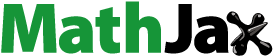
ABSTRACT
A novel strategy for reclamation and reuse of phosphate from aqueous solution was developed using Mg-enriched biochar prepared from anaerobically digested sludge (ADBC). The ADBC pyrolyzed at 600°C (ADBC-600) exhibited the maximum phosphate removal capacity of 23.2 mg/g, which was much higher than that of raw sludge biocha (10.1 mg/g). Adsorption kinetic and isotherm experiments showed that the adsorption of phosphate on ADBC-600 could be well described by the pseudo-second-order model and Langmuir model, indicating that the adsorption was mainly dominated by chemisorption process. The postsorption characterization results suggested that Mg-induced precipitation was likely the main P adsorption mechanism by ADBC. Moreover, the spent biochar was re-utilized as an efficient phosphate fertilizer for improving wheat seed germination and growth. Provided a sustainable and eco-friendly strategy for sludge treatment and phosphate recovery in wastewater treatment plants (WWTPs) and potential agronomic application.
1. Introduction
The activated sludge process is extensively used in municipal and industrial wastewater treatment plants (WWTPs) for over 100 years due to its high efficiency in removing organic contaminants [Citation1]. However, large amounts of waste activated sludge (WAS) are inevitably generated as a by-product of biological wastewater treatment and become a severe environmental problem for wastewater industry. Most common practices for WAS disposal (e.g. landfill and incineration) are not suitable due to land scarcity, severe secondary pollution and increasingly stringent environmental regulations. At this juncture, anaerobic digestion (AD) has been recommended as an efficient technology to treat WAS, by which the sludge reduction and bioenergy production are simultaneously realized [Citation2,Citation3]. The potential application of AD as a practicable and sustainable solution for sludge management is widely recognized.
In principle, raw sludge flocs can be converted into biogas (e.g. methane and hydrogen), digestion liquid and residue after anaerobic digestion process. Although various pretreatments including chemical, mechanical, thermal and enzymatic methods are employed to promote the solubilization and hydrolysis of WAS and/or accelerate bio-resource accumulation [Citation4,Citation5], most of AD reactors generate 30–85% of the WAS volume as digested residues [Citation6,Citation7]. Conventional disposal technologies of anaerobically digested sludge (ADS) such as compost and landfill may pose potential threats to environmental safety and human health due to its contamination with pathogens and heavy metals (HMs) [Citation8,Citation9]. Therefore, it is necessary and urgent to develop a novel strategy for the stabilization and resourcelization of ADS.
The pyrolytic conversion of biomass into biochar is attracting increasing attention for its potential applications including pollutants removal, soil amelioration and agronomic cultivation. Various organic agricultural by-products such as cereal straw [Citation10] and palm leaves [Citation11], as well as solid waste such as WAS [Citation12–14] have been widely employed to obtain biochar. Recently, as a relatively new concept, the utilization of digested agricultural residues such as bagasse and sugar beet tailings for biochar production is considered as an efficient and promising strategy to recycle the digested residues in an sustainable and environmentally benign way [Citation15,Citation16]. These studies inspire us that the pyrolysis of ADS may not only achieve more energy-efficient utilization from digested sludge residues, but also create powerful and engineered carbon-based material via biological activation [Citation17]. However, the research about feasibility of ADS-derived biochar and its potential application is still limited.
In this study, a new strategy of sludge management through the pyrolysis of ADS to produce biochar as a beneficial product was established. Both raw and digested sludge were employed for biochar production via pyrolysis and their physicochemical properties were comparatively characterized. The ADS-based biochar was used to examine its performance for phosphate removal from wastewater. Subsequently, a pot experiment was conducted to test the effects of spent biochar as phosphate fertilizer on seed germination and seedling growth. The specific objectives of this research were to: (1) investigate whether the ADS can be considered as a potentially promising feedstock for biochar production, (2) determine the sorption characteristics of phosphate to the biochar derived from ADS, and (3) evaluate the availability of phosphate adsorbed biochar as a fertilizer for plant growth.
2. Materials and methods
2.1. Sludge sources
The raw sludge used in this study was obtained from the secondary sedimentation tank of a municipal wastewater treatment plant in Nanjing, China. The collected WAS were concentrated by settling for 24 h and refrigerated at 4°C before use. ADS was harvested from a thermophilic anaerobic digester treating WAS for 2 weeks. To improve anaerobic digestion efficiency, the pH value was adjusted at 10.0 by adding the mixture of NaOH and Mg(OH)2 according to our previous study [Citation18]. The main characteristics of the raw sludge and ADS are shown in .
Table 1. Characteristics of waste activated sludge (WAS) and anaerobically digested sludge (ADS).
2.2. Biochar preparation
Both raw sludge and ADS were first oven-dried at 80°C for 3 days. Subsequently, a certain amount of dried sample was placed in a porcelain boat and pyrolyzed at a horizontal muffle furnace at the desired temperatures of 400, 500 and 600°C under N2 atmosphere, respectively. The pyrolysis was carried out at a heating rate of 10°C min−1 with a residence time of 2 h. After pyrolysis, the as-prepared biochar was passed through a sieve with a mesh of 2 mm, weighted and stored in a desiccator before characterization. The raw sewage sludge biochars (RSBC) obtained at different temperatures were named as RSBC-400, RSBC-500 and RSBC-600, respectively. The corresponding anaerobically digested sludge biochars (ADBC) were named as ADBC-400, ADBC-500 and ADBC-600, respectively.
2.3. Biochar characterization
The elemental C, H and N contents of the biochars were determined using an elemental analyzer (CHN-O-Rapid, Heraeus, Germany). The total contents of HMs in the samples were determined by microwave-assisted digestion method based on our previous study [Citation19]. Briefly, 0.100 g of sample was digested with the mixture of 6 mL HNO3, 2 mL HCl and 1 mL HF in a microwave digester (GH08Z-Z, Shanghai Metash Instruments, China), and then the concentration of major HMs in the digested liquid was measured by inductively coupled plasma-atomic emission spectrometry (ICP-AES) (Optima 7000DV, PerkinElmer, U.S.A.). The Brunauer-Emmett-Teller (BET) surface area was measured using N2 adsorption-desorption isotherm measurements with an automatic specific surface analyzer (ASAP2020, Micromeritics, U.S.A.). The surface functional groups of biochars were characterized using Fourier transform infrared (FTIR) spectroscopy (Nicolet iS50R, Thermo Fisher, U.S.A.). The pH of biochar was determined at a ratio of 1:10 (m/v) for biochar to Milli-Q water with a pH analyzer (Mettler FE20, Mettler Toledo, U.S.A.).
2.4. Phosphate adsorption experiments
The stock solution of phosphate was prepared by dissolving appropriate amount of KH2PO4 in Milli-Q water. The desired working concentrations in the experiments were prepared by further diluting the stock solution. The adsorption experiments of phosphate were conducted by mixing 0.1 g biochar samples (RSBC or ADBC) with 100 mL of phosphate solutions (50 mg P/L) in 250 mL conical flasks. The flasks were placed in an air-bath shaker at 150 rpm with constant temperature of 25 ± 2°C. After 24 h sharking, the mixtures were centrifuged at 5000 rpm for 5 min and then filtered through 0.22 μm PFS membrane filter prior to analysis. The post-sorption solid samples were dried and ground to pass through a 2-mm sieve for subsequent plant growth experiments. The concentration of residual phosphate was determined by the molybdenum antimony ascorbic acid colorimetry method [Citation20] using UV spectrophotometer (LAMBDA 25, PerkinElmer, U.S.A.). The phosphate adsorption capacity of biochar qe (mg/g) was calculated using the following equation:
where C0 (mg/L) and Ce (mg/L) are the initial and equilibrium concentration of phosphate in aqueous solution, respectively, and m represents the biochar dose (g).
Specifically, the adsorption kinetics of phosphate was conducted as aforementioned procedures in the contact time from 0 to 180 min. The adsorption isotherm experiments were carried out in the various initial phosphate concentrations from 5 to 100 mg/L with constant temperature of 25 ± 2°C, and the Langmuir and Freundlich adsorption isotherm models were used to fit the experimental data of ADBC-600 [Citation21].
2.5. Wheat seed germination rate and seedling growth bioassay
The potted soils were collected from the top 0–20 cm for a garden field in Nanjing, China (32°01’51“N, 118°51’17“E). The main characteristics of soil were presented in Table S1. The seedling growth bioassay was conducted in a glasshouse condition using the soils amended with or without P-loaded biochar to determine the feasibility of P adsorbed biochar as a slow-release fertilizer. Twenty wheat (Triticum aestivum L.) seeds were sown in 500 mg soil in a plastic container (diameter 12 cm, depth 7.5 cm). All pots were kept moist by irrigating approximately every 2 days and incubated at room temperature. The seed germination and shoot growth were determined after 10 days of growth.
2.6. Statistical analysis
All experimental data were determined through triplicate measurements, and the results were expressed as mean ± standard deviation. One-way analysis of variance (ANOVA) was conducted by SPSS statistics 23 to evaluate the significance of results, and p value < 0.05 was considered statistically significant.
3. Results and discussion
3.1. Physicochemical characteristics of the RSBC and ADBC
3.1.1. The yield and pH value
The main properties of both RSBC and ADBC are presented in . The yield of all the biochars decreased with the increasing pyrolysis temperature. For instance, the yield rates of RSBC and ADBC ranged from 53.47% to 43.38% and 68.32% to 59.43%, respectively, when the pyrolysis temperature increased from 400 to 600°C. A similar observation was reported for biochar derived from both sludge [Citation22] and other feedstock [Citation23]. The results were attributed to the gradual decomposition of volatile substances with the increased temperature. Moreover, the biochar yield from digested sludge was much higher compared with that of raw sludge. The difference could be due to the consumption of carbon content during the digestion process, and the other reason was likely the accumulation of minerals caused by dosing of Mg(OH)2 and NaOH.
Table 2. Physicochemical properties of the biochars under different pyrolysis temperature.
In addition, it was not surprising that both RSBC and ADBC were alkaline and the alkalinity of biochar became stronger at higher temperature. Moreover, the pH of ADBC was much higher than that of raw sludge biochar. In detail, the pH value of RSBC increased from 8.68 to 9.24 with increasing temperature from 400 to 600°C. By contrast, the pH was 9.25, 9.38 and 9.58 for ADBC-400, ADBC-500 and ADBC-600, respectively. The higher pH values of ADBC samples could be attributed to the addition of alkali metal salts, i.e. Mg(OH)2 as its concentration were relatively high with ADBC. It should be noted that high alkalinity of biochar could influence the removal capacity of some pollutants [Citation24]. Additionally, increased pH is beneficial for mitigating soil acidy when biochar is applied to soil, thereby changing the availability of nutrients and some elements uptake by root [Citation25].
3.1.2. Elemental composition
As presented in , the total C contents of ADBCs were in the range of 10.12–13.58%, which were slightly lower compared with the RSBCs produced at the same condition. The results could be attributed to the conversion of organic substrates to biomass energy during anaerobic digestion. The molar H/C and N/C ratios are the main parameters for carbonization degree, which is generally used to characterize the organic compound aromaticity in biochar. The results showed that the molar ratios of ADBC were lower compared with the corresponding RSBC, indicating strong carbonization and higher aromaticity that resist decomposition.
3.1.3. Hms contents and leaching characterizations
Previous researches have shown that the presence of HMs in sludge-derived biochar is still of great concern for practical application in soil remediation and contaminant removal [Citation26]. Therefore, the total concentrations of HMs were measured to evaluate the environmental risk of ADBC and RSBC (). For all the biochars, the total concentrations of HMs decreased as follows: Zn>Cr>Cu>Ni>As>Cd. Comparing with the threshold values, all the HMs contents were lower than the content limit required in CJ/T 362–2011, indicating the low toxicity to environment if the ADBC was used in forestland. In the current study, the sewage sludge samples were collected from strictly domestic wastewater without the influx of industrial wastewater, thereby most of trace elements are likely below the concentrations of concern. However, the concentration of Zn from the biochars slightly exceeded the national standard for sludge used in farmland, which was likely due to the extensive use of galvanized pipes in sewage treatment system in China [Citation27]. Moreover, showed that the total contents of HMs in RSBC and ADBC increased when the pyrolysis temperature increased from 400°C to 600°C, which likely attributed to the lower loss in weight of HMs than the loss in weight of organic matter during pyrolysis.
Table 3. Total concentrations of HMs in biochars and their limits in the disposal standards of China.
Furthermore, the leaching characterization of HMs extracted from RSBC-600 and ADBC-600 was also investigated using the TCLP method recommended by USEPA, and the results are shown in . The concentrations of HMs leached from RSBC and ADBC did not exceed the permissible limits, indicating that these HMs in the biochars wouldn’t create any environmental risk to the applied groundwater and soil recipients. Moreover, the hazardous metals leaching in ADBC was much lower compared to those in the RSBC (except for As), which clearly suggested that the immobilization of HMs was improved by AD process. Although it has been reported that the HMs in sludge enriched during anaerobic digestion, their leachability largely depend on the chemical forms and binding state [Citation8]. The extremely lower leaching toxicity of ADBC could be attributed to the presence of alkaline matter such as Ca and Mg-compounds, which was beneficial for immobilizing HMs into biochar matrix during the carbonization process. These observations were consistent with the reports of Ho et al. [Citation28].
3.2. Phosphate removal performance of biochars
The removal performance synthetic wastewater of ADBC and RSBC pyrolyzed at temperature range of 400–600°C is shown in . ADBC exhibited significantly better P removal capacity than RSBC at same pyrolysis temperature, indicating that digested sludge could be considered as more effective feedstock for phosphate sorption than non-digested sludge. The adsorption capacity increased with the raised pyrolysis temperature, which could be attributed to greater carbonization at higher temperatures [Citation29]. Notably, the maximum phosphate adsorption amount of ADBC-600 was 23.2 mg/g, which was 1.3 times higher than that of RSBC-600. The results suggested that ADBC could be used as an effective adsorbent for P removal without any further modification. Therefore, ADBC-600 was selected for further investigation to reveal the comprehensive phosphate removal characteristics and mechanism.
Figure 2. Phosphate adsorption capacities of RSBC and ADBC under different pyrolysis temperatures. (different lowercase letters above bars indicates significant difference at p < 0.05 among various data groups).

The adsorption kinetics models are usually conducted to predict the adsorption efficiency and also provide valuable data to interpret the mechanism of phosphate adsorption [Citation30]. In the present work, two commonly used pseudo-first-order and pseudo-second-order models were employed to fit the experimental data and express as:
Pseudo-first-order model:
Pseudo-second-order model:
Where k1 (min−1) and k2 (g/mg min) are the rate constants for the pseudo-first-order and pseudo-second-order models, respectively. Here, qt and qe (mg/g) are the amount of phosphate adsorbed at time t and at equilibrium, respectively.
The fitting curves describing the experimental results are shown in , and the determined kinetic parameters are summarized in . The resutls indicated that the pseudo-second-order model was fitting the experimental data better with the R2 value of 0.996. Moreover, the theoretical phosphate adsorption capacity from pesudo-second-order model was very close to observed vaule in the experimental data (21.46 versus 21.23 mg/g), indicating that the chemisorption process might play the dominant role on the phosphate adsroption of ADBC-600.
Table 4. Phosphate adsorption kinetics and isotherm fitting parameters of ADBC-600.
Generally, adsorption isotherms are used to define the equilibrium adsorption capacity of absorbent and the distribution of adsorbate at solid/liquid interfaces [Citation24,Citation31]. In this study, Langmuir and Freundlich models were applied to fit the isothermal curves of P adsorption by ADBC-600, and the equations are presented in EquationEquations 4(4)
(4) –Equation6
(6)
(6) .
Langmuir model:
Freundlich model:
Where KL (L/mg) and KF is the Langmuir constant related to interaction energies and the Freundlich capacity coefficient respectively, Ce (mg·g−1) is the equilibrium concentration of phosphate, Qm (mg·g−1) is the maximum adsorption capacity, RL is the separation factor, indicating the favorability and affinity of adsorption process.
As shown in , the Langmuir model fit the experimental data better for phosphate adsorption with the correlation coefficient of 0.9601 compared to the Freundlich model (R2 = 0.8202), which suggested that the P adsorption on ADBC-600 was dominated by monolayer homogeneous adsorption process without interactions between the sorption species. Moreover, according to the separation factor RL value of 0.109 from Langmuir model, the adsorption of P on ADBC-600 was favorable. In addition, the maximum P adsorption capacity of ADBC-600 was compared to other chemically modified biochars used for phosphate removal (). It can be clearly noted that the adsorption capacity of ADBC-600 was superior or comparable to that of many other chemically modified biochars. Also, the simple fact of utilizing waste resource in a favorable manner suggests that ADBC could be considered a competitive alternative to meet with the requirement of both sludge management and energy recovery.
Table 5. Comparison of phosphate adsorption capacities of ADBC with other chemically modified biochars.
3.3. Adsorption mechanisms
Phosphate adsorption mechanisms by biochar have been extensively studied in previous literatures, and the major mechanisms underlying P adsorption can be distinguished into intraparticle diffusion, electrostatic interaction, ligand exchange, surface precipitation [Citation38,Citation39]. In this study, ADBC-600 and the corresponding raw biochar (RSBC-600) were comparatively characterized with the aim of understanding the mechanisms involved in P adsorption.
Many studies have demonstrated that the porous structure play an important role in physical adsorption. As provided in Table S2, the BET specific area of RSBC-600 and ADBC-600 were 78 and 68 m2/g, respectively, which were much less than that of modified biochars using other feedstocks [Citation40,Citation41]. The results were mainly attributed to the properties of biomass source and the absence of activation process. Although RSBC-600 has a larger surface area, its adsorption activity to phosphate was much less than ADBC-600. The FTIR spectra of ADBC and RSBC were also examined and the results are presented in Fig. S1. Although the peaks including 3729 cm−1 for the −OH stretching vibration, 1582 cm−1 for the C−O, 1405 cm−1 for C−H stretching vibrations in aromatic bonds and 600–800 cm−1 for M – O or O – M–O (M= Mg, Al and Fe) vibration bands appeared in all the groups [Citation42], no significant difference can be observed between ADBC and RSBC, which indicated that the phosphate removal capacity of ADBC should not be greatly attributed to the complexation interaction. Moreover, the zeta potential of ADBC-600 was −17.18 mV at pH 7, suggesting that the phosphate ions (i.e. H2PO4−, HPO42- and PO43-) with negative charge cannot easily be adsorbed in the surface of ADBC-600 due to the electrostatic repulsion [Citation43,Citation44]. Thus, the results indicated that the physical adsorption and electrostatic interaction were not responsible for the adsorption process with ADBC-600, which is consistent with the results of kinetics study that the whole phosphate adsorption process was controlled by multiple mechanisms.
Chemical precipitation was considered as the predominant mechanism for biochars containing metal oxides or hydroxides [Citation39]. shows the leaching contents of Mg2+ and Ca2+ from biochars during the phosphate adsorption process. It is noted that the amounts of Ca2+ in RSBC-600 and ADBC-600 were constant as the initial phosphate concentration increased, while the equilibrium Mg2+ concentration was sharply declined from 32.5 to 14.8 mg/L for ADBC-600. These results suggested that the Mg2+-induced chemical precipitation played an important role in phosphate adsorption by ADBC-600. Furthermore, XPS results of ADBC-600 before and after P adsorption were presented in . The main P 2p spectra could be deconvoluted into three peaks at 133.9 and 134.9 and 136 eV binding energies, which corresponded to the PO43-, HPO42- and H3PO4, respectively [Citation45]. After P adsorption, the integral area of P 2p peaks was distinctly increased from 306 to 1288 (CPS. eV), indicating that a certain amount of P was adsorbed on the surface of ADBC-600. In , the Mg binding energy could be deconvoluted into three magnesium components, i.e. MgO (1304 eV), MgHPO4 (1305 eV) and Mg3(PO4)2 (1306.7 eV) [Citation45]. A significant decline in the content of MgO was observed from 66.64% to 30.32%, while the ratio of magnesium-containing phosphate increased notably after adsorption. This evidence confirmed that the MgO on the surface of ADBC readily reacted with phosphate and Mg3(PO4)2 and MgHPO4 formed. In summary, the phosphate adsorption on the ADBC was mainly attributed to the precipitation of Mg-P precipitates.
3.4. The potential land application of P-laden ADBC as fertilizer
Previous studies have proposed that the use of P-laden absorbent as a soil additive could be more practical rather than regenerating it. Thus, the recycling feasibility of P-laden ADBC-600 as an inexpensive phosphate fertilizer was investigated in pot experiment for wheat germination and seedling growth (). The seed germination rate increased from 48% (control group) to 60–68% with the P-laden biochar dosage increased. After 10 days growth, the average shoot heights were also significantly increased with the amendment of ≥ 1.0% P-laden ADBC compared to the control treatment (p < 0.05). As expected, P-laden ADBC effectively improved both the seed germination and radicle growth, which was attributed to more available P under spent biochar application. These results are consistent with the findings of Fang et al. [Citation46] that higher P mobility in Mg-impregnated biochar improved P uptake by ryegrass.
Furthermore, the temporal changes of nutrients availability (including P, K, Ca and Mg) in the soil samples under different treatments are shown in Fig. S1. Compared with the unamended soil, the application of 0.5%, 1% and 2% P-laden biochar increased available P contents significantly (p < 0.05) by 61.8, 117% and 146% after 26 days of incubation, respectively, indicating that a large fraction of total P in P-laden biochar was readily extractable. This result was similar to the work of Yao et al. [Citation47], in which outer-sphere complex can be formed between protonated MgOH+ and phosphate and subsequently deposit onto the surface of biochar through weak chemical bonds. The concentrations of available K and Mg in soils also significantly increased as the biochar application dosages increased. However, P-laden ADBC amendment had non-significant effect on the Ca availability, although a slight increase could be observed compared to the control (p > 0.05). Thus, P-laden ADBC can not only be considered as phosphate fertilizer, but also act as a direct source of essential macronutrients for plant growth.
3.5. Implication to in-situ sludge management and future consideration
Our previous study has shown that combined NaOH and Mg(OH)2 pretreatment is a promising and economically attractive strategy for the simultaneous enhancement of VFAs production and sludge dewaterability [Citation18]. However, 30–85% of digested sludge will remain as residue and cannot be directly used on agricultural land due to contamination with high concentrations of hazardous substances (HMs, pathogens and organic pollutants). This study proposed and demonstrated that the magnesium-rich ADS can be converted into functional biochar with high phosphate removal efficiency from aqueous solution. shows a ‘closed-loop’ concept with the proposed multi-stage utilization strategy of WAS in WWTPs. Under this concept, the combined NaOH and Mg(OH)2 treatment is used as an efficient and low-cost technology to enhance WAS digestion and VFAs production. Thereafter, solid-liquid separation is conducted, by which anaerobic digestate can be divided as digestion liquor and residue sludge. Following separation unit, the Mg-modified biochar is fabricated by one-pot pyrolysis of the magnesium-rich ADS, which can be in-situ used for phosphorus recovery from the sludge supernatant. Moreover, the ‘purified’ digestion liquor is sent to bioreactor as supplement wastewater carbon source for biological nutrients removal (BNR) enhancement, and the exhausted phosphate-rich biochar can be readily used as fertilizer for plant growth in WWTPs. In summary, this study provides a promising strategy, i.e. combining anaerobic digestion and pyrolysis technology to reuse the digested sludge for nutrients recovery and agronomic application.
It should be highly noted that different pretreatment can be regarded as in-situ modification method to change the properties of digested sludge, which is expected to applied to expand the application filed of biochars. For example, persulfate/zero-valent iron treated ADS was successfully used to produce magnetic biochar catalyst for wastewater purification [Citation48]. Also, the ability of digested sludge-derived biochar in enhancing AD methanogenesis was demonstrated by previous study [Citation49]. Further study is therefore needed to evaluate the characterization of engineered ADS-derived biochars based on different pretreatment technologies and their potential application. Moreover, a comprehensive analysis of technical optimization and economic feasibility in full-scale trials is also required in the future.
4. Conclusions
In this work, the feasibility of using anaerobically digested sludge biochar (ADBC) to remove phosphate from aqueous solution was investigated. Adsorption experiments showed that ADBC-600 exhibited the maximum phosphate adsorption capacity of 23.2 mg/g, which was 1.3 times higher than that of the original biochar. The analysis of zeta potential, FTIR, BET and XPS demonstrated that chemical precipitation with Mg-minerals was likely the main mechanism of phosphate sorption by ADBC. Moreover, the spent biochar could be regarded as a P-laden fertilizer to improve soil fertility and plant growth based on the results of seedling growth bioassay. These findings provided a clear closed-loop approach for sludge management to achieve resources recovery and zero sludge discharge.
Supplemental Material
Download MS Word (375.5 KB)Acknowledgments
The authors thank the supporters of this project and the referees for their constructive comments.
Disclosure statement
No potential conflict of interest was reported by the author(s).
Supplementary material
Supplemental data for this article can be accessed online at https://doi.org/10.1080/26395940.2023.2287237
Additional information
Funding
References
- Wang X, Hou H, Liu P, et al. Acceleration of nitrogen removal performance in a biofilm reactor augmented with Pseudomonas sp. using polycaprolactone as carbon source for treating low carbon to nitrogen wastewater. Biores Technol. 2023;386:129507. doi: 10.1016/j.biortech.2023.129507
- Chai Y, Huang C, Sui M, et al. Fe-loaded alginate hydrogel beads activating peroxymonosulfate for enhancing anaerobic fermentation of waste activated sludge: performance and potential mechanism. J Environ Manage. 2023;341:118079. doi: 10.1016/j.jenvman.2023.118079
- Tulun Ş, Bilgin M. Enhancement of anaerobic digestion of waste activated sludge by chemical pretreatment. Fuel. 2019;254:115671. doi: 10.1016/j.fuel.2019.115671
- Ding HH, Chang S, Liu Y. Biological hydrolysis pretreatment on secondary sludge: enhancement of anaerobic digestion and mechanism study. Bioresour Technol. 2017;244:989–597. doi: 10.1016/j.biortech.2017.08.064
- Huang C, Wang W, Sun X, et al. A novel acetogenic bacteria isolated from waste activated sludge and its potential application for enhancing anaerobic digestion performance. J Environ Manage. 2020;255:109842. doi: 10.1016/j.jenvman.2019.109842
- Carrère H, Dumas C, Battimelli A, et al. Pretreatment methods to improve sludge anaerobic degradability: a review. J Hazard Mater. 2010;183(1):1–15. doi: 10.1016/j.jhazmat.2010.06.129
- Selvaraj PS, Periasamy K, Suganya K, et al. Novel resources recovery from anaerobic digestates: Current trends and future perspectives. Critical Rev Environ Sci Technol. 2022;52(11):1915–1999. doi: 10.1080/10643389.2020.1864957
- Dong B, Liu X, Dai L, et al. Changes of heavy metal speciation during high-solid anaerobic digestion of sewage sludge. Bioresour Technol. 2013;131:152–158. doi: 10.1016/j.biortech.2012.12.112
- Wang Z, Liu T, Duan H, et al. Post-treatment options for anaerobically digested sludge: Current status and future prospect. Water Res. 2021;205:117665. doi: 10.1016/j.watres.2021.117665
- Cui L, Noerpel MR, Scheckel KG, et al. Wheat straw biochar reduces environmental cadmium bioavailability. Environ Int. 2019;126:69–75. doi: 10.1016/j.envint.2019.02.022
- Almanassra IW, Chatla A, Zakaria Y, et al. Palm leaves based biochar: advanced material characterization and heavy metal adsorption study. Biomass Convers Biorefin. 2022. doi:10.1007/s13399-022-03590-y
- Chen T, Zhang Y, Wang H, et al. Influence of pyrolysis temperature on characteristics and heavy metal adsorptive performance of biochar derived from municipal sewage sludge. Biores Technol. 2014;164:47–54. doi: 10.1016/j.biortech.2014.04.048
- Li J, Li B, Huang H, et al. Removal of phosphate from aqueous solution by dolomite-modified biochar derived from urban dewatered sewage sludge. Sci Total Environ. 2019;687:460–469. doi: 10.1016/j.scitotenv.2019.05.400
- Song XD, Xue XY, Chen DZ, et al. Application of biochar from sewage sludge to plant cultivation: influence of pyrolysis temperature and biochar-to-soil ratio on yield and heavy metal accumulation. Chemosphere. 2014;109:213–220. doi: 10.1016/j.chemosphere.2014.01.070
- Inyang M, Gao B, Pullammanappallil P, et al. Biochar from anaerobically digested sugarcane bagasse. Biores Technol. 2010;101(22):8868–8872. doi: 10.1016/j.biortech.2010.06.088
- Yao Y, Gao B, Inyang M, et al. Biochar derived from anaerobically digested sugar beet tailings: characterization and phosphate removal potential. Biores Technol. 2011;102(10):6273–6278. doi: 10.1016/j.biortech.2011.03.006
- Ni BJ, Huang QS, Wang C, et al. Competitive adsorption of heavy metals in aqueous solution onto biochar derived from anaerobically digested sludge. Chemosphere. 2019;219:351–357. doi: 10.1016/j.chemosphere.2018.12.053
- Huang C, Lai J, Sun X, et al. Enhancing anaerobic digestion of waste activated sludge by the combined use of NaOH and Mg (OH) 2: performance evaluation and mechanism study. Biores Technol. 2016;220:601–608. doi: 10.1016/j.biortech.2016.08.043
- Huang C, Wang L, Fan L, et al. Co-pyrolysis of fenton sludge and pomelo peel for heavy metal stabilization: speciation mechanism and risk evaluation. Water. 2023;15(21):3733. doi: 10.3390/w15213733
- Yu J, Li X, Wu M, et al. Synergistic role of inherent calcium and iron minerals in paper mill sludge biochar for phosphate adsorption. Sci Total Environ. 2022;834:155193. doi: 10.1016/j.scitotenv.2022.155193
- Khalil AKA, Dweiri F, Almanassra IW, et al. Mg-al layered Double hydroxide doped activated carbon composites for phosphate removal from synthetic water: adsorption and thermodynamics studies. Sustainability. 2022;14(12):6991. doi: 10.3390/su14126991
- Hossain MK, Strezov V, Chan KY, et al. Influence of pyrolysis temperature on production and nutrient properties of wastewater sludge biochar. J Environ Manage. 2011;92(1):223–228. doi: 10.1016/j.jenvman.2010.09.008
- Wang Z, Guo H, Shen F, et al. Biochar produced from oak sawdust by Lanthanum (La)-involved pyrolysis for adsorption of ammonium (NH4+), nitrate (NO3−), and phosphate (PO43−). Chemosphere. 2015;119(Supplement C):646–653. doi: 10.1016/j.chemosphere.2014.07.084
- Tang Y, Alam MS, Konhauser KO, et al. Influence of pyrolysis temperature on production of digested sludge biochar and its application for ammonium removal from municipal wastewater. J Clean Prod. 2019;209:927–936. doi: 10.1016/j.jclepro.2018.10.268
- Bolan N, Sarmah AK, Bordoloi S, et al. Soil acidification and the liming potential of biochar. Environ Pollut. 2023;317:120632. doi: 10.1016/j.envpol.2022.120632
- Zhang S, Gu W, Geng Z, et al. Immobilization of heavy metals in biochar by co-pyrolysis of sludge and CaSiO3. J Environ Manage. 2023;326:116635. doi: 10.1016/j.jenvman.2022.116635
- Wang X, Wei-Chung Chang V, Li Z, et al. Co-pyrolysis of sewage sludge and food waste digestate to synergistically improve biochar characteristics and heavy metals immobilization. Waste Manage. 2022;141:231–239. doi: 10.1016/j.wasman.2022.02.001
- Ho S-H, Chen Y-D, Yang Z-K, et al. High-efficiency removal of lead from wastewater by biochar derived from anaerobic digestion sludge. Bioresour Technol. 2017;246:142–149. doi: 10.1016/j.biortech.2017.08.025
- Huang P, Ge C, Feng D, et al. Effects of metal ions and pH on ofloxacin sorption to cassava residue-derived biochar. Sci Total Environ. 2018;616-617:1384–1391. doi: 10.1016/j.scitotenv.2017.10.177
- Shi W, Fu Y, Jiang W, et al. Enhanced phosphate removal by zeolite loaded with Mg–al–La ternary (hydr)oxides from aqueous solutions: performance and mechanism. Chem Eng J. 2019;357:33–44. doi: 10.1016/j.cej.2018.08.003
- Chen Y-D, Ho S-H, Wang D, et al. Lead removal by a magnetic biochar derived from persulfate-ZVI treated sludge together with one-pot pyrolysis. Bioresour Technol. 2018;247:463–470. doi: 10.1016/j.biortech.2017.09.125
- Li JH, Lv GH, Bai WD, et al. Modification and use of biochar from wheat straw (triticum aestivum L.) for nitrate and phosphate removal from water. Desalin Water Treat. 2016a;57(10):4681–4693.
- Wang L, Wang J, He C, et al. Development of rare earth element doped magnetic biochars with enhanced phosphate adsorption performance. Colloids Surf A Physicochem Eng Asp. 2019;561:236–243. doi: 10.1016/j.colsurfa.2018.10.082
- Li R, Wang JJ, Zhou B, et al. Recovery of phosphate from aqueous solution by magnesium oxide decorated magnetic biochar and its potential as phosphate-based fertilizer substitute. Biores Technol. 2016b;215:209–214. doi: 10.1016/j.biortech.2016.02.125
- Peng Y, Luo Y, Li Y, et al. Effect of corn pre-puffing on the efficiency of MgO-engineered biochar for phosphorus recovery from livestock wastewater: mechanistic investigations and cost benefit analyses. Biochar. 2023;5(1):26. doi: 10.1007/s42773-023-00212-2
- Luo H, Wan Y, Cai Y, et al. Enhanced phosphate adsorption by mg-stirred leaf biochar in a complex water matrix via active MgO facet exposure. ACS EST Eng. 2022;2(12):2254–2265. doi: 10.1021/acsestengg.2c00212
- Zheng Q, Yang L, Song D, et al. High adsorption capacity of Mg–Al-modified biochar for phosphate and its potential for phosphate interception in soil. Chemosphere. 2020;259:127469. doi: 10.1016/j.chemosphere.2020.127469
- Chatla A, Almanassra IW, Jaber L, et al. Influence of calcination atmosphere on Fe doped activated carbon for the application of lead removal from water. Colloids Surf A Physicochem Eng Asp. 2022;652:129928. doi: 10.1016/j.colsurfa.2022.129928
- Vikrant K, Kim KH, Ok YS, et al. Engineered/Designer biochar for the removal of phosphate in water and wastewater. Science Of The Total Environment. 2018;616-617:1242–1260. doi: 10.1016/j.scitotenv.2017.10.193
- Takaya CA, Fletcher LA, Singh S, et al. Recovery of phosphate with chemically modified biochars. J Environ Chem Eng. 2016;4(1):1156–1165. doi: 10.1016/j.jece.2016.01.011
- Wan S, Wang S, Li Y, et al. Functionalizing biochar with Mg–al and Mg–Fe layered double hydroxides for removal of phosphate from aqueous solutions. J Ind Eng Chem. 2017;47:246–253. doi: 10.1016/j.jiec.2016.11.039
- Jiang D, Chu B, Amano Y, et al. Removal and recovery of phosphate from water by Mg-laden biochar: batch and column studies. Colloids Surf A Physicochem Eng Asp. 2018;558:429–437. doi: 10.1016/j.colsurfa.2018.09.016
- Wang Z, Shen D, Shen F, et al. Phosphate adsorption on lanthanum loaded biochar. Chemosphere. 2016;150:1–7. doi: 10.1016/j.chemosphere.2016.02.004
- Yao Y, Gao B, Zhang M, et al. Effect of biochar amendment on sorption and leaching of nitrate, ammonium, and phosphate in a sandy soil. Chemosphere. 2012;89(11):1467–1471. doi: 10.1016/j.chemosphere.2012.06.002
- Zhu D, Chen Y, Yang H, et al. Synthesis and characterization of magnesium oxide nanoparticle-containing biochar composites for efficient phosphorus removal from aqueous solution. Chemosphere. 2020;247:125847. doi: 10.1016/j.chemosphere.2020.125847
- Fang L, Yan F, Chen J, et al. Novel recovered compound phosphate fertilizer produced from sewage sludge and its incinerated ash. ACS Sustain Chem Eng. 2020;8(17):6611–6621. doi: 10.1021/acssuschemeng.9b06861
- Yao Y, Gao B, Chen J, et al. Engineered biochar reclaiming phosphate from aqueous solutions: mechanisms and potential application as a slow-release fertilizer. Environ Sci Technol. 2013;47(15):8700–8708. doi: 10.1021/es4012977
- Chen Y-D, Bai S, Li R, et al. Magnetic biochar catalysts from anaerobic digested sludge: production, application and environment impact. Environ Int. 2019;126:302–308. doi: 10.1016/j.envint.2019.02.032
- Duan S, He J, Xin X, et al. Characteristics of digested sludge-derived biochar for promoting methane production during anaerobic digestion of waste activated sludge. Bioresour Technol. 2023;384:129245. doi: 10.1016/j.biortech.2023.129245