The metastable graphitic N-PDS complex was dominant active sites.
The catalytic ability of biochar is well maintained over a wide pH range.
1. Introduction
Antibiotics such as tetracycline have been abusively used in pharmaceuticals and poultry animal husbandry. Concerns have since been raised regarding excessive discharge of antibiotics into soil and water environments because over-accumulation of antibiotics could undermine food safety and native microbial communities [Citation1–3]. However, the recalcitrant nature of the aromatic ring molecular structure has conferred tetracycline-like antibiotics good stability in natural aqueous conditions [Citation4]. To resolve this, Fenton (like) oxidation, as a common advanced oxidation process (AOP) has been considered as an efficient practice to enhance degradation of antibiotics in water to less-toxic intermediates or even carbon dioxides in recent years [Citation5–7]. Persulfate (PS) has demonstrated as a more prevailing oxidant over traditional hydrogen peroxides in AOP technique because of its high redox potential and better applicability over a wide pH range [Citation8–10]. For better degradation performance, some catalysts such as metal (oxides) and carbon-based materials are designed to activate PS to yield more active substances, such as hydroxyl radical (•OH), sulfate radical (SO4•−), superoxide radical (O2•−) and singlet oxygen (1O2) [Citation11–13].
Biochar, a pyrogenic carbonaceous material evolved from decomposition of organic materials under oxygen-limited conditions, comprises a range of different-structured carbon moieties such as condensed aromatic carbon, non-condensed carbon, amorphous and aliphatic carbon, as well as inorganic components such as minerals [Citation14]. Biochar has emerged as a low-cost and efficient catalyst for PS activation, resulting from such active moieties as aromatic carbon, noncondensed carbon with abundant functional groups (hydroxyl (−OH), carbonyl (C=O), and carboxyl (−COOH) nitrogen (N) bonded to carbon) and inorganic metal (oxide) components [Citation15,Citation16]. The intimate association of PS activation ability with active moieties of biochars has been evidenced by many previous works. For example, our recent work showed that N-functionalized pinewood derived biochar is more than five-fold efficient over pristine BC with respect to PS activation, in which pyridinic N as a dominant active N species enhanced generation of singlet oxygen and direct electron transfer [Citation17]. Another works doping biochars with single-atom manganese and Cu0/Fe3O4 have associated the enhanced PS activation with impregnated metals [Citation18,Citation19]. That is, the PS activation ability varied according to elemental compositions and carbon structure of biochars. Not surprisingly, these active moieties vary considerably between condensed aromatic carbon and noncondensed carbon moieties of biochar [Citation14], and it is thus anticipated that these moieties may be characterized with varied PS activation ability. However, there is no consensus on contribution of different active moiety of biochar on PS activation.
Sewage sludge is the byproduct of water treatment process with abundant organic substances, toxic elements and even harmful microorganisms. Compared to some common methods for disposal of sewage sludge, pyrolysis is recognized as a promising resolution from the resource utilization perspective. Because of innate heterogeneity in composition, the sludge-derived biochars is characterized by more diverse carbon structure and a larger quantity of inorganic minerals such as nitrogen, phosphorus and metals than plants derived biochars [Citation20,Citation21]. To date, the significance of each biochar component on PS catalysis has yet discriminated, although the condensed aromatic carbon is reported more important in retention of bisphenol A than noncondensed carbon does as a result of the high-adsorption domain and linear adsorption of the condensed aromatic carbon [Citation14]. Based on the composition of functional moiety in different structured biochars as discussed above, we hypothesized that the non-condensed carbon rich in carbon and nitrogen-bearing functional groups in sewage-sludge biochar may dominate the PS activation with unneglected contribution from inorganic components, in which these components may act via various mechanisms.
To test above hypothesis, the noncondensed aromatic carbon and inorganic minerals of sludge biochar was selectively removed to discriminate their roles in PS activation [Citation14]. Besides, the carbon structure in biochar inherited from the precursor feedstock is known to be enormously influenced by the pyrolysis temperature. For example, the carbon becomes more condensed as pyrolysis temperature rises, resulting in increased percentage of condensed aromatic carbon in high-temperature biochars [Citation14]. Herein, the sewage sludge-derived biochar was prepared at high temperature (800°C) to minimize the content of noncondensed carbon so that its importance can be more evidently visualized (see supporting information for more information). Overall, the specific objectives of this work were to (1) characterize the carbon structure and major property of pristine and bleached biochars and (2) evaluate the PS catalytic ability and associated mechanisms of different components of sewage sludge derived biochars.
2. Materials and methods
2.1. Reagents
The sewage sludge was obtained from a sewage treatment plant in Yangzhou, China. Sodium peroxydisulfate (Na2S2O8), furfuryl alcohol (FFA), tetracycline (TC), 5−dimethyl −1−pyrroline−N−oxide (DMPO) and 2,2,6,6 −Tetramethyl −4−piperidone (TEMP) were purchased from Sigma-Aldrich. Methanol (MeOH), Tert-butanol (TBA) and p-benzoquinone (BQ) were obtained from Sinopharm Chemical Reagent Co., Ltd. Sodium chlorite (NaClO2) and acetic acid (CH3COOH) (analytical grade reagents) were purchased from Aladdin Bio-Chem Technology Co. Deionized (DI) water (18.25 MΩ cm−1) was used for the preparation of aqueous solutions.
2.2. Preparation of biochars
The sewage sludge collected was washed three times with DI water and oven dried at 105°C for 24 h. The dried sludge was then transferred to a tube furnace and heated to 800°C under constant nitrogen atmosphere at a heating rate of 5°C min−1, which was maintained for 2 h. The obtained biochar sample was ground into powder and passed through a 100-mesh sieve (particle size <0.15 mm), and denoted as SDBC. To remove the noncondensed aromatic carbon (NAC) moieties, the condensed carbon counterpart was bleached following methods described previously [Citation14]. Briefly, SDBC (1 g) was bleached continuously for 5 h in a oxidizing solutions with 10 g of NaClO2, 10 mL of CH3COOH and 100 mL of deionized water. The bleaching procedure was repeated twice to ensure complete removal of noncondensed carbon, and the resulting biochar was denoted as BSDBC. To remove minerals in biochars, SDBC was soaked in 1 M HCl solution and stirred at room temperature (25 ± 2°C) for 24 h. The acid-washed biochars were named as HSDBC. All biochar samples were then washed with deionized water and freeze-dried 24 h [Citation22].
2.3. Characterization
13C NMR spectroscopy analysis was performed with a solid-state NMR spectrometer (AYANCE III 400 MHz WB, Bruker, Germany) and operated at a 13C frequency of 12 kHz to obtain structural information of pristine and bleached biochars. The surface elemental composition and functional groups of SDBC and BSDBC were characterized using X-ray photoelectron spectroscopy (XPS, ESCALAB 250Xi, Thermo, U.S.A.). The structural defects of the biochars were identified by a Raman spectroscope (INVIA REFLEX 12–80000) with the laser excitation of 532 nm. The crystal structures of SDBC and BSDBC were acquired using an X-ray diffraction (XRD) analysis with a Cukα radiation source (λ = 1.5418 Å) in the diffraction angles between 10–80° (Ultima IV X-Ray Diffractometer, Rigaku Corporation, Japan). The electrochemical properties of SDBC and BSDBC catalysts were tested with an electrochemical workstation (CHI660e, CH Instruments, China). The reactive oxygen species were tested by the electron paramagnetic resonance (EPR) (A300–10/12, Bruker, Germany), in which DMPO was used to trap sulfate radical (SO4•−) and hydroxyl radical (•OH), and TEMP was adopted to analyze singlet oxygen (1O2). The surface functional groups of biochars before and after bleaching were analyzed with a Fourier Transform infrared spectrometer (FTIR) (Cary 610/670, Varian, U.S.A.). Elemental content of C, N, O in SDBC and BSDBC was analyzed by an elemental analyzer (Vario EL Cube, Elementar, Germany). The Brunauer Emmett Teller (BET) specific surface areas and pore volumes of biochars were obtained using ASAP 2460 analyzer (Micromeritics, U.S.A.).
2.4. Catalytic degradation of TC
The batch TC degradation experiments were carried out in a 250 mL conical flask at room temperature. Specifically, 20 mg of catalysts were added to 100 mL of TC solution (20 mg L−1), and the suspension was agitated with a magnetic stirrer for 120 min until the sorption equilibrium was reached. Thereafter, 20 mg of sodium peroxydisulfate (PDS) was added to above suspension to initiate the catalytic degradation reaction. 1 mL of subsamples was collected at 5, 15, 30, 60 and 120 min, and instantly filtered through a 0.22 μm filter. The filtrate was added to a centrifuge tube containing methanol in order to quench the catalytic reactions. The absorbance of the sample was measured at 357 nm with an UV spectrophotometer (SG23-SP-2102UVPC-V). For experiments under different pH conditions, HCl (0.1 M) or NaOH (0.1 M) were used to adjust solution pH to the appropriate pH. For experiments under different anionic conditions, 10 mM NaCl, NaHCO3 and Na2HPO3 were added respectively to simulate experiments under different anionic conditions. In the stability experiment, the catalysts were tested for three consecutive cycles. After each run, the biochar samples were collected and washed with DI water for 3–4 times and saved for subsequent analysis.
3. Results and discussion
3.1. Characterization of bleached biochar
It is observed by 13C NMR spectra that bleaching treatment greatly altered carbon structure of SDBC. As expected, the SDBC comprised predominately aromatic carbon (108–165 ppm) and minor NAC such as aliphatic carbon (0–108 ppm) and other componentS with COOH and C-O groups [Citation14] (). After bleaching, the aromatic carbon remained but slightly decreased in its intensity, and the noncondensed carbon components were almost completely removed.
Similar to previous observations, the elemental and spectral analysis suggested that the sludge-derived biochar is rich in inorganic elements such as Si, cations and phosphorus (). The increased aromaticity after bleaching was affirmed by the greater H/C ratios of BSDBC (). A greater reduction of H concentration and an increase in the H/C value of BSDBC suggested bleaching could mainly selectively remove the noncondensed carbon in biochars. Besides, the bleaching procedure could remove metal and nonmetal elements partially, which decreased by 19.251%, 32.481%, 29.098%, 6.954%, 25.264% for Ca, Mg, Fe, Al and P, respectively ().
Table 1. Elemental composition and pore characteristics of SDBC and BSDBC.
Table 2. The content of major nonvolatile elements in SDBC and BSDBC.
Brunauer – Emmett–Teller (BET) analysis indicated surface area and pore volume of BSDBC decreased from 48.82 m2 g−1 to 45.53 m2 g−1 and 0.115 cm3 g−1 to 0.103 cm3 g−1, respectively, as compared to SDBC (). This is possibly due to the cracking of the micropores during the removal of the NAC and the incorporation of oxygen-containing functional groups [Citation14,Citation23]. The SEM results (Fig. S1) can also intuitively show that the pores of the sample after bleaching were reduced, which consolidated with the BET results.
Alteration of surface functional groups due to bleaching was revealed by XPS and FTIR spectra. As per high-resolution C1s XPS spectrum of SDBC, the characteristic peaks at 284.6, 287.2 and 288.5 eV, corresponding to C-C, C=O and O=C-O, respectively, were enhanced to different extents after bleaching () [Citation24]. Similarly, FTIR analysis suggested -OH, C=O, C=O-C functional groups of BSDBC were more intense than those of SDBC () [Citation23]. This indicated the carbon oxidation as a result of bleaching contributed to enrichment of oxygen-bearing functional groups. Besides, the peaks associated with Fe-O and Si-O was observed in both biochars, which were enhanced in BSDBC relative to SDBC.
Figure 2. C1s XPS spectra of SDBC (a), BSDBC (b) and SDBC after degradation test (c); N1s XPS spectra of SDBC (d), BSDBC (e) and SDBC after degradation test (f).

Carbon defects can be created at harsh conditions such as high temperature and oxidative conditions. Defects in biochar after bleaching were analyzed by a Raman spectroscopy. The D band at 1345 and 2869 cm−1 corresponds to defective sites and the G band at 1604 cm−1 represents the crystallinity of graphite, whereas the ID/IG ratio can indicate the graphitization degree of biochars () [Citation25,Citation26]. The results showed that ID/IG ratio of BSDBC (0.906) was remarkably higher than that of SDBC (0.816), which suggested the formation of defect was promoted during the removal of the NACs. This phenomenon was confirmed by the EPR system, which suggested that the peak at 3315 Gauss, an indication of free electrons [Citation17], was several folds greater than pristine SDBC after beaching ().
X-ray diffraction (XRD) patterns of SDBC and BSDBC (Fig. S2) presented a strong SiO2 peak for both SDBC and BSDBC. The similar XRD patterns showed that bleaching had little effect on the graphite structure of biochar.
3.2. Evaluation of the SDBC and BSDBC performance on PDS activation
The PDS activation ability by SDBC and BSDBC was inferred by TC degradation ability in aqueous solution. The direct oxidative degradation of TC induced by PDS was negligible (). Within first 2 h prior to PDS introduction, the TC removal was ascribed to adsorption by the adsorbents. The TC adsorption by SDBC (29.33%) was lower than BSDBC (25.18%), which was probably due to diminished specific surface area and pore volume as a result of pore collapse after bleaching [Citation27]. TC adsorption kinetics showed that both SDBC and BSDBC demonstrated fast sorption kinetics which reached adsorption equilibrium in 60 minutes (Fig. S3). There were no significant differences for TC sorption between SDBC and BSDBC, implying beaching treatment would not change the TC adsorption capacity of SDBC. Two hours after sorption process, PDS was added to the system to induce the oxidative degradation reaction. After 2 h degradation, 84.09% and 33.18% of TC were degraded by SDBC and BSDBC, respectively, which clearly indicated bleaching treatment remarkably compromised the catalytic performance of SDBC. Since bleaching can remove NAC component of biochars, it can be inferred that the catalytic ability of SDBC is mainly attributed to the NAC moiety.
Figure 4. TC degradation in different systems (a); EPR signals detected in (b) SDBC/PDS and (c) BSDBC/PDS systems at 10 min by using DMPO and TEMP as spin trapping agents; effects of (d) water matrices and (e) solution pH on the degradation of TC in the SDBC/PDS and BSDBC/PDS systems; reusability experiments (f). Experimental conditions. ([PDS] = 0.2 g L−1, [SDBC] = [BSDBC] = 0.2 g L−1, [DMPO] = [TEMP] = 10 mM, initial pH = 6.2, and T = 25 ± 2°C).
![Figure 4. TC degradation in different systems (a); EPR signals detected in (b) SDBC/PDS and (c) BSDBC/PDS systems at 10 min by using DMPO and TEMP as spin trapping agents; effects of (d) water matrices and (e) solution pH on the degradation of TC in the SDBC/PDS and BSDBC/PDS systems; reusability experiments (f). Experimental conditions. ([PDS] = 0.2 g L−1, [SDBC] = [BSDBC] = 0.2 g L−1, [DMPO] = [TEMP] = 10 mM, initial pH = 6.2, and T = 25 ± 2°C).](/cms/asset/ccbe4d14-b368-42be-bc27-c5eb3ee69ce1/tcsb_a_2267755_f0004_oc.jpg)
In general, sludge-derived biochar is rich in metals which is considered an active component to activate PDS and produce free radicals [Citation28]. To quantify the contribution of metals in PDS activation, SDBC was acid-washed repeatedly to remove metals in the pristine biochar. Upon PDS introduction, 80.46% TC was degraded by the metal-depleted biochar (HSDBC), which indicated that metal component in the SDBC exhibited minor contribution to PDS activation. This is probably due to the low availability of metal species that is active in PDS catalysis. After reactions, very low concentration of Fe was observed in solutions (), although a relatively high amount of leached Ca, Mg and Al was found which is inefficient in PDS activation [Citation29].
Table 3. Concentration of elements in solution after reaction.
3.3. Mechanistic insights into PDS activation by SDBC and BSDBC
3.3.1. Identification of reactive oxygen species
A quenching experiment was carried out to explore the role of main reactive species that involved in the degradation process. Different scavengers were added to the reaction solutions to discriminate the contribution of potential reactive species. For example, MeOH, TBA, BQ and FFA were considered efficient scavengers for hydroxyl (•OH) and sulfate (SO4•–) radicals, •OH radical, superoxide radical (O2•–) and singlet oxygen (1O2), respectively [Citation30–32]. The results showed that free radicals (•OH, SO4•–, O2•–) and 1O2 barely participated in the degradation process, as they affected degradation to a minimal extent (Fig. S4).
For validation purpose, EPR analysis was performed to detect the ROS responsible for the TC degradation using two spin-trapping agents (i.e. DMPO and TEMP). As depicted in , SDBC and BSDBC did not produce SO4•– and •OH signals in the presence of DMPO. Besides, 1O2 peaks were observed with roughly similar intensity in systems with and without catalysts (SDBC and BSDBC), which likely resulted from the oxidation of TEMP. That is, both catalysts were unable to catalyze formation of 1O2 and ROS such as SO4•– and •OH (). The EPR result agreed well with the quenching experiment, excluding the participation of 1O2 and ROS in TC degradation. Regarding BSDBC, the negligible TC degradation after PDS introduction is an indication of its poor catalytic ability. It is concluded that radical pathway or 1O2 contributed minorly to TC degradation.
3.3.2. PDS activation mechanism
It is speculated that the direct electron transfer may be a dominant mechanism for SDBC to activate TC degradation. Electrochemical tests were further performed to demonstrate the roles of electron transfer in TC degradation. The charge transfer between SDBC/PDS and TC molecules was studied by the timing amperometry (). After the addition of PDS, the electrical currents increased which is likely due to formation of metastable complex between the active sites on SDBC and PDS. Right after TC was added to the system, the electrical currents increased abruptly possibly resulting from the electron transfer from TC to biochar-PDS metastable complexes [Citation33]. This observation collaborated with several previous work which reported that the metastable complex derived from biochar moiety could complex with PDS and thus enhance degradation of organic contaminants [Citation34,Citation35]. In contrast, a much weaker change in the electrical currents was observed for BSDBC with depleted NAC component, possibly because the bleached biochar was substantially less capable to complex with PDS.
C and N bearing functional groups were affirmed previously as the dominant moieties in biochars to potentially complex with PDS. Herein, an XPS analysis was performed to identify the moiety of biochar that could bind with PDS. The N1s peak was mainly deconvoluted into pyridinic, pyrrolic and graphitic peaks, which changed significantly before and after bleaching (). After bleaching, the percent of graphitic N in biochar decreased sharply from 48.02% to 6.31%, but pyrrolic N increased from 23.05% to 50.03%. Considering very limited catalytic ability of bleached biochar (BSDBC), it is assumed that graphitic N may be the active sites to complex PDS. The spent SDBC after reaction with PDS was also analyzed with XPS, which showed N1s underwent a substantial change. After degradation reaction, the graphitic N in SDBC decreased from 48.02% to 16.44%, but pyrrolic N increased from 23.05% to 67.82%, likely because graphitic N acted as Lewis alkaline sites to accept electrons from TC during catalytic oxidation [Citation36]. To conclude, graphitic N in NAC is vital to accept electron from TC, which facilitated direct transfer between TC and biochar-PDS complex (Eqs. 1–2).
3.4. Catalytic performance evaluation
3.4.1. Effect of the pH
The pH of polluted environment may vary in different environmental conditions, which may significantly affect the degradation behaviors of contaminants in practical application. In an effort to testify the applicability of the as-prepared catalysts across different pH conditions, the degradation performance was evaluated within the commonly encountered environmental pH ranges, e.g. pH 3–9. The results showed that TC sorption and degradation kinetics decreased as the solution pH increased from 3 to 9. This may be due to the increased surface negativity of SDBC and TC deprotonation, which enhanced the electrostatic repulsion between PDS and TC [Citation34]. However, the overall TC removal efficiency did not vary significantly between pH 3–7, although the TC removal was decreased from 83.031% to 74.759% at pH 9 (). Therefore, an excellent TC removal efficiency by SDBC remained over a wide pH range (from 3.0 to 9.0), which is advantageous for advanced oxidation systems [Citation37].
3.4.2. Effects of inorganic anions
Chloride ions (Cl−), phosphate ions (PO43-) and bicarbonate ions (HCO3−) are the main inorganic anions in the aquatic environment, which may have a certain impact on the removal of organic pollutants in wastewater. Herein, Cl−, PO43- and HCO3− with a concentration of 10 mM were added to the TC solution separately to evaluate their effects on TC sorption and degradation in the SDBC/PDS system. As shown in , the addition of Cl− had little effect on the degradation of TC, whereas PO43- and HCO3− could inhibit TC degradation by 10.975% and 12.338%. The greater inhibitory effects of CO32-, HCO3−, PO43- and HPO42- could be ascribed to their ability to increase solution pH to around 8, which inhibits the sorption of TC and thus compromise electron transfer from TC to metastable complex [Citation29,Citation34]. Overall, the catalysts maintained reasonably good TC removal efficiency in wastewater with high ionic strength.
3.4.3. The reusability and stability
The reusability and stability of SDBC were also evaluated for three consecutive cycles. It showed the degradation of TC by SDBC decreased to 47.50% and 27.12% after the second and third cycle, respectively (). This may result from the coverage of active sites by degradation intermediates or loss of the metastable complex due to rinsing process [Citation38]. Considering excellent TC degradation ability by SDBC, the unfavorable reusability of the catalysts could be compensated by easy availability of raw materials and simple synthesis method. Overall. sewage sludge-derived biochars demonstrated good TC degradation ability in wastewater with different background electrolyte and under various pHs.
4. Conclusions
Herein, the NAC was selectively removed from the sludge-derived biochars. NAC removal undermined the PDS catalytic capacity of sludge-derived biochars roughly by 51%. However, depletion of metals barely compromised the PDS activation by SDBC. Thus, the NAC is a vital component for PDS activation in SDBC. The quenching experiment and EPR determination proved that free radicals and 1O2 were not involved in the degradation process. Instead, the direct electron transfer predominated the TC degradation by SDBC after PDS was introduced as per electrochemical test. This work highlighted the roles of different carbon components in biochars for PDS activation and provided an alternative for utilization of sewage sludge in advanced oxidation techniques.
4.1. CRediT authorship contribution statement
Xianni Yang: Conceptualization, Investigation, Original draft preparation; Investigation, Data analysis, and Discussion; Xuemin Xu: Materials, Methodology, Investigation; Yanyan Lu: Writing-reviews and editing; Shengsen Wang: Conceptualization, Proofreading, Writing-reviews and editing, Funds acquisition.
Supplemental Material
Download MS Word (840.2 KB)Disclosure statement
No potential conflict of interest was reported by the author(s).
Data availability statement
The authors declare that they have no known competing financial interests or personal relationships that could have appeared to influence the work reported in this paper.
Supplementary material
Supplemental data for this article can be accessed online at https://doi.org/10.1080/26395940.2023.2267755
Additional information
Funding
References
- Ao X, Sun W, Li S, et al. Degradation of tetracycline by medium pressure UV-activated peroxymonosulfate process: influencing factors, degradation pathways, and toxicity evaluation. J Chem Eng. 2019;361:1053–537. doi: 10.1016/j.cej.2018.12.133
- Xin S, Huo S, Zhang C, et al. Coupling nitrogen/oxygen self-doped biomass porous carbon cathode catalyst with CuFeO2/biochar particle catalyst for the heterogeneous visible-light driven photo-electro-fenton degradation of tetracycline, Appl. Catal , B: Environ. 2022;305:121024. doi: 10.1016/j.apcatb.2021.121024
- Yang K, Yue Q, Kong J, et al. Microbial diversity in combined UAF–UBAF system with novel sludge and coal cinder ceramic fillers for tetracycline wastewater treatment. J Chem Eng. 2016;285:319–330. doi: 10.1016/j.cej.2015.10.019
- Huang X, Zhu N, Mao F, et al. Enhanced heterogeneous photo-fenton catalytic degradation of tetracycline over yCeO2/Fh composites: performance, degradation pathways, Fe2+ regeneration and mechanism. J Chem Eng. 2020;392:123636. doi: 10.1016/j.cej.2019.123636
- Bao X, Wu Q, Shi W, et al. Polyamidoamine dendrimer grafted forward osmosis membrane with superior ammonia selectivity and robust antifouling capacity for domestic wastewater concentration. Water Res. 2019;153:1–10. doi: 10.1016/j.watres.2018.12.067
- Zhang Y, Cui W, An W, et al. Combination of photoelectrocatalysis and adsorption for removal of bisphenol a over TiO2-graphene hydrogel with 3D network structure, Appl. Catal , B: Environ. 2018;221:36–46. doi: 10.1016/j.apcatb.2017.08.076
- Zhang R, Zheng X, Zhang D, et al. Insight into the roles of endogenous minerals in the activation of persulfate by graphitized biochar for tetracycline removal. Sci Total Environ. 2021;768:144281. doi: 10.1016/j.scitotenv.2020.144281
- Chen M, He Y, Gu Z. Microwave irradiation activated persulfate and hydrogen peroxide for the treatment of mature landfill leachate effluent from a membrane bioreactor, Sep. Purif Technol. 2020;250:117111. doi: 10.1016/j.seppur.2020.117111
- Ribeiro AR, Nunes OC, Pereira MF, et al. Silva, an overview on the advanced oxidation processes applied for the treatment of water pollutants defined in the recently launched directive 2013/39/EU. Environ Int. 2015;75:33–51. doi: 10.1016/j.envint.2014.10.027
- Zhu L, Ai Z, Ho W, et al. Core–shell Fe–Fe2O3 nanostructures as effective persulfate activator for degradation of methyl orange, Sep. Purif Technol. 2013;108:159–165. doi: 10.1016/j.seppur.2013.02.016
- Mousset E, Loh WH, Lim WS, et al. Cost comparison of advanced oxidation processes for wastewater treatment using accumulated oxygen-equivalent criteria. Water Res. 2021;200:117234. doi: 10.1016/j.watres.2021.117234
- Song B, Zeng Z, Almatrafi E, et al. Pyrite-mediated advanced oxidation processes: applications, mechanisms, and enhancing strategies. Water Res. 2022;211:118048. doi: 10.1016/j.watres.2022.118048
- Tian K, Hu L, Li L, et al. Recent advances in persulfate-based advanced oxidation processes for organic wastewater treatment, Chin. Chem Lett. 2022;33(10):4461–4477. doi: 10.1016/j.cclet.2021.12.042
- Chu G, Zhao J, Liu Y, et al. The relative importance of different carbon structures in biochars to carbamazepine and bisphenol asorption. J Hazard Mater. 2019;373:106–114. doi: 10.1016/j.jhazmat.2019.03.078
- Ho SH, Chen YD, Li R, et al. N-doped graphitic biochars from C-phycocyanin extracted spirulina residue for catalytic persulfate activation toward nonradical disinfection and organic oxidation. Water Res. 2019;159:77–86. doi: 10.1016/j.watres.2019.05.008
- Li A, Wu Z, Wang T, et al. Kinetics and mechanisms of the degradation of PPCPs by zero-valent iron (Fe°) activated peroxydisulfate (PDS) system in groundwater. J Hazard Mater. 2018;357:207–216. doi: 10.1016/j.jhazmat.2018.06.008
- Qu S, Yuan Y, Yang X, et al. Carbon defects in biochar facilitated nitrogen doping: the significant role of pyridinic nitrogen in peroxymonosulfate activation and ciprofloxacin degradation. J Chem Eng. 2022;441:135864. doi: 10.1016/j.cej.2022.135864
- Cui P, Liu C, Su X, et al. Atomically dispersed manganese on biochar derived from a hyperaccumulator for photocatalysis in organic pollution remediation. Environ Sci Technol. 2022;56(12):8034–8042. doi: 10.1021/acs.est.2c00992
- Yuan Y, Zhang C, Zhao C, et al. One-step preparation of a novel graphitic biochar/Cu-0/Fe3O4 composite using CO2-ambiance pyrolysis to activate peroxydisulfate for dye. J Environ Sci. 2023;125:26–36. doi: 10.1016/j.jes.2021.10.030
- Wang Z, Shen R, Ji S, et al. Effects of biochar derived from sewage sludge and sewage sludge/cotton stalks on the immobilization and phytoavailability of pb, Cu, and zn in sandy loam soil. J Hazard Mater. 2021;419:126468. doi: 10.1016/j.jhazmat.2021.126468
- Xiao P, Yi X, Wu M, et al. Catalytic performance and periodate activation mechanism of anaerobic sewage sludge-derived biochar. J Hazard Mater. 2022;424:127692. doi: 10.1016/j.jhazmat.2021.127692
- Han L, Ro KS, Sun K, et al. New evidence for high sorption capacity of hydrochar for hydrophobic organic pollutants. Environ Sci Technol. 2016;50(24):13274–13282. doi: 10.1021/acs.est.6b02401
- Zhang J, Xin B, Shan C, et al. Roles of oxygen-containing functional groups of O-doped g-C3N4 in catalytic ozonation: quantitative relationship and first-principles investigation, Appl. Catal , B: Environ. 2021;292:120155. doi: 10.1016/j.apcatb.2021.120155
- Jing F, Liu Y, Chen J. Insights into effects of ageing processes on Cd-adsorbed biochar stability and subsequent sorption performance. Environ Pollut. 2021;291:118243. doi: 10.1016/j.envpol.2021.118243
- Baek J, Shin HS, Chung DC, et al. Studies on the correlation between nanostructure and pore development of polymeric precursor-based activated hard carbons: II. transmission electron microscopy and raman spectroscopy studies. J Ind Eng Chem. 2017;54:324–331. doi: 10.1016/j.jiec.2017.06.007
- Smith MW, Dallmeyer I, Johnson TJ, et al. Structural analysis of char by raman spectroscopy: improving band assignments through computational calculations from first principles. Carbon. 2016;100:678–692. doi: 10.1016/j.carbon.2016.01.031
- Li J, Liang N, Jin X, et al. The role of ash content on bisphenol asorption to biochars derived from different agricultural wastes. Chemosphere. 2017;171:66–73. doi: 10.1016/j.chemosphere.2016.12.041
- Li S, Barreto V, Li R, et al. Nitrogen retention of biochar derived from different feedstocks at variable pyrolysis temperatures. J Anal Appl Pyrolysis. 2018;133:136–146. doi: 10.1016/j.jaap.2018.04.010
- Wei X, Gao N, Li C, et al. Zero-valent iron (ZVI) activation of persulfate (PS) for oxidation of bentazon in water. J Chem Eng. 2016;285:660–670. doi: 10.1016/j.cej.2015.08.120
- Anipsitakis GP, Dionysiou DD. Degradation of organic contaminants in water with sulfate radicals generated by the conjunction of peroxymonosulfate with cobalt. Environ Sci Technol. 2003;37:4790–4797. doi: 10.1021/es0263792
- Lee J, von Gunten U, Kim JH. Persulfate-based advanced oxidation: critical assessment of opportunities and roadblocks. Environ Sci Technol. 2020;54:3064–3081. doi: 10.1021/acs.est.9b07082
- Wang S, Wang J. Nitrogen doping sludge-derived biochar to activate peroxymonosulfate for degradation of sulfamethoxazole: modulation of degradation mechanism by calcination temperature. J Hazard Mater. 2021;418:126309. doi: 10.1016/j.jhazmat.2021.126309
- Huang KZ, Zhang H. Direct electron-transfer-based peroxymonosulfate activation by iron-doped manganese oxide (δ-MnO2) and the development of galvanic oxidation processes (GOPs. Environ Sci Technol. 2019;53:12610–12620. doi: 10.1021/acs.est.9b03648
- Dou J, Cheng J, Lu Z, et al. Biochar co-doped with nitrogen and boron switching the free radical based peroxydisulfate activation into the electron-transfer dominated nonradical process, Appl. Catal , B: Environ. 2022;301:120832. doi: 10.1016/j.apcatb.2021.120832
- Wang H, Guo W, Liu B, et al. Sludge-derived biochar as efficient persulfate activators: sulfurization-induced electronic structure modulation and disparate nonradical mechanisms, Appl. Catal , B: Environ. 2020;279:119361. doi: 10.1016/j.apcatb.2020.119361
- Cheng X, Guo H, Zhang Y, et al. Non-photochemical production of singlet oxygen via activation of persulfate by carbon nanotubes. Water Res. 2017;113:80–88. doi: 10.1016/j.watres.2017.02.016
- Yin R, Guo W, Wang H, et al. Singlet oxygen-dominated peroxydisulfate activation by sludge-derived biochar for sulfamethoxazole degradation through a nonradical oxidation pathway: performance and mechanism. J Chem Eng. 2019;357:589–599. doi: 10.1016/j.cej.2018.09.184
- Xu Y, Lin H, Li Y, et al. The mechanism and efficiency of MnO2 activated persulfate process coupled with electrolysis. Sci Total Environ. 2017;609:644–654. doi: 10.1016/j.scitotenv.2017.07.151