ABSTRACT
Water scarcity is a critical global challenge exacerbated by population growth and industrialization. Contamination of water sources by diverse pollutants poses significant threats to public health. Consequently, there is an urgent need for the development of advanced technologies capable of purifying and detoxifying water. Beyond safeguarding health, these water purification technologies play a pivotal role in enabling water recycling to meet demands during periods of limited availability.This review provides a comprehensive overview of various methods for water remediation, encompassing both chemical and biotechnological processes while highlighting distinct water treatment approaches. The key points include the integration of nanotechnology in water remediation as an innovative approach to enhance water treatment efficiency. The article explains various techniques for synthesizing environmentally friendly nanomaterials and discusses their applications in environmental remediation in detail. The use of nanomaterials in water remediation has shown promising results, as they can effectively remove contaminants from water without causing harm to the environment. The review also emphasizes the need for further research to explore the potential of nanotechnology in addressing the global water scarcity issue. Nanomaterials have shown promise in effectively removing contaminants without harming the environment. However, challenges such as addressing long-term toxicity effects on ecosystems, scaling up nanoparticle synthesis, and conducting more pilot studies persist. Future directions should focus on optimizing nanotechnology-based water remediation processes through interdisciplinary research and pilot-scale implementation to ensure efficacy, safety, and scalability. By elucidating key aspects of water remediation, with a spotlight on nanotechnology, the review aims to contribute to a deeper understanding of methods that can mitigate the challenges of water scarcity and contamination.
1. Introduction
The discharge of wastewater from industries, characterized by a continuous flow and containing complex organic compounds, colors, heavy metals, phenolic compounds, refractory chlorine, and elevated levels of toxic non-organic pollutants, poses a significant environmental concern. This effluent is regularly released into the atmosphere, presenting serious health risks to both ecosystems and human populations [Citation1,Citation2]. Due to the enormous manufacturing release of industrial wastewater (IWW) without treatment, water bodies are seriously compromising the sustainability and safety of the environment by polluting natural resources [Citation3]. Adsorption, precipitation, ion exchange, coagulation, biosorption, electro-dialysis (ED), and several other traditional treatment techniques have been used for treating and managing waste produced by industrial operations since antiquity [Citation4–6]. These methods are more expensive and need more energy; hence they are less suited and efficient. To boost efficiency over traditional methods, new and advanced techniques are being developed. These technologies bring excellent analytical functionality of wastewater characterization. The low cost, environmental protection ability, and more effective disposal of hazardous waste, numerous renewable initiatives, including bioremediation and phytoextraction, are increasingly widely used for treating Industrial Waste Water (IWW) [Citation7–9].
The discriminating surface reactivity of nanoparticles and nanomaterials has garnered substantial interest among researchers [Citation10]. Their remarkable versatility for environmental cleanup [Citation11] and effectiveness in decomposing aqueous organic solutes [Citation12,Citation13] make them particularly promising for various applications. The unique optical, electrical, antimicrobial, and catalytic characteristics of nanoparticles and nanomaterials have led to their widespread utilization across diverse fields, including engineering, biology, bio-remediation, clinical materials, and cosmetics [Citation14–18]. Their adaptability and multifunctionality position them as valuable tools in addressing complex challenges and advancing innovative solutions in these domains. The use of nanoparticles and nanomaterials not only underscores their efficacy in specific applications but also highlights their potential to revolutionize various industries. Researchers and practitioners are increasingly recognizing and harnessing the distinct properties of these materials to create novel and improved technologies that contribute to advancements in science and technology. As investigations into their capabilities continue, the integration of nanoparticles and nanomaterials into different fields is expected to expand, further enhancing their role in shaping the future of interdisciplinary research and application development.
Diverse protocols, like chemical reactions [Citation19], photo degradation [Citation20], and thermal evaporation [Citation21,Citation22], have been used by the nanoparticles for water remediation. However, most of these methods use risky chemicals, call for specialized equipment, require a lot of energy, are typically high cost, and demand time-consuming cleaning procedures. Because of this, it is essential to develop environmentally benign and enduring processes for the preparation of nanoparticles. Natural principles have recently been used to get around these technical challenges, and materials science researchers are working to comprehend, emulate, and take advantage of natural processes in order to do this [Citation23]. Natural physicochemical processes give rise to naturally occurring nanoparticles. Incidental nanomaterials, commonly referred to as anthropogenic or trash particles, are produced by industrial processes [Citation24]. In order to create materials with specified properties, engineered nanoparticles are created in the lab or industry. Engineered nanomaterials that replicate the characteristics of biological materials or living things are called bioinspired nanomaterials [Citation25]. Researchers interest in engineered and bioinspired nanoparticles is growing, but their mass share compared to natural nanomaterials is still quite limited [Citation26–29]. Due to the necessity for bulk-scale manufacturing and risk evaluation procedures, few manufactured and bioinspired nanoparticles have been accepted and deployed in the industry despite their unique features.
This review comprehensively examines bioinspired nanomaterials for water remediation, providing insights into their sources, preparation methods, and diverse applications. By offering a succinct overview of industries discharging wastewater and the typical contaminants found in industrial wastewater (IWW), the review establishes a context for understanding the complexities of water pollution. It further consolidates information on traditional and operational treatment systems while emphasizing the need for contextually appropriate approaches to effectively monitor and remediate IWW before its discharge into the environment. This synthesis aims to serve as a valuable resource for researchers, practitioners, and policymakers seeking a holistic understanding of bioinspired nanomaterials and their role in sustainable water management practices.
In the last few decades, the field of interdisciplinary study known as nanotechnology has grown tremendously, acquired interest worldwide, and influenced various aspects of human existence [Citation30,Citation31]. Researchers have shown a considerable amount of interest in metal nanoparticles due to the unique physicochemical properties that they possess. They open up various applications in various fields, including industry, optics, the medical area, materials research, multi component reaction and organic transformations [Citation32–39]. Industrial waste, a non-degradable pollutant from industries, is harmful to the environment and has a negative impact on the living systems of modern society. Because of rapid industrial expansion and agronomic operations, water becomes contaminated and unsanitary; this water is primarily utilized in the lives of disadvantaged people [Citation40,Citation41]. Nanotechnology-based Materials have great potential in the remediation of these pollutants due to their larger surface-to-volume ratio. As a result, nanomaterials have improved reactivity and are more effective than their bulkier counterparts in various applications. In addition, nanomaterials can harness distinctive surface chemistry compared to conventional methods for degrading toxic compounds. In addition, the purposeful manipulation of nanomaterials’ size, shape, porosity, and surface area can bestow additional positive features that directly impact the material’s performance for environmental remediation [Citation42].
This comprehensive review paper thoroughly explores diverse approaches to water remediation, encompassing both chemical and biotechnological methods, and underscores various water treatment techniques. Significantly, the paper delves into the transformative role of nanotechnology in augmenting water treatment efficiency, presenting it as an innovative and promising solution. Extensive attention is given to the synthesis of environmentally friendly nanomaterials and their intricate applications in environmental remediation. Notably, the application of nanomaterials in water remediation has exhibited promising results by efficiently eliminating water pollutants without causing harm to the environment. The review underscores the imperative for further research to unlock the full potential of nanotechnology in effectively addressing the global water scarcity challenge.
2. Methodology
A systematic review of peer-reviewed articles was conducted using electronic databases such as PubMed, Web of Science, and Scopus. Firstly, the sources of the water contamination were discussed. Keywords such as water contamination through ‘industrial waste’, municipal waste”, ‘agricultural waste’, and ‘human waste/domestic waste’ were used to fetch the relevant references. Upon understanding the various sources of water contamination and the types of pollutants, various methods of water remediation were analyzed and discussed, and the key challenges and the future scope were identified and discussed. Keywords related to water remediation, including ‘chemical treatment’, ‘bioremediation’, and ‘nanotechnology’, were used to retrieve relevant literature published mostly between 2010 and 2023. Some of the important references on ‘Chemical treatment’ published before 2010 have also been discussed to understand the progress in water remediation with the advancements in biotechnological and nanotechnological processes. The retrieved articles were screened based on their focus on water remediation through chemical treatments and subsequent advances in the water remediation process through biotechnological and nanotechnological approaches. Key findings were synthesized to elucidate trends, challenges, and emerging developments in water remediation strategies across different perspectives.
3. Source of water contamination
Wastes come in many forms, and they can pollute the environment. Many environmental impacts and exposures result in the production of many types of garbage. Municipal and industrial wastes are the two main sources that are mostly to blame for the deterioration of the environment. Alternatively, trash can be categories based on the origin and types of pollutants. Various sources of water contamination are highlighted in .
Figure 1. Sources of water contamination – a comprehensive overview of factors contributing to water pollution, including industrial waste, agricultural waste, municipal waste and human waste.
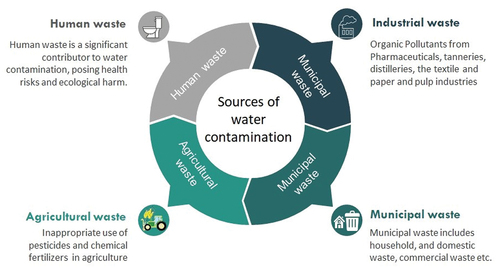
Municipal waste, such as municipal garbage, is a significant origin of several pollutants containing dissolved solids and suspended particulate matter. It includes a huge amount of metals and nitrogenous elements that are persistent in organic compounds. Waste material and products produced from household, and domestic waste, are released openly into water bodies and their soil surroundings. This can result in serious aesthetic issues with odor and discoloration. Water can become unfit for use if it contains disease-causing organisms (bacteria and viruses). The tannery sector is the largest producer of treated leather components, which is classified as a red-category business because of its high contamination levels. Because of its enormous applicability for export and engagement of workers, it significantly contributes to the socio-economic equity of a nation [Citation43–45]. It is the oldest process for turning raw material into prized things but requires a lot of fresh water and much of this water is discharged as effluent in enormous amounts, which is bad for the environment [Citation46,Citation47]. Hazardous metals (mostly chromium), colored combinations, and other contaminants are in significant quantities in tannery wastewater [Citation15,Citation26,Citation43]. Tannery effluent has significant carcinogenic, mutagenic, and toxicological effects that are irreversible on the critical bodily structure and exceedingly harmful to the marine and human environment [Citation14,Citation47,Citation48]. Chromium is notably toxic at high exposure levels and negatively impacts plants and other living things [Citation15,Citation49].
Due to the widespread usage of alcohol in chemicals, medicines, cosmetics, food, and the fragrance sector, among other industries, the production of alcohol is growing rapidly. These industries are heavy polluters of the environment, generating 8 to 15 L of seepage for each 1 L of alcohol production [Citation50]. Approximately 300 molasses-based distilleries in India produce 40 billion liters of wastewater yearly [Citation51]. Typically, distilleries that produce trash, known as treacle wash, employ molasses as a raw material because of its low cost. Molasses spent wash (MSW) seriously harms the ecosystem because it contains significant levels of melanoidin [Citation52–54]. Dark brown color in wastewater was due to the formation of complex polymers of melanoidin molecules. These distilleries effluents have high oxygen demands, acidic pH, and high dissolved solids (DS) concentrations [Citation55–57]. Due to the presence of putrescible organics, sulfur, and aromatic alcohols, an enormous quantity of IWW is produced by distilleries. Persistent and toxic contaminants have a deadly impact on microbial societies, fish, and other planktonic organisms [Citation54,Citation58].
Pulp and paper industries use high amounts of chemicals, energy and water, requiring between 4,000 and 12,000 gallons per tonne of pulp [Citation59]. Wastewater from pulp and paper industries has a series impact on the environment [Citation60]. The textile industry uses high amounts of fresh water, which has caused significant pollution issues. The textile, apparel, and dying industries utilize about 700,000 tonnes of various dyes and nearly 8000 chemicals to make textile products [Citation61]. It contains harmful dyes, chlorine traces, persistent organic pollutants (POPs), dioxins, and pesticides; among other things, wastewater from the textile and fabric manufacturing sectors can negatively affect the surroundings [Citation62]. Because raw ingredients and dyes are used, the effluent from textile yards is extremely black in color and contains dissolved solids. Effects of such a form of fabric effluents released in the environment are liable to increase the pollutants and the degradation of water and soil characteristics, which would frequently be hazardous to humans [Citation63].
The industrial field, the food and beverage industry, has a significant role in economic growth. Waste from these industries, which is primarily made up of leftover organic compounds from used materials, uses a lot of water. Hence, the wastewater these factories discharge varies significantly from industry to industry and even within the same industry sector, depending on the products and production processes used [Citation64]. The food business comprises several industries: processing meat, producing dairy products, making beer and wine, processing grains, and growing fruits and vegetables [Citation65]. Wastewater from these industries is highly polluted and contains a lot of particulates [Citation66]. Because of this, wastewater has significantly greater percentages of BOD and COD, and its direct discharge into the environment results in serious pollution issues [Citation67]. Due to substantial chemical and water use caused by a severe effluent management problem that might affect the environment, the meat industry significantly contributes to the deterioration of the atmosphere [Citation68].
Organic Pollutants from Pharmaceuticals, tanneries, distilleries, the textile and wood preservation industries, as well as the inappropriate use of pesticides in agriculture, all produce phenolics and their organic waste. Volatile compounds (VOCs), are released into the atmosphere by a manmade and natural actions. At high pressure and room temperature, they are quite reactive. Such compounds are made by the metal mining and finishing, petrochemical, automotive, and some chemical production industries [Citation69]. The small concentration of VOCs has a severe negative effect on the health of humans. In addition to reducing reproductive potential and causing reproductive problems like respiratory issues and birth abnormalities, they also have neurotoxic and genotoxic effects [Citation70–72].
For a hygienic and positive atmosphere, the process of water remediation and maintaining a good environment is necessary. There are numerous technologies developed by scientists and adopted by industries; some are described in detail as follows.
4. Water treatment technologies using physical treatment
4.1. Adsorption
Adsorption is a traditional physical technique that effectively treats and recycles the organic, metallic, and chlorinated contaminants from IWW [Citation73]. It entails a phase transfer procedure wherein contaminated materials are typically transferred from the liquid and gas phase into a solid surface and bonded via chemical or physical interactions [Citation74]. Electrostatic force and Van der Waals force contribute to physio-sorption or chemisorption. The best materials for IWW treatment include various low-cost and modified materials [Citation75–80].
4.2. Electro-dialysis
Electro-dialysis (ED) is a membrane-based separation technique for controlling hazardous inorganic pollutants by separating anions and cations. ED is an effective approach for eliminating inorganic impurities from water [Citation81]. The membranes used in this technique are polymers derived from monomers such as ethylene and styrene [Citation82,Citation83] and contain ion-exchange resins that facilitate the transport of ions. In the ED process, an electric current is applied to an aqueous metal solution, causing the migration of cations toward the negatively charged electrode [Citation84].
However, the ED technique requires frequent preprocessing before application. Factors such as membrane quality, current density, assembly of the ED cell, water ion concentration, and flow rate significantly impact the efficiency of ED [Citation85]. ED can effectively address hazardous pollutants present in industrial wastewater (IWW) as ions and solids and also enable the recovery of important metals such as copper and chromium. Nonetheless, degradation and fouling of the membrane pose a significant drawback that hinders ion transport [Citation86].
4.3. Ion exchange
Premature and unwanted ions from IWW can be treated using a new, more alluring method called ion exchange. Ion exchange resin has a network structure made of minerals and carbon with linked functionalities. However, synthetic resins are more frequently utilized as ion exchange matrices () [Citation87]. Negative ions are exchanged in Anionic resins, while cationic resins do the opposite. These resins are created by polymerizing organic materials into a three-dimensional porous framework. Hydroxyl ions, Amines are used to produce Anionic, while cationic resins contain acidic functionality. By this remediation method, the heavy metal ions Cr6+, Cu2+, Zn2+, Pb2+ and Cd2+ can effectively detoxify [Citation88,Citation89]. It is widely employed in the plating business, where water reuse and chromium recovery have led to significant cost savings.
Figure 2. A new hybrid process for separation of heavy metal ions from contaminated water using integrated processes combining metal bonding [Citation87].
![Figure 2. A new hybrid process for separation of heavy metal ions from contaminated water using integrated processes combining metal bonding [Citation87].](/cms/asset/3e2d8825-0379-456b-bc30-39dbe92c05f0/tcsb_a_2329660_f0002_b.gif)
4.4. Sedimentation
Gravitational settlement is a commonly used physical, operational treatment method called sedimentation that removes large, heavy particles retained in wastewater [Citation90]. It continues in a clarifier, a settling container. It operates based on gravitational force and the gradient of elemental densities. It is frequently used to separate grit and large solid contaminants from wastewater based on their densities and settles them in the primary sedimentation chambers. For the treatment of IWW, three primary types of deposition designs are used: horizontal flow, solid contact, and inclined surface [Citation91].
5. Treatment technologies using chemicals
5.1. Coagulation/flocculation
To clarify IWW containing colloidal, the coagulate method is used. Using a coagulating agent, like alum, IWW comprises mixed oils and paperboard waste simply clear by coagulation. There are a variety of substances that function as coagulating agents, including silica, ferrous sulphate (FeSO4), and ferric chloride (FeCl3), etc., have been used for the removal of poisonous pollutants as well as solid residues from IWW [Citation92]. Coagulating substances are pH-specific; when the pH is low, they frequently cause an emulsion to disintegrate.
5.2. Precipitation and filtration
The separation of dispersed and dissolved sulphates, carbonates, chloride pollutants, and metallic elements from IWW is mainly accomplished through precipitation and filtration. Typically, metals are precipitated through lime as hydroxide (NaOH). Metal salts that form complexes with polluted substances, such as ferric chloride and aluminum sulphate, are frequently used in chemical precipitation as precipitated agents [Citation93]. Chemical precipitations are a dependable and affordable approach; however, it heavily depends on the wastewater’s pH value [Citation94]. This well-known method removes phosphorus excessively. Further, a different filtration or clearing procedure removes the precipitates from the effluent.
5.3. Oxidation
Due to the efficient breakdown of phenolic, chlorinated, and organic contaminants, which are mostly present in industrial wastes, the oxidation approach associated with Fenton’s reagent is gaining prominence [Citation55]. Oxidation by Fenton’s process is a straightforward and appealing technique that, thanks to its capacity to function at low pH levels, boosts the biocompatibility of polyphenols, organic halides, total organic carbon, and insoluble contaminants. Fenton’s procedure is widely used to refine wastewater, remove the color from lignocellulosic materials, and potentially decolorize textile dyes [Citation95,Citation96]. The primary benefit of this method is its simplicity, as it does not require any additional complicated equipment for oxidation, and its chemicals are environmentally safe, which impacts the high elimination rate of hazardous chromophoric substances [Citation97,Citation98].
6. Biotechnological treatment methods
Microorganisms (mostly bacteria and fungus) are used in biological treatment procedures in particular to handle toxic organic waste; contaminants can easily transform into flocculent/settable compounds and gases. Physical and chemical therapy methods are less suited and unsafe than biological treatment methods. Sludge processing is a common aerobic process for treating large volumes of water waste, primarily used to eliminate solid organic contaminants. Sewage treatment plants (STPs)/common and mixed sewage processing, a conventional approach for the elimination of industrial waste, and discharge treatment plants of industry, ASP is the approach that is used the most frequently in developing nations. It involves suspending fungal, bacterial, or biomass of algae, which is primarily responsible for the breakdown and decay of organic materials [Citation9]. In an aeration basin, microbes are often cultivated in the existence of air and organic waste that they can consume. These microorganisms also cause floc development, which causes solid waste to acclimatize at the lowermost position of the chamber and clear wastewater liquid to collect at the topmost position of chamber. It is a promising technique, however, the two primary limitations of this effective technology are sludge thickening and foam creation.
For many years, anaerobic waste treatment techniques have been used extensively to recover municipal, industrial, and agricultural non-conventional energy returns. Anaerobic digesters have mostly been advocated for the more efficient and economical degradation of hazardous chemicals and substances for the treatment of solid and sludge STP waste and municipal garbage, as well as effluents released from possible harmful industrial processes.
7. Treatment technologies in development
Nowadays, various irritant impurities and contaminants are formed due to the increasing use of chemicals, pesticides, and herbicides in farming practices. These contaminants and chemicals are difficult to remove with conventional technological processes, so conventional processes have slowly lost their suitability for the efficient treatment of water waste and effluent. So, new and innovative technologies have been introduced, such as membrane filtration, sonication, photocatalysis, redox mediators, and enzymatic therapies. These developing technologies could offer better solutions to current IWW and public health treatments. Various water treatment technologies are summarized in .
Figure 3. Overview of advanced water treatment technologies, including chemical, biotechnological, and nanotechnological approaches for comprehensive water remediation.
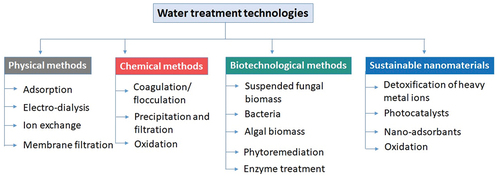
7.1. Process of advanced oxidation
Advanced oxidation processes (AOPs) are efficient methods of waste management that generate sufficient hydroxyl radicals for complete oxidation of chemical substances. The use of powerful oxidizing agents in AOPs considerably accelerates the degradation process [Citation99–101], making them increasingly popular as state-of-the-art treatment methods. AOPs employ various oxidation techniques such as Fenton oxidation, photooxidation, photo-Fenton oxidation, ozonation, and photocatalysis to treat industrial wastewater (IWW) by targeting the molecules produced after the decay of biological and hazardous components [Citation102]. These procedures can eliminate pollutants such as nitrites, pesticides, sulphides, and other chemical contaminants at a constant rate of 106–109 m1 s1 [Citation103]. AOPs have shown significant efficacy in reducing the toxicity and burden of organic contaminants in IWW, to the extent that the treated water can be reused. In some cases, AOPs have been capable of reducing pollutant concentration from 100 ppm to 5 ppb [Citation104].
7.2. Photo-catalysis
Photo-catalysis is a highly efficient and effective method for removing toxic contaminants from industrial wastewater (IWW) and is commonly used to eliminate organic and metallic waste [Citation105]. In terms of technological advancement, photocatalysis stands out among other oxidation processes. Many organic pollutants that absorb UV energy become energized and more reactive with chemical oxidants or undergo direct photolysis and degrade to some extent. As such, the photo-catalytic process is a unique and efficient method for the environmentally conscious removal of hazardous pollutants [Citation106].
7.3. Membrane filtration
The membrane separation process is a sophisticated method used to remove unwanted particles, microbial, sediments, and natural organic substances from wastewater. Due to their thinness and microporous ness, membranes act as a physical barrier separating the feed stream into retentate and permeating fractions [Citation107]. Ultrafiltration (UF), reverse osmosis (RO), microfiltration and nanofiltration (NF) are some of the different types of processes of membrane filtration. These processes depend on the membrane type, size of the pore, as well as charge used in solid/liquid and liquid/liquid treatment. Relative to other barrier techniques, these NF and UF barriers provide substantial advantages for treating industrial and surface wastewater due to their high flux, superior chemical resistance, and large pore size [Citation108]. However, RO primarily treats inorganic pollutants due to ionic diffusion. The membranes have high transparency and specificity and are prepared from organic polymers like polyethylene, polysulfones, acetates and ester of cellulose, polypropylene, polyether ketones, and polyimide/polyamide among others, and have great transparency and specificity [Citation107]. To remove color from aqueous media, membrane processes are successfully beneficial in various net operations of tanning processes. Koyuncu (2002) reported on the important application of NF membranes and used it for the elimination of > 99% hazardous and color compounds from the textile industry by enhancing the salt amount [Citation109]. Schokker and co-workers (2002) created a two-step NF technique that could recover up to 90% of trash while lowering the color content by 99.8% [Citation110].
7.4. Enzymatic treatments
Enzymes are biological agents that act as catalysts in converting raw materials into finished goods by establishing advantageous conditions that lower the reaction’s activation energy. Biological methods, including enzymatic digestion, are significantly superior, safer, and more successful for environmental remediation as compared to chemical and physical treatments [Citation111]. Enzymes are only relevant for extremely high concentrations of resistant harmful pollutants, with a variety of substrates, pH levels, temperatures, and minimal biomass generation. They are also highly selective and have a low danger of producing secondary pollutants [Citation112,Citation113]. Enzymes are very effective and more desirable from an environmental standpoint because they have adaptability, biodegradability, and selectivity to break down contaminants [Citation114,Citation115]. Degradative enzymes from plants and microorganisms, such as oxygenases, laccases, tyrosinases, peroxidases, lipases, and cellulases, have recently been demonstrated to be effectively applicable and helpful for IWW therapies. These enzymes transform and reduce chlorinated and non-chlorinated, poisonous chemicals [Citation116–118]. Polymerizing, copolymerizing, and interacting with soluble organic or other substrates, oxidoreductase enzymes can detoxify phenolic, anilines, and hazardous xenobiotic chemicals [Citation119]. Due to their inexpensive cost and potential for processability for treatment applications, soybean peroxidase and Arthromyces ramosus peroxidase have also been described as promising enzymes [Citation112,Citation120]. Dealkylation, dehalogenation, reduction, and conversion and removal of pollutants are only a few of the chemical reactions that cytochrome P450 enzymes have demonstrated promise biotechnological and atmospheric uses for [Citation121]. Using fungi and bacterial agents, tannins and caffeine in coffee and tannery production waste are easily transformed into less harmful forms [Citation122]. Organophosphates can be treated using organophosphorus hydrolase [Citation123]. The detoxification of phenolic compounds uses enzymes secreted from fungal mycelium, including laccase, manganese peroxidase and lignin peroxidase [Citation116]. Laccase enzymes may facilitate the degradation of pollutants, enhancing their uptake by soil microbes and thereby promoting bioremediation and waste detoxification. The potential contribution of laccase to pollutant detoxification is illustrated in . Extracellular hydrolases offer a variety of possible uses in different commercial sectors [Citation124].
Figure 4. Role of laccase enzymes and their natural phenolic mediators in soil bioremediation and detoxification of industrial waste [Citation116].
![Figure 4. Role of laccase enzymes and their natural phenolic mediators in soil bioremediation and detoxification of industrial waste [Citation116].](/cms/asset/e7b77ffd-53d9-4396-a4e8-58b912437aa5/tcsb_a_2329660_f0004_b.gif)
8. Industrial pollutant remediation using green technology
8.1. Bioremediation
Regarding waste management, bioremediation is a revolutionary, advantageous, economical, and ecologically friendly choice for decontaminating polluted soil and wastewater containing hazardous and harmful contaminants. This natural process reduces and degrades hazardous pollutants by mineralizing them or converting them into less dangerous chemicals by using the metabolic power of living things, primarily bacteria in either ex situ or in situ circumstances [Citation125]. The word ‘bioremediation’ encompasses an extensive range of green technologies, including phytoremediation, land farming, bioventing, composting, rhizofiltration, bioaugmentation, biostimulation, and bioleaching. The basic knowledge regarding bioremediation is provided by microorganisms (fungi and bacteria) able to thrive and live in polluted settings, which can be significantly altered via numerous abiotic and biotic variables in direct or indirect ways. Absorption, biostimulation, and bioaugmentation are the three main stages of bioremediation [Citation126]. In the attenuation phase, native natural bacteria remove pollutants and harmful chemicals without the aid of any anthropogenic actions. The second is the biostimulation process, which uses oxygen and nutrients to increase the efficiency of the biodegradation process. During the bioaugmentation phase, other bacteria that may potentially degrade target materials were added to the system’s contaminants more effectively than the local flora. Bacteria like Pseudomonas, Bacillus, Alcaligenes, Micrococcus, Sphingomonas, and Mycobacterium can degrade and remove pesticides, hydrocarbons, and polyaromatic chemicals, and detoxify hazardous metals, including Cu, Cr, Ni, Pd, and As is described [Citation93].
8.2. Phytoremediation
The term ‘phytoremediation’ can also refer to a plant-mediated treatment [Citation127]. It replaces conventional repair and removal methods using the complete plant and its parts. It is a green technology based on plants that can store significant amounts of toxic substances. Phytoextraction, phytostabilization, rhizofiltration, phytovolatilization, and phytodegradation are the five primary physiological plant processes through which phytoremediation technology is a helpful instrument for improving the environment and understanding the interaction between plants and microbes and utilizing these beneficial microbial relationships in the rhizosphere of plants to remove, degrade, or detoxify dangerous contaminants from the environment is a somewhat novel and helpful technology. In particular, phytoremediation offers useful support for a clean, natural environment. The physiological processes leading to the removal of toxic substances are shown in .
8.3. Systems for engineered wetlands
The built wetlands (CWs), which are environmentally favorable, are created to filter highly hazardous contaminants from IWW in a sustainable way. By utilizing the natural biogeochemical processes of soil microorganisms, CWs are employed for the treatment of IWW to restore the quality of wastewater. In CWs, plant-associated microbes are essential for detoxifying and transforming toxic contaminants into less toxic ones [Citation128–130]. It is an aesthetically pleasing and economical method for cleaning up a variety of waste materials from home wastewater and IWW [Citation130]. A rhizospheric microbial population found in abundance in wetlands is crucial for removing non-essential contaminants and nutrients from IWW [Citation131]. According to earlier research, CWs are efficient at reducing heavy metal pollution. Aquatic macrophytes with the potential to detoxify or remove heavy metals, such Typha, Phragmitis, Eichornia, Azolla, among others, are taken into consideration for potent wetlands [Citation132]. Benefits and drawbacks of traditional and newer treatment technologies every treatment method has some restrictions on how they can be used to manage wastewater.
9. Applications of nanoparticles in water remediation
Based on all available technologies, it is estimated that while nanoparticle utilization is growing in various emerging fields, it is also applicable in environmental remediation.
There have been various attempts in recent years to create the most efficient and affordable adsorbents to eliminate hazardous metal ions. Fly ash, titanium oxide, red mud, sawdust, clay montmorillonite, yeast, biomass materials and microorganisms are a few of these adsorbents. These substances work well as adsorbents and can be removed from the system to be used again. The advantages of nanoparticles (NPs) include ease of synthesis and the ability to anchor necessary functionality on their interfaces. These NPs could be used in a variety of separation, biomedical, and environmental scientific fields. By adding functionality to the surfaces of these NPs, they can be transformed into selective adsorbents [Citation133].
Many physical and chemical processes, such as ion sputtering, laser ablation, solvothermal synthesis, sol-gel, and the chemical reduction approach, are used to create NPs. In general, top-down and bottom-up methods are used to synthesize nanoparticles. Bottom-up approaches use molecular building blocks that are assembled into increasingly complicated assemblies, whereas top-down approaches use larger, externally controlled devices to direct the production of nanoscale things. The top-down method frequently uses microfabrication processes, in which materials are cut, milled, and shaped using tools controlled from outside the system. Inkjet printing and photolithography are well-known examples of top-down approaches in micropatterning. Contrarily, bottom-up methods make use of single molecules’ inherent abilities to self-assemble into useful conformations [Citation134].
10. Sustainable nanomaterials for environmental remediation
Physical and chemical methods both have advantages, but ‘Green’ chemistry and biochemical methods are gradually merging with contemporary advancements in science and technology, in line with efforts made on a global scale to limit the creation of hazardous waste and develop energy-efficient manufacturing methods. So, by using nontoxic chemicals and ecologically friendly solvents, the fundamentals of ‘green chemistry’ should be followed by any synthetic procedure or chemical pathway. This green chemistry technique, known as ‘bioinspired nanoparticles’, is used to create the nanoparticles. According to green chemistry perspectives, the green process for NP synthesis should involve three main steps: Choosing a biocompatible and environmentally friendly solvent solution, eco-friendly reduction agents and nontoxic materials to stabilize the nanoparticles are the first three steps. The creation and processing of nanosized devices and nanomaterials that are intrinsically safer will be made easier by applying these ideas to nanoscience. Hence, green nanotechnology attempts to apply green chemistry ideas to creating nanoscale products and to develop ways for producing nanomaterials that produce less hazardous waste and have safer applications.
Biochemical reactions, facilitated by biocatalysts, exhibit remarkable selectivity even at low temperatures, leading to constant energy savings and reduced environmental impact compared to conventional processes. Incorporating one or more biological phases into synthetic pathways can optimize nanoparticle production, making bio-based technologies preferable due to their potential to mitigate the hazardous conditions associated with conventional manufacturing processes. Nature-inspired approaches, such as biomineralization, offer improved methods for assembling nanomaterials. Biomineralization involves biologically regulated processes in living organisms, resulting in highly efficient and tightly controlled syntheses. Biomolecular templates interact with inorganic materials at the nanoscale, yielding exceptionally effective products. Examples of biomineralized materials include siliceous resources from sponges and diatoms, apatite in vertebrates, and calcite or aragonite in invertebrates. These biominerals, combined with organic polymers, form structural elements such as bones in mammals, birds, and seashells. Leveraging biomineralization techniques in nanoparticle production holds promise for achieving sustainable and environmentally friendly synthesis methods [Citation135].
11. Green methods for the preparation of nanomaterials
Plant extracts, microbes, and biopolymers are a few examples of natural resources that can be used to synthesize nanomaterials. These techniques are inexpensive, biocompatible, non-toxic, and simple to synthesize, among other benefits. Phytochemicals such as polyphenols, flavonoids, and terpenoids, which function as reducing agents and stabilizers for the formation of nanoparticles, are abundant in plant extracts. Extracellular enzymes that function as reducing and stabilizing agents for the creation of nanoparticles can be produced by microorganisms like bacteria and fungi. Chitosan, cellulose, and starch are a few examples of biopolymers that can function as stabilizers and reducing agents during the manufacture of nanoparticles. Various biological sources used to synthesize metal NPs are summarized in . Some methods to synthesize nanomaterials with a green chemistry approach are discussed below.
11.1. Using microbial system
Microorganisms manufacture nanoparticles by capturing target ions from their environment and using enzymes created by cell processes to convert the metal ions into the element metal. The process can be separated into extracellular and intracellular synthesis depending on where nanoparticles are produced. The applications of various microorganisms-mediated nanoparticles are summarized in .
Table 1. Preparation of NPs by using microbes and their applications.
Moreover, bacterial strains have been used to demonstrate bimetallic Ag-Au nanostructures [Citation148]. Recently, it was discovered that a variety of filamentous fungi are skilled in producing AuNPs. For the biosynthesis of AuNPs, multiple techniques were used in this study. The scientists suggested that the components of the fungal media and the fungal compounds may have contributed to the stabilization of the nanoparticles [Citation149]. In another study, three different fungal strains Fusarium oxysporum, Aureobasidium pullulans, and the Fusarium sp. were employed to biosynthesize AuNPs. Authors claimed that the reducing sugars were used to modify spherical AuNPs during the biosynthesis, which took place in the vacuoles of fungi. Also, they showed how specific fungal proteins functioned in the capping of the Au NPs [Citation150].
Extracellular enzymes produced by several fungi, including cellobiohydrolase D, acetyl xylan esterase, -glucosidase, and glucosidase, are recognized to be crucial in manufacturing metal NPs.
11.2. Using proteins and templates like DNA
Bio-extracts from various microorganisms, including bacteria, fungi, and algae, are used in the environmentally friendly synthesis of nanoparticles as a reducing and seldom capping agent. Filamentous fungi are more effective biomass producers in bioreactors than algae and bacteria because their mycelial mats can survive flow pressure, agitation, and other environmental factors. Reductive proteins secreted extracellularly make it easier for silver nanoparticles to form outside of cells while minimizing undesired cellular interference, making them suitable for use in a range of applications [Citation151]. By adjusting the sequencing and structure of the DNA scaffolds, site-specific growth of ultrasmall nanomaterials, such as Ag, Au, and Cu NCs, may even be accomplished [Citation152–155].
11.3. Using plant extract
Plant flowers and leaves can be utilized to create nanoparticles if they are well-cleaned with nonionizing water and allowed to air dry. The dry plant sample will then be weighed and crushed. The plant extract is then diluted with water to the required concentration, boiled, and stirred continuously. Whatman filter paper is then used to filter the resulting solution, with the clear solution portion being used for sampling (plant extract).
A silver metal ion solution and a reducing biological agent are required to produce environmentally friendly silver nanoparticles. Reducing and stabilizing silver ions by a fusion of biomolecules such as vitamins, polysaccharides, amino acids, saponins, proteins, terpenes, alkaloids, and phenolics is the simplest and most economical approach for producing silver nanoparticles [Citation156].
Eco-friendly bio-organisms found in plant extracts are used to make shape-controlled, stable silver nanoparticles by acting as a reducing as well as a capping agent. Polymers and Surfactants added to silver nanoparticles showed significant microbiological activity against Gram-positive and Gram-negative bacteria. Several bacterial species are used to assess the antibacterial activity of plant-derived nanoparticles [Citation157].
Several plants have been successfully employed to synthesize gold and silver nanoparticles in an extracellular manner. Lemongrass (Cymbopogon flexuosus), Geranium (Pelargonium graveolens), Cinnamomum camphora, Tamarind (Tamarindus indica), aloe vera, neem (Azadirachta indica), and the fruit extract of Emblica officinalis have shown potential in decreasing Au((0) [Citation158–166].
Moreover, alfalfa (Medicago sativa) biomasses, native and chemically modified hop biomasses, residual water from soaked Bengal gram bean, oat (Avena sativa) and wheat (Triticum aestivum) biomasses, and alfalfa (Medicago sativa) biomasses have all been used to create gold nanoparticles (Cicer arietinum) [Citation167]. Gold nanoparticles were found inside living plant components of Chilopsis linearis, Alfalfa (Medicago sativa) and Sesbania seedlings. For Ag and Ag – Au – Cu alloy NPs synthesis, alfalfa (Medicago sativa) [Citation168]. Sprouts and Brassica juncea germinating seeds were employed. The improvement of hygienic, nontoxic, and environmentally suitable ‘green chemistry’ processes, likely including species ranging from bacteria to fungi and even plants, will enhance the synthesis and assembly of metallic nanoparticles [Citation158–169].
12. Application of bioinspired nanoparticles in water remediation
The removal of harmful metal ions, filtration, and chemical degradation of dye in wastewater have shown to be the most successful approaches to the remediation of polluted water. Colloidal silver particles have been shown to have antimicrobial effects against both viruses and bacteria [Citation170,Citation171]. Thanks to their promising catalytic activity, they can also degrade potentially harmful synthetic dyes, including congo red, methylene blue (MB), methyl red, rhodamine-B and methyl orange [Citation170,Citation172,Citation173]. Also, it has been discovered that they work well as sensors for finding heavy metals like mercury and copper ions [Citation174]. Ag NPs can be produced chemically, physically, or biologically. Environmental harm results from chemical and physical synthesis methods using toxic and non-biodegradable reducing and stabilizing agents [Citation171]. As an alternative, biological synthesis, also known as ‘Green Synthesis’, of Ag NPs is seen to be a novel technique due to many benefits, such as environmental friendliness, ease of synthesis, the practicality of large-scale synthesis, and the lack of hazardous chemical agents [Citation171]. Ag NPs are synthesized using plant extracts or microorganisms in the biological method. From a commercial standpoint, plant-mediated green synthesis of Ag NPs is thought to be safer and more cost-effective than microbe-assisted synthesis because managing and preserving microbial cultures is typically time-consuming [Citation175].
In several studies, fresh plant extracts from plants like olive leaf, Ocimum sanctum (Tulsi), Amaranthus gangeticus (Chinese red spinach), Datura stramonium, Citrullus lanatus, coffee, Matricaria recutita (Babunah), Azadirachta indica (neem), Aloe vera, and others were used to successfully synthesized AgNPs [Citation164] Instead, AgNPs have been synthesized using vegetable and fruit wastes in an effort to lessen their significant environmental impact [Citation176]. Due to improper disposal, vegetable or fruit waste has become a critical environmental issue. With nearly 35% of the world’s total production, the Second-largest producer of cauliflower is India [Citation177]. Cabbage (Brassica oleracea var. Botrytis) is a dietary mainstay in India. Cauliflower leaves are traditionally removed from the vegetable floret before cooking and thrown away. Before being sold in vegetable stores, a lot of additional cauliflower leaves are also plucked and illegally disposed of. Its improper disposal leads to the unneeded development of harmful microbes. Due to their adverse effects, cabbage and other vegetable trash are being thoroughly investigated in an effort to find alternate uses for them, such as compost, biofuels, and so forth. Polyphenols, which have been described as possible reducing agents for the production of nanoparticles, are abundant in cauliflower leaves [Citation178].
Ag NPs can be produced from new cauliflower and have been shown in prior studies to have antibacterial and antifungal effects against pathogens like Klebsiella pneumoniae, E. coli, Staphylococcus aureus, Candida albicans, Bacillus subtilis, and Aspergillus sp [Citation179,Citation180]. Ag NPs created from fresh cabbage floret extract were discovered in another investigation to exhibit promising antioxidant and cancer-preventing properties [Citation181]. Many plant extracts can be utilized as reducing and capping agents for the creation of NPs, doing away with the requirement for risky chemical substances and other refining procedures [Citation182]. Azadirachta indica, Cissus quadrangularis [Citation183], Catharanthus roseus [Citation184] Phoma glomerata [Citation185], Elettaria cardamom, and [Citation186]. Lantana camara flower. In a green synthesis method, the Ag NPs are made by using the extracts of tomato (T), acacia catechu (C), onion (O), and combined extracts of COT as reducing and stabilizing agents. Also, the catalytic potential of each molecule created was examined for the MO, MR, and CR dyes as well as NaBH4 degradation. summarises the use of various plant extracts for the synthesis of metal and metal oxide nanoparticles and their applications in environmental remediation.
Table 2. Plant extract mediated synthesis of nanoparticles and their applications in environmental remediation.
Conversion of heavy metal ions into helpful end products is not possible. As a result, they must be collected before the waste water is discharged into the environment. The primary causes of hazardous ions are Hg, Pb, Zn, Cd, As, Cr, Ni, Cu, Mn, and others, including the tanning industries, paint, fertilizer, mining, and textile dyeing (Volesky, B. 1990) [Citation219]. The WHO sets the acceptable range of Cd and as in drinking water, at 0.003 mg L-1 and 0.01 mg L-1, respectively (A. Balci 1993; C. Reimann 2004) [Citation220]. Organic arsenic species have been found to have less harmful effects than inorganic arsenic species (C. K. Jain 2000) [Citation221]. By removing Zn from enzymes, Pb acts as an inhibitor (S. Skerfving 1998, M. Soylak 2002) [Citation222,Citation223]. Most amounts of heavy metals discharged into the environment surpass permissible limits. They are toxic and pose significant health concerns to people. shows the hazardous heavy metals and their negative impact on human health (Gong Z. et al. 2021) [Citation224].
Table 3. The hazardous heavy metal contaminants and their negative impact on human health.
This would also increase the risk of heavy metal ions accumulation above permitted levels, producing serious health problems shown in [Citation224,Citation225]. The practice of municipal wastewater for agricultural irrigation be inclined to cause toxic heavy metal accumulation in agricultural products and soils. Entitlement of city sewage water for agricultural irrigation has the potential to cause a high amount of accumulation of heavy metals in agricultural goods and soils [Citation226,Citation227]. These harmful heavy metals are conveyed and absorbed by plants from the territory through absorption. Toxic heavy metals harm vegetation and endanger human and animal health. At sufficient concentrations, toxic heavy metals convert into poisons, harming natural microbial populations and disturbing essential ecological processes [Citation228,Citation229]. Numerous scientific reports explained the consequences of hazardous heavy metals on plant and microorganism growth [Citation230–237]. It is vital to investigate a mechanism to desorb harmful metal/nonmetal ions from the environment and water due to their negative consequences on the environment and water. Researching a better method for detecting harmful substances and treating them appropriately is necessary. To date, various literature reports have been published to remove these harmful materials from wastewater. Functionalized polymer membranes have also been explored for heavy metal ion desorption by ultra/nanofiltration and adsorption [Citation238]. However, these membranes foul over time. Nano adsorbents with a high surface volume ratio, such as silica, alumina, and titania nanoparticles, are utilized to free from these ions [Citation239–242]. Furthermore, activated carbon is the best effective sorbent for removing these ions [Citation243,Citation244]. The difficulties in handling activated carbon and its high cost have prompted the quest for less expensive adsorbents. Clay montmorillonite and Red mud [Citation245,Citation246] were found to be a good sorbent for removing heavy metals from electroplating sawdust [Citation247], rice husks an agricultural waste [Citation248–250], coconut husk (Tan W. T. 1993) [Citation251], sugarcane bagasse, and other materials are among them [Citation252,Citation253], Besides biomass [Citation254,Citation255], fertilizer waste [Citation256], yeast and microorganisms were employed [Citation257]. Despite their low cost, these materials are not handy and not reusable. So, the need for worthier adsorbents based on metal-organic frameworks has been created to withdraw contaminants to obtain a high chemical selectivity [Citation258]. The abolition of pollutants has been studied using boron nitride-based materials having a graphene-like arrangement.
In-vivo nickel, zinc, copper, and cobalt nanoparticle production was also perceived from mustard, sunflower, and alfalfa. Plant leaf extracts, such as crown flower, coriander, and others, have also been utilized to make ZnO nanoparticles, which have been employed as sorbents for harmful metal ions [Citation259].
Sensing ions such as Hg2+, Cd2+, Cr3+, Zn2+, and Ca2+ in water was done by utilizing Ag NPs synthesized from diverse plant extracts. Similarly, AgNPs prepared from fresh and dried mango leaves (MD-AgNPs and MF-AgNPs) showed selective sensitivity for Pb2+ and Hg2+ [Citation260].
It is generally known that iron and iron oxide nanoparticles have higher dye reduction and removal catalytic activity. In recent decades, much interest has been in synthesizing metal nanoparticles for industrial uses using plant extracts, such as iron, silver, gold, magnesium, and platinum. Iron oxide nanoparticles are both safer and less expensive. Biological approaches were used to create iron oxide nanoparticles. These involve the use of various plant extracts. offers summaries of previous research projects.
One of the easiest, cheapest, and most successful methods for producing iron nanoparticles exists. Nanosized iron oxides have the potential to be promising metal ion binders in water remediation. It is due to their small distribution size and high surface area, allowing it to interact well with an extensive range of chemical species, both aqueous and gaseous. Changing the form and size of iron oxide during activation exposes the material’s most active catalytic sites, making it an additional efficient and economical catalyst for various processes. Adjusting the composition of iron oxides can also allow for the selective sorption of diverse metal ions. Metal ions from natural water streams or industrial waste are increasingly seen as appealing for adsorption or recovery using iron oxide nanoparticles [Citation261].
Lunge et al. successfully generated low-cost magnetite nanoparticles via tea trash. The crystal structure of magnetite nanoparticles is cuboid/pyramid-shaped. To remove the arsenic (As (III) and (V), the sorption ability of as-synthesized nanoparticles was considerably more significant than that of previously described adsorbents. The sorption capability of As (V) was 153.8 mg/g while As (III) was 188.69 mg/g [Citation262].
Rao et al. estimated the dimensions of a Fe0/Fe3O4 bio nanocomposite made from a yeast cell used to remove chromium (VI), which is a worthy biosorbent. Magnetically modified yeast cells have a three-fold higher sorption capacity than untreated yeast cells. Modified NCIM 3589 had a higher sorption capability. i. e. 186.32 mg/g than reformed NCIM 3590 (137.31 mg/g) at a starting chromium content of 1000 mg/L and under ideal circumstances [Citation263].
The preparation of zero-valent iron nanoparticles (ZVIN) by Madhavi et al. [Citation264] involves using an extract from the leaf of the Eucalyptus globules plant. ZVIN has been applied in the process of metal sorption for Cr (VI). Batch studies were used to study sorption parameters such as the amount of adsorbent to be used, its kinetics, and the initial concentration. At a reaction time of 30 minutes and a dose of 0.8 g/L, ZVNI demonstrated an extremely high sorption efficacy of 98.1%. The stability of iron nanoparticles (ZVIN) prepared from Plant extract is up to two months from the preparation, which was very interesting. Similarly, Savasari et al. [Citation265] used ascorbic acid to synthesize green ZVIN, which was used to reduce Cd(II) from water. These nanoparticles were found to be effective and stable.
Mystrioti et al. prepared a steady colloidal suspension of Zero Valent Iron NPs (nZVI) coated with polyphenol (obtained from green tea) and investigated the removal of chromium ions. The nZVI NPs of 5–10 nm were used to assess the abolition of Cr(VI) from contaminated groundwater [Citation266].
Iron oxide prepared from different plants, their size, and capacity for toxic metal ion removal are shown in .
Table 4. Iron nanoparticles prepared from plant, size and adsorption capacity.
Shaidatul Matussin and their co-authors released a paper on tin oxide biosynthesis NPs by utilizing diverse plants and their components and assessing their possible uses [Citation267]. In a review study on the production of zirconia nanoparticles using plant extracts and their applications, Amol Nikam and colleagues [Citation268] published their findings. A paper by Saba Pirtarighat and colleagues [Citation269] discusses the environmentally friendly production of silver nanoparticles utilizing Salvia spinosa plant extract. The availability of several reports on the synthesis of NPs using plant extracts demonstrated the breadth of applications. Still, only a few of these reports applied to detecting harmful metal ions. Metallic NPs, such as iron oxide, Nickel oxide, Co oxide, etc., are normally used as a sorbent material for toxic metal ions. Due to their magnetic nature, they can be readily disorbed from the water or aqueous medium. A review on Iron nanoparticle synthesis using plant extract and their uses as sorbents for water remediation was published by Segun Michael Abegunde et al. [Citation261].
Iron oxide NPs prepared from tangerine peel with sizes ranging from 50–20 nm show a 90% recovery of Cd. Eriobotrya japonica plant extract was also used to synthesize iron oxide NPs for the sorption of Cr. The rate of sorption was found to be 312.5 mg/g. Iron oxide NPs prepared from L. camara fruit extract showing the Ni sorption efficiency 227.20 mg/g [Citation261]. Biosynthesized iron NPs, Iron oxide NPs, Al-oxides, and composite materials containing iron minerals, such as Fe3O4 magnetite graphene oxide encapsulated in calcium alginate beads and Fe-modified activated carbon, have all been informed as efficient sorbents for the abstraction of Cr(VI) ions from water [Citation270].
Chromium and arsenic adsorption [Citation271] for nickel [Citation272] and for lead removal are some of the published papers on iron oxide nanoparticles based adsorbents. They also have considerable magnetic characteristics, which would aid in separating nanoparticles following adsorption. Magnetic separation is a viable alternative to classic separation procedures such as centrifugation and filtering in extracting nanoparticles from an aqueous solution. Cadmium removal from an aqueous solution can be observed by iron oxide NPs synthesized using tangerine peel extract, as explained by Mohammad Hassan Ehrampoush and colleagues. It has a high adsorption capacity for cadmium ions [Citation273,Citation274].
13. A comparative analysis
The result was comparable when we compared the capability of NPs synthesized using biological and traditional (chemical and physical) methods ().
Table 5. Comparative analysis of toxic ion absorption capabilities of NPs synthesized using traditional and biological methods.
As we compare the results of toxic ions sorption, it is observed that the result is comparable with the traditional methods.
14. Challenges in nanomaterials based water remediation
Nanotechnology has emerged as a promising approach to effectively and efficiently remove toxins from water during remediation processes. Despite its immense potential, the field faces some challenges that must be overcome. One of the major concerns is the potential toxicity of nanoparticles to the environment and human health. However, with proper regulation and quality control, nanoparticles that are safe for the environment and human health can be developed. Synthesis of nanomaterials using various biological sources, such as plant extracts, microorganisms, plant polysaccharides, phytochemicals, etc., could efficiently produce nanomaterials with environmentally benign processes. Another challenge is the high cost of nanomaterials and the production process, but with continued research and development, costs can be reduced, making the technology more accessible.
Additionally, stability and efficient dispersion of nanoparticles in water is critical for ensuring long-term effectiveness, and research efforts are underway to address these issues. Increasing the production of nanoparticles for large-scale water treatment while maintaining their quality and stability is another challenge. Standardizing testing procedures and regulating nanoparticles in water treatment is also a crucial issue that requires careful consideration. With concerted efforts to address these challenges, nanotechnology-based water remediation has the potential to play a significant role in addressing the global problem of water scarcity.
15. Conclusion
Water remediation methods, encompassing chemical, technological, and nanotechnological approaches, represent vital strategies for mitigating water pollution and ensuring access to clean water resources. Chemical methods, while effective in removing a wide range of contaminants, often suffer from drawbacks such as the formation of harmful byproducts, high costs, and limited effectiveness against certain pollutants. In contrast, technological methods, including enzyme technology and microbe-mediated bioremediation, offer more environmentally friendly and cost-effective alternatives by harnessing natural processes for targeted degradation of contaminants. However, challenges remain in optimizing their efficiency and scalability for widespread implementation. Nanotechnology, with its ability to enhance adsorption, catalysis, and membrane technologies, presents promising opportunities to overcome these limitations and revolutionize water remediation efforts. By leveraging the unique properties of nanoparticles, such as their high surface area-to-volume ratios and catalytic capabilities, nanotechnological approaches offer innovative solutions for improving water treatment efficiency, reducing environmental impacts, and promoting sustainable water management practices. Comparative analysis reveals that while chemical methods provide rapid treatment, technological and nanotechnological methods offer more sustainable and targeted approaches with potential long-term benefits. Future research directions should focus on advancing nanotechnology-based water remediation technologies, including the development of novel nanoparticles with enhanced adsorption and catalytic properties, as well as the integration of nanomaterials into scalable and cost-effective water treatment systems. Additionally, interdisciplinary collaborations between scientists, engineers, and policymakers are essential to address regulatory and implementation challenges and facilitate the translation of research findings into practical solutions for addressing global water challenges.
Author’s contribution
PBR and MPS: Literature review, Writing-original draft, review and editing. AST and SUD: literature review. CKT, AK and ABK: Conceptualization, literature reviewing, writing and editing of the manuscript. All authors have read and agreed to the published version of the manuscript.
Acknowledgment
Dr. Anant B. Kanagare thanks to The Principal, Deogiri College, Chhatrapati Sambhajinagar, Maharashtra, India for providing the necessary infrastructure and research facilities.
Disclosure statement
No potential conflict of interest was reported by the author(s).
Data availability statement
Data sharing does not apply to this article as no datasets were generated or analyzed during the current study.
References
- Yadav A, Chowdhary P, Kaithwas G, et al. Toxic metals in environment, threats on ecosystem and bioremediation approaches. In: Das S, Dash HR, (editors) Handbook of metal microbe interactions and bioremediation. Boca Raton, Florida: CRC Press, Taylor & Francis Group, Boca Raton 2017. p. 813.
- Zainith S, Sandhya S, Saxena G, et al. Microbes an ecofriendly tool for the treatment of industrial wastewater. In: Singh JS, Singh DP, editors. Microbes and environmental management. New Delhi: Studium Press (India) Pvt. Ltd; 2016. p. 78–250.
- Gricic I, Vujevic D, Sepcic J, et al. Minimization of organic content in simulated industrial a wastewater by Fenton type processes: a case study. J Hazard Mater. 2009;170(2–3):954–961. doi: 10.1016/j.jhazmat.2009.05.060
- Narmadha D, Kavitha SVM. Treatment of domestic wastewater using natural flocculants. Int J Life Sci Biotechnol Pharma Res. 2012;1(3):206.
- Kanagare AB, Singh K, Kumar GK, et al. Synthesis of potassium nickel hexacyanoferrate encapsulated polymeric beads for extraction of cesium. Int J Innov Res Sci Eng Technol. 2016;5:265–273. doi: 10.15680/IJIRSET.2015.0501033
- Shivajirao AP. Treatment of distillery wastewater using membrane technologies. Int J Adv Res Stud. 2012;1(3):275–283.
- Rajasulochana P, Preethy V. Comparison on efficiency of various techniques in treatment of waste and sewage water – a comprehensive review. Resour Efficient Technol. 2016;2(4):175–184. doi: 10.1016/j.reffit.2016.09.004
- Kanagare AB, Ajish JK, Singh K, et al. Synthesis of ionically cross-linked N-Succinyl chitosan hydrogel beads for recovery of palladium from acidic aqueous solution. Asian J Mater Chem. 2017;2(2): 60–68. doi: 10.14233/ajmc.2017.AJMC-P39
- Kumar BL, Gopal DS. Effective role of indigenous microorganisms for sustainable environment. 3 Biotech. 2015;5(6): 867–876. doi: 10.1007/s13205-015-0293-6
- Prasad KS, Gandhi P, Selvaraj K. Synthesis of green nano iron particles (GnIP) and their application in adsorptive removal of As(III) and As(V) from aqueous solution. Appl Surf Sci. 2014;317:1052–1059. doi: 10.1016/j.apsusc.2014.09.042
- Shu HY, Chang MC, Yu HH, et al. Reduction of an azo dye acid black 24 solution using synthesized nanoscale zerovalent iron particles. J Colloid Interface Sci. 2007;314(1):89–97. doi: 10.1016/j.jcis.2007.04.071
- Shin S, Yoon H, Jang J. Polymer-encapsulated iron oxide nanoparticles as highly efficient Fenton catalysts.Catal. Commun. 2008;10(2):178–182. doi: 10.1016/j.catcom.2008.08.027
- Xu L, Wang J. A heterogeneous Fenton-like system with nanoparticulate zero-valent iron for removal of 4-chloro-3-methyl phenol. J Hazard Mater. 2011;186(1):256–264. doi: 10.1016/j.jhazmat.2010.10.116
- Li X, Elliot WL, Zhang W.Zero-valent iron nanoparticles for abatement of environmental pollutants: materials and engineering aspects. Crit Rev Solid State Mater Sci. 2006;31(4):111–122. doi: 10.1080/10408430601057611
- Ajay KG, Mona G. Synthesis and surface engineering of iron oxide nanoparticles for biomedical applications. Biomaterials. 2005;26(18):3995–4021. doi: 10.1016/j.biomaterials.2004.10.012
- Juliane B, Julian B, Rainer UM. Iron oxide nanoparticles in geomicrobiology: from biogeochemistry to bioremediation. New Biotechnol. 2013;30(6):793–802. doi: 10.1016/j.nbt.2013.03.008
- Chen C, Jiang X, Kaneti YV, et al. Design and construction of polymerized-glucose coated Fe3O4 magnetic nanoparticles for delivery of aspirin. Powder Technol. 2013;236:157–163. doi: 10.1016/j.powtec.2012.03.008
- Ana LS, Riansares MO, Jon SL, et al. Nanoparticles: a global vision. Characterization, separation, and quantification methods. Potential Environ Health Impact Anal Methods. 2014;61:38–56. doi: 10.1039/C3AY40517F
- Yin B, Ma H, Wang S, et al. Electrochemical synthesis of silver nanoparticles under protection of Poly(N-vinylpyrrolidone). J Phys Chem B. 2003;107(34):8898–8904.
- Bagade AV, Pund SN, Nagwade PA, et al. Ni-doped Mg-zn nano-ferrites: fabrication, characterization, and visible-light-driven photocatalytic degradation of model textile dyes. Catal Commun. 2023;181:106719. doi: 10.1016/j.catcom.2023.106719
- Smetana AB, Klabunde KJ, Sorensen CM. Synthesis of spherical silver nanoparticles by digestive ripening, stabilization with various agents, and their 3-D and 2-D superlattice formation. J Colloid Interface Sci. 2005;284(2):521–526. doi: 10.1016/j.jcis.2004.10.038
- Kuhn LT, Bojesen A, Timmermann L, et al. Structural and magnetic properties of core shell iron iron oxide nanoparticles. J Phys Condens Matter. 2002;14(49):13551–13567. doi: 10.1088/0953-8984/14/49/311
- Jayseelan C, Ramkumar R, Rahman AA, et al. Green synthesis of gold nanoparticles using seed aqueous extract of abelmoschus esculentus and its antifungal actvity. Ind Crops Prod. 2013;45:423–429. doi: 10.1016/j.indcrop.2012.12.019
- Hammani S, Barhoum A, Nagarajan S, et al. Toner waste powder (twp) as a filler for polymer blends (LDPE/HIPS) for enhanced electrical conductivity. Materials. 2019;12(19):3062. doi: 10.3390/ma12193062
- Sudha PN, Sangeetha K, Vijayalakshmi K, et al. Nanomaterials history, classification, unique properties, production and market. In: Hamdy Makhlouf AS, Barhoum A, editors. Emerging applications of nanoparticles and architectural nanostructures: current prospects and future trends. Amsterdam, The Netherlands: Elsevier Inc.; 2018. pp. 341–384.
- Liu JL, Bashir S. Advanced nanomaterials and their applications in renewable energy. Amsterdam, The Netherlands: Elsevier Science; 2015.
- Akpan EI, Shen X, Wetzel B, et al. Design and synthesis of polymer nanocomposites. In: Pielichowski K, Majka TM editors. Polymer composites with functionalized nanoparticles: synthesis, properties, and applications. Amsterdam, The Netherlands: Elsevier; 2018. p. 47–83.
- Ajish JK, Kanagare AB, Ajish Kumar KS, et al. Self-assembled Glycobis(acrylamide)-stabilized gold nanoparticles for fluorescent turn-on sensing of lectin and Escherichia coli. ACS Appl Nano Mater. 2020;3(2):1307–1317. doi: 10.1021/acsanm.9b02127
- Wang Z, Hu T, Liang R, et al. Application of zero-dimensional nanomaterials in biosensing. Front Chem. 2020;8:320. doi: 10.3389/fchem.2020.00320
- Roco MC, Bainbridge WS. Societal implications of nanoscience and nanotechnology: maximizing human benefit. J Nanopart Res. 2005;7(1):1–13. doi: 10.1007/s11051-004-2336-5
- Albrecht MA, Evan CW, Raston CL. Green chemistry and the health implications of nanoparticles. Green Chem. 2006;8(5):417–432. doi: 10.1039/b517131h
- Bhattacharyya D, Singh S, Satnalika N, et al. Nanotechnology, big things from a tiny world: a review. Int J U E-Serv Sci Technol. 2009;2: 229–238.
- Kumar A, Kanagare AB, Banerjee S, et al. Synthesis of cobalt hexacyanoferrate nanoparticles and its hydrogen storage properties. Int J Hydrogen Energy. 2018;43(16):7998–8006. doi: 10.1016/j.ijhydene.2018.03.011
- Kanagare AB, Yadav AR, Katariya AP, et al. Insights into 4,4′-arylmethylene-bis-1 H -pyrazol-5-ols scaffolds: various synthetic routes and their applications. Chem Select. 2023;8(4):e202204088. doi: 10.1002/slct.202204088
- Jadhav PM, Kanagare AB, Dhirbassi AB, et al. Recent advances in nanocatalyzed synthesis of Triazoles and tetrazoles and their biological studies. Boca Raton, Florida: CRC Press; 2022. p. 177–200.
- Katariya AP, Dhas AK, Kanagare AB, et al. Nano-catalyzed synthesis of pyranopyrazole and pyridine scaffolds. Nanopart Green Org Synth. 2023;485–504. doi: 10.1016/B978-0-323-95921-6.00005-6
- Kanagare AB, Pansare DN, Dhas AK, et al. Nanocatalysis: an efficient tool for the synthesis of Triazines and Tetrazines Nanocatalysis. In: Ameta KL, Kant R, editors. Nanocatalysis -Synthesis of Bioactive Heterocycles. Boca Raton, Florida: CRC Press; 2022. p. 127–146.
- Kane AL, Bond DR, Gralnick JA. Electrochemical analysis of Shewanella oneidensis engineered to bind gold electrodes. ACS Synth Biol. 2013;2:93–101. doi: 10.1021/sb300042w
- Lee Y, Loew A, Sun S. Surface- and structure-dependent catalytic activity of Au nanoparticles for oxygen reduction reaction. Chem Mater. 2010;22(3):755–761. doi: 10.1021/cm9013046.
- Marshall F, Chishala BH, Kapungwe E, et al. Contaminated irrigation water and food safety for the urban and peri urban poor: appropriate measures for monitoring and control from the field research in India and Zambia, Inception report DFID Enkar r8160. Brighton: SPRU, University of Sussex; 2007.
- Mishra S, Bharagava RN. Toxic and genotoxic effects of hexavalent chromium in environment and its bioremediation strategies. J Environ Sci Health Part C. 2016;34(1):1–34. doi: 10.1080/10590501.2015.1096883
- Guerra FD, Attia MF, Whitehead DC, et al. Nanotechnology for environmental remediation: materials and applications. Molecules. 2018;23(7):1760. doi: 10.3390/molecules23071760
- Chowdhary P, More N, Raj A, et al. Characterization and identification of bacterial pathogens from treated tannery wastewater. Microbiol Res Int. 2017b;5(3):30–36. doi: 10.30918/MRI.53.17.016
- Nandy T, Kaul SN, Shastry S, et al. Wastewater management in cluster of tanneries in Tamil Nadu through implementation of common effluent treatment plants. J Sci Ind Res. 1999;58:475–516.
- Yadav A, Mishra S, Kaithwas G, et al. 2016a Organic pollutants and pathogenic bacteria in tannery wastewater and their removal strategies. In: Singh J, Singh D, editors Microbes and environmental management. New Delhi: Studium Press (India) Pvt. Ltd; 2016a. p. 101–127.
- Chowdhury M, Mostafa MG, Biswas TK, et al. Treatment of leather industries effluents by filtration and coagulation process. Water Res Ind. 2013;3:11–22. doi: 10.1016/j.wri.2013.05.002
- Kumari V, Yadav A, Haq I, et al. Genotoxicity evaluation of tannery effluent treated with newly isolated hexavalent chromium reducing Bacillus cereus. J Environ Manag. 2016;183:204–211. doi: 10.1016/j.jenvman.2016.08.017
- Yadav A, Raj A, Bharagava RN.Detection and characterization of a multidrug and multimetal resistant enterobacterium Pantoea sp. from tannery wastewater after secondary treatment process. Int J Plant Environ. 2016b;2(2):37–42. doi: 10.18811/ijpen.v2i1-2.6616
- Kumari V, Sharad K, Izharul H, et al. Effect of tannery effluent toxicity on seed germination á-amylase activity and early seeding growth of mung bean (Vigna Radiata) seeds. Int J Lat Res Sci Technol. 2014;3(4):165–170.
- Saha NK, Balakrishnan M, Batra VS. Improving industrial water use: case study for an Indian distillery. Resour Conserv Recycl. 2005;43(2):163–174. doi: 10.1016/j.resconrec.2004.04.016
- CPCB Central Pollution Control Board. Central zonal office Bhopal, a report on assessment of grain based fermentation technology. 2011.
- Bharagava RN, Chandra R. Biodegradation of the major color containing compounds in distillery wastewater by an aerobic bacterial culture and characterization of their metabolites. Biodegradation. 2010a;21(5):703–711. doi: 10.1007/s10532-010-9336-1
- Chowdhary P, Raj A, Bharagava RN. Environmental pollution and health hazards from distillery wastewater and treatment approaches to combat the environmental threats: a review. Chemosphere. 2017c;194:229–246. doi: 10.1016/j.chemosphere.2017.11.163
- Pant D, Adholeya A. Biological approaches for treatment of distillery wastewater: a review. Bioresour Technol. 2007;98(12):2321–2334. doi: 10.1016/j.biortech.2006.09.027
- Meric S, Lofrano G, Belgiorno V. Treatment of reactive dyes and textile finishing wastewater using Fenton’s oxidation for reuse. International journal of environment and pollution. 2005;23(3):248–258. doi: 10.1504/IJEP.2005.006865
- Chen W, Westerhoff P, Leenheer JA, et al. Fluorescence excitation−emission matrix regional integration to quantify spectra for Dissolved organic matter. Environ Sci Technol. 2003;37(24):5701–5710. doi: 10.1021/es034354c
- Mohana S, Acharya BK, Madamwar D. Distillery spent wash: treatment technologies and potential applications. J Hazard Mater. 2009;163(1):12–25. doi: 10.1016/j.jhazmat.2008.06.079
- Mahimraja S, Bolan NS. Problems and prospects of agricultural use of distillery spent wash in India. In: Super soil, 3rd Australian New Zealand soils conference. University of Sydney, Australia; Dec 5–9, 2004.
- Ince BK, Zeynep C, Ince O. Biotransformation of malachite green by Saccharomyces cerevisiae MTCC 463. Yeast. 2011;23(4):315–323. doi: 10.1002/yea.1356
- Yadav BR, Garg A. Treatment of pulp and paper mill effluent using physicochemical processes. IPPTA J. 2011;23:155–160.
- Mani S, Bharagava RN. Exposure to crystal violet, its toxic genotoxic and carcinogenic effects on environment and its degradation and detoxification for environmental safety. Rev Contam Toxicol. 2016;273:71–104.
- Zhou H, Smith DW. Advanced technologies in water and wastewater treatment. J Environ Eng Sci. 2002;1(4):247–264. doi: 10.1139/s02-020
- Chavan RB. Indian textile industry- environmental issue. Indian J Fibre Text Res. 2001;26:11–21.
- Jayathilakan K, Sultan K, Radhakrishnan K, et al. Utilization of by products and waste material from meat, poultry and fish processing industries: a review. J Food Sci Technol. 2012; 49(3):278–293. doi: 10.1007/s13197-011-0290-7
- Helkar PB, Sahoo AK, Patil NJ. Review: food industry by products functional food ingredients department of technology. Int J Waste Resour. 2016;6(3):3 doi: 10.4172/2252-5211.1000248
- Pathak N, Hagare P, Guo W, et al. Australian food processing industry and environmental aspect– a review. International conference on biological civil and engineering. Bali, Indonesia. BCEE; Feb, 3-4, 2015.
- Russ W, Pittroff RM Utilizing waste products from the food production and processing industries. Crit Rev Food Sci Nutr. 2016;44(2):57–62. doi: 10.1080/10408690490263783
- Weiland P, Rozzi A. The start-up operation and monitoring of high-rate anaerobic treatment systems: discussion report. Water Sci Technol. 1991;24(8):257–277, 10.2166/wst.1991.0227
- Ramirez N, Marce RM, Borrull F. Determination of volatile organic compounds in industrial wastewater plant air emission by multi-sorbent adsorption and thermal desorption-gas chromatography-mass spectrometry. Int J Environ Anal Chem. 2011;91(10):911–928. doi: 10.1080/03067310903584073
- WHO World Health Organization. Air quality guidelines for Europe. 2nd. Copenhagen: WHO Regional Publications, 2000. European series no. 29.
- USEPA U.S. Environmental Protection Agency. Office of air quality planning cancer risk from outdoor exposure to air toxics EPA-450/1-90-004a. Triangle Park NC: Office of Air Quality Planning and Standards Research; 1990.
- Lofrano G, Meric S, Zengin GE, et al. Chemical and biological treatment technologies for leather tannery chemicals and wastewater: a review. Sci Total Environ. 2013;461&462:265–281. doi: 10.1016/j.scitotenv.2013.05.004
- Worch E. Adsorption technology in water treatment fundamental processing and modelling. Berlin: Walter de Gruyter& Co. KG; 2012.p. 1–345.
- Kurniawan TA, Babel S. A research study on Cr(VI) removal from contaminated wastewater using low cost adsorbents and commercial activated carbon. In: Second international conference on energy and technology towards clean environment (RCETE). Thailand; VI. 2. Phuket. 2003;2:1110–1117.
- Gillion RJ. Pesticide in US streams and groundwater. Environ Sci Technol. 2007; 41(10):3408–3414. doi: 10.1021/es072531u
- Kanagare AB, Singh K, Bairwa KK, et al. Dithiodiglycolamide impregnated XAD-16 beads for separation and recovery of palladium from acidic waste. J Env Chem Eng. 2016;4(3):3357–3363. doi: 10.1016/j.jece.2016.06.031
- Kanagare AB, Singh K, Kumar M, et al. DTDGA impregnated XAD-16 beads for separation of gold from electronic waste. Ind Eng Chem Res. 2016;55(49):12644–12654. doi: 10.1021/acs.iecr.6b03350
- Romo-Hualde A, Penas FJ, Isasi JR, et al. Extraction of phenols from aqueous solutions by beta-cyclodextrin polymers. Comparison of sorptive capacities with other sorbents. React Funct Polym. 2008;68(1):406–413.
- Saleh TA, Tuzen M, Sarı A. Effective antimony removal from wastewaters using polymer modified sepiolite: isotherm kinetic and thermodynamic analysis. Chem Eng Res Des. 2022; 184: 215–223.
- Saleh TA, Sarı A, Tuzen M. Effective adsorption of antimony (III) from aqueous solutions by polyamide-graphene composite as a novel adsorbent. Chem Eng J. 2017;307: 230–238.
- Pedersen AJ. Characterization and electrolytic treatment of wood combustion fly ash for the removal of cadmium. Biomass Bioenergy. 2003;25(4):447–458 doi: 10.1016/S0961-9534(03)00051-5
- Oztekin E, Atlin S. Wastewater treatment by electro dialysis system and fouling problems. Online J Sci Technol. 2016;6(1):91–99.
- Chang DI, Chook H, Jung, et al. Foulant identification and fouling control with iron oxide adsorption in electro dialysis for the desalination of secondary effluent. Desalination. 2009;236(1–3):152–159.
- Chen GH. Electrochemicals technologies in wastewater treatment. Sep Purif Technol. 2004;38(1):11–41. doi: 10.1016/j.seppur.2003.10.006
- Mohammadi T, Razmi A, Sadrzadeh M. Effect of operating parameters on Pb+2 separation from wastewater using electrodialysis. Desalination. 2004;167:379–385. doi: 10.1016/j.desal.2004.06.150
- Lee HJ, Moon SH, Tsai SP. Effects of pulsed electric field on membrane fouling in electro dialysis of NaCl solution containing humate. Sep Purif Technol. 2002;27(2):89–95. doi: 10.1016/S1383-5866(01)00167-8
- Barakat MA. New trends in removing heavy metals from industrial wastewater. Arab J Chem. 2011;4(4):361–377. doi: 10.1016/j.arabjc.2010.07.019
- Bose P, Bose MA, Kumar S. Critical evaluation of treatment strategies involving adsorption and chelation for wastewater containing copper, zinc, and cyanide. Adv Environ Res. 2002;7(1):179–195. doi: 10.1016/S1093-0191(01)00125-3
- Kanagare AB, Singh K, Kumar M, et al. Synthesis of D2EHPA impregnated polymeric beads for extraction of zinc from zinc-rich waste liquor. Curr Appl Polym Sci. 2017;1 (2):193–206. doi: 10.2174/2452271601666170619125415
- Omelia C. Coagulation and sedimentation in lakes, reservoirs and water treatment plants. Water Sci Technol. 1998;37(2):129. doi: 10.2166/wst.1998.0122
- USEPA U.S. Environmental Protection Agency. Primer for municipal wastewater treatment systems. Document no. EPA832-R-04-001, Washington. 2004.
- Lofrano G, Meriç S, Zengin GE, et al. Chemical and biological treatment technologies for leather tannery chemicals and wastewaters: a review. Sci Total Environ. 2013;461–462:265–281. doi: 10.1016/j.scitotenv.2013.05.004
- Megharaj M, Ramakrishnan B, Venkateswarlu K, et al. Bioremediation approaches for organic pollutants: a critical perspective. Environ Int. 2011;37(8):1362–1375. doi: 10.1016/j.envint.2011.06.003
- Nassef E. Removal of phosphates from industrial wastewater by chemical precipitation. Eng Sci Technol Int J. 2012;2(3):409–413.
- Bianco B, Michelis DI, Veglio F. Fenton treatment of complex industrial wastewater: optimization of process condition by surface response method. J Hazard Mater. 2011; 186(2–3):1733–1738. doi: 10.1016/j.jhazmat.2010.12.054
- Covinch LG, Bengoechea DI, Fenoglio RJ, et al. Advanced oxidation process for wastewater in pulp and paper industry: a review. Am J Environ Eng. 2014;4(3):56–70. doi: 10.5923/j.ajee.20140403.03
- Gogate PR, Pandit AB. A review of imperative technologies for wastewater treatment I: oxidation technologies at ambient conditions. Adv Environ Res. 2004;8(3–4):501–551. doi: 10.1016/S1093-0191(03)00032-7
- Krishnan S, Rawindran H, Sinnathambi CM, et al. Comparison of various advanced oxidation processes used in remediation of industrial wastewater laden with recalcitrant pollutants. 29th Symposium of Malaysian chemical engineers IOP conference. Series: Material Science and Engineering 206. Malaysia; 2016.
- Dixit S, Yadav A, Dwivedi PD, et al. Toxic hazard of leather industry and technologies to combat threat: a review. J Clean Prod. 2015;87:39–49. doi: 10.1016/j.jclepro.2014.10.017
- Soury R, Jabli M, Saleh TA, et al. Degradation of calmagite by dichloride (5, 10, 15, 20tetraphenylporphyrinato) antimony hexachloridoantimonate: [Sb (TPP) Cl2] SbCl6. Inorg Chem Commun. 2019;104:54–60. doi: 10.1016/j.inoche.2019.03.033
- Srinivasam SV, Mary GPS, Kalyanaraman C, et al.Combined advanced oxidation and biological treatment of tannery effluent. Clean Tech Environ Policy. 2012; 14(2):251–256. doi: 10.1007/s10098-011-0393-x
- Rameshraja D, Suresh S. Treatment of tannery wastewater by various oxidation and combined processes. Int J Environ Res. 2011;5(2):349–360.
- Glaze WH, Kang JW. Advanced oxidation process. Description of a kinetic model for the oxidation of hazardous materials in aqueous media with ozone and hydrogen peroxide in a semi batch reactor. Ind Eng Chem Res. 1989;28(11):1573–1580. doi: 10.1021/ie00095a001
- Santos WDL, Ramosa T, Pozyak I, et al. Remediation of lignin and its derivatives from pulp and paper industry wastewater by the combination of chemical precipitation and ozonation. J Hazard Mater. 2009;169(1–3):428–434. doi: 10.1016/j.jhazmat.2009.03.152
- Ananpattarachai J, Kumket P. Chromium (VI) removal using nano-TiO2/chitosan film in photocatalytic system. Int J Environ Waste Manag. 2015;16(1):55–70. doi: 10.1504/IJEWM.2015.070481
- Skubal LR, Meshkov NK, Rajh T, et al. Cadmium removal from water using thiolactic acid-modified titanium dioxide nanoparticles. Photochem Photobiol A Chem. 2002;148(1–3):393–397. doi: 10.1016/S1010-6030(02)00069-2
- Van der Bruggen B, Vandecasteele C, Gastel TV, et al. A review of pressure- driven membrane processes in wastewater treatment and drinking water production. Environ Prog. 2003;22(1):46–56.
- Zhou H, Smith DW. Advanced technologies in water and wastewater treatment. J Environ Sci. 2002;1(4):247–264. doi: 10.1139/s02-020
- Koyuncu I. Reactive dye removal in dye/salt mixture by nanofiltration membranes containing vinyl sulphone dyes effects of food concentration and cross flow velocity. Desalination. 2002;143(3):243–253. doi: 10.1016/S0011-9164(02)00263-1
- Frank MJW, Westerink JB, Schokker A. Recycling of industrial waste water by using a two step nanofiltration process for the removal of colour. Desalination. 2002;145(1–3):69–74. doi: 10.1016/S0011-9164(02)00388-0
- Rao MA, Scelza R, Acevedo F, et al. Enzymes as useful tools for environmental purposes. Chemosphere. 2014;107:145–162. doi: 10.1016/j.chemosphere.2013.12.059
- Sharma L, Kahandal A, Kanagare A, et al. The multifaceted nature of plant acid phosphatases: purification, biochemical features, and applications. J Enzyme Inhib Med Chem. 2023;38(1):2282379. doi: 10.1080/14756366.2023.2282379
- Nicell JA. Enzymatic treatment of waters and wastes. In: Tarr MA editor. Chemical degradation methods for wastes and pollutants: environmental and industrial applications. Boca Raton: CRC Press; 2003. p. 384–428.
- Adam W, Lazarus M, Saha-Mollera C, et al. Biotransformation with peroxidase. Adv Biochem Eng Biotechnol. 1999;63:73–107.
- Nelson C, Cox M. Principles of biochemistry. 4th edn. New York: W. H. Freeman; 2004 p. 47–50.
- Chandra R, Chowdhary P. Properties of bacterial laccases and their application in bioremediation of industrial wastes. Environ Sci Process Impacts. 2015;17(2):326–342. doi: 10.1039/C4EM00627E
- Guenther T, Sack U, Hofrichter M, et al. Oxidation PAH and PAG derivatives by fungal and plant oxidoreductases. J Basic Microbiol. 1998;38(2):113–122. doi: 10.1002/(SICI)1521-4028(199805)38:2<113:AID-JOBM113>3.0.CO;2-D
- Toumela M, Hatakka A. Oxidative fungal enzymes for bioremediation. In: Agathos A, MooYoung M editors. Comprehensive biotechnology: environmental biotechnology and safety, Vol. 2, Amsterdam: Elsevier; 2011. p. 183–196.
- Park JW, Park BK, Kim JE. Remediation of soil contaminated with 2,4-dicholrophenol by treatment of minced shepherd’s purse roots. Arch Environ Contam Toxicol. 2006;50(2):191–195. doi: 10.1007/s00244-004-0119-8
- Biswas MM. Removal of reactive azo dyes from water by Feo reduction followed by peroxidase catalysed polymerization. Canada: University of Windsor; 2004.
- Barnhardt R. Cytochromes P450 as versatile biocatalyst. J Biotechnol. 2006124(1):128–145. doi: 10.1016/j.jbiotec.2006.01.026
- Murthy PS, Naidu MM. Sustainable management of coffee industry by-products and value addition – a review. Resour Conserv Recycl. 2012;66:45–58. doi: 10.1016/j.resconrec.2012.06.005
- Kapoor M, Rajagopal R. Enzymatic bioremediation of organophosphorus insecticides by recombinant organophosphorus hydrolase. Int Biodeter Biodegr. 2011;65(6):896–901. doi: 10.1016/j.ibiod.2010.12.017
- Porro SC, Martin S, Mellado, et al. Diversity of moderately halophilic bacteria producing extracellular hydrolytic enzymes. J Appl Microbiol. 2003;94(2):295–300.
- Okonko IO. Shittu OB bioremediation of wastewater and municipal water treatment using latex exudate from calotropis procera (sodium apple). Elec J Env Agricult Food Chem. 2007;6(3):1890–1904.
- Diez MC. Biological aspects involved in the degradation of organic pollutants. J Soil Sci Plant Nutr. 2010;10(3):244–267. doi: 10.4067/S0718-95162010000100004
- Chaney RL, Malik M, Li YM, et al. Phytoremediation of soil metals. Curr Opin Biotechnol. 1997;8(3):279–284. doi: 10.1016/S0958-1669(97)80004-3
- Kabra AN, Khandare RV, Govindwar SP. Development of a bioreactor for remediation of textile effluent and dye mixture: a plant–bacterial synergistic strategy. Water Res. 2012;47(3):1035–1048. doi: 10.1016/j.watres.2012.11.007
- Oliveira H. Chromium as an environmental pollutant: insights on induced plant toxicity. J Bot. 2012;2012:1–8. Article ID 375843. doi: 10.1155/2012/375843
- Vyzmazal J. Plants used in constructed wetlands with horizontal subsurface flow: a review. Hydrobiologia. 2011;674(1):133–156. doi: 10.1007/s10750-011-0738-9
- Bai Y, Liang J, Liu R, et al. Metagenomic analysis reveals microbial diversity and function in the rhizosphere soil of a constructed wetland. Environ Technol. 2014;35(20):2521–2527. doi: 10.1080/09593330.2014.911361
- Rai PK. Heavy-metal pollution in aquatic ecosystem and its phytoremediation using wetland plants: an eco-sustainable approach. Int J Phyto. 2008;10(2):133–160. doi: 10.1080/15226510801913918
- Rathod PB, Chappa S, Ajishkumar KS, et al. Cadmium(ii)-loaded Fe3O4@MPTS nanoparticles: preparation and application as catalyst for c-n coupling reactions. ChemistrySelect. 2019;4(40):11796–11800. doi: 10.1002/slct.201902432
- Saravanan M, Barik SK, Mubarak Ali D, et al. Synthesis of silver nanoparticles from Bacillus brevis (NCIM 2533) and their antibacterial activity against pathogenic bacteria. Microb Pathog. 2018;116:221–226. doi: 10.1016/j.micpath.2018.01.038
- Klaus T, Joerger R, Olsson E, et al. Silver-based crystalline nanoparticles, microbially fabricated. Proc Natl Acad Sci, USA. 1999;96(24):13611–13614. doi: 10.1073/pnas.96.24.13611
- Pugazhenthiran N, Anandan S, Kathiravan G, et al. Microbial synthesis of silver nanoparticles by bacillus sp. J Nanopart Res. 2009;11(7):1811. doi: 10.1007/s11051-009-9621-2
- Husseiny M, El-Aziz MA, Badr Y, et al. Biosynthesis of gold nanoparticles using Pseudomonas aeruginosa. Spectrochim. Acta A Mol Biomol Spectrosc. 2007;67(3–4):1003–1006. doi: 10.1016/j.saa.2006.09.028
- He S, Guo Z, Zhang Y, et al. Biosynthesis of gold nanoparticles using the bacteria Rhodopseudomonas capsulata. Mater Lett. 2007;61(18):3984–3987. doi: 10.1016/j.matlet.2007.01.018
- Dhandapani P, Siddarth AS, Kamalasekaran S, et al. Bio-approach: Ureolytic bacteria mediated synthesis of ZnO nanocrystals on cotton fabric and evaluation of their antibacterial properties. Carbohydr Polym. 2014;103:448–455. doi: 10.1016/j.carbpol.2013.12.074
- Selvarajan E, Mohanasrinivasan V. Biosynthesis and characterization of ZnO nanoparticles using lactobacillus plantarum VITES07. Mater Lett. 2013;112:180–182. doi: 10.1016/j.matlet.2013.09.020
- Jayaseelan C, Rahuman AA, Kirthi AV, et al. Novel microbial route to synthesize ZnO nanoparticles using Aeromonas hydrophila and their activity against pathogenic bacteria and fungi. Spectrochim Acta A Mol Biomol Spectrosc. 2012;90:78–84. doi: 10.1016/j.saa.2012.01.006
- Rad M, Taran M, Alavi M. Effect of incubation time, CuSO4 and glucose concentrations on biosynthesis of copper oxide (CuO) nanoparticles with rectangular shape and antibacterial activity: Taguchi method approach. Nano Biomed Eng. 2018;10(1):25–33. doi: 10.5101/nbe.v10i1.p25-33
- Fatemi M, Mollania N, Momeni-Moghaddam M, et al. Extracellular biosynthesis of magnetic iron oxide nanoparticles by Bacillus cereus strain HMH1: characterization and in vitro cytotoxicity analysis on MCF-7 and 3T3 cell lines. J Biotechnol. 2018;270:1–11. doi: 10.1016/j.jbiotec.2018.01.021
- Abdel Rahim K, Mahmoud SY, Ali AM, et al. Extracellular biosynthesis of silver nanoparticles using Rhizopus stolonifer. Saudi J Biol Sci. 2017;24(1):208–216. doi: 10.1016/j.sjbs.2016.02.025
- Jalal M, Ansari M, Alzohairy M, et al. Biosynthesis of silver nanoparticles from Oropharyngeal Candida glabrata isolates and their antimicrobial activity against clinical strains of bacteria and fungi. Nanomaterials. 2018;8(8):586. doi: 10.3390/nano8080586
- Kalpana V, Kataru BAS, Sravani N, et al. Biosynthesis of zinc oxide nanoparticles using culture filtrates of aspergillus niger: antimicrobial textiles and dye degradation studies. OpenNano. 2018;3:48–55. doi: 10.1016/j.onano.2018.06.001
- Vijayanandan AS, Balakrishnan RM. Biosynthesis of cobalt oxide nanoparticles using endophytic fungus Aspergillus nidulans. J Environ Manag. 2018;218:442–450. doi: 10.1016/j.jenvman.2018.04.032
- Nair B, Pradeep T Coalescence of nanoclusters and formation of submicron crystallites assisted by lactobacillus strains. Cryst Growth Des. 2002;2(4):293–298. doi: 10.1021/cg0255164
- Molnár Z, Bódai V, Szakacs G, et al. Green synthesis of gold nanoparticles by thermophilic filamentous fungi. Sci Rep. 2018;8(1):3943. doi: 10.1038/s41598-018-22112-3
- Zhang X, He X, Wang K, et al. Different active biomolecules involved in biosynthesis of gold nanoparticles by three fungus species. J Biomed Nanotechnol. 2011;7(2): 245–254. doi: 10.1166/jbn.2011.1285
- Vahabi K, Ali MG, Sedighe K. Biosynthesis of silver nanoparticles by fungus Trichoderma Reesei (A route for large scale production of AgNPs; Insciences J. 2011;1(1): 65–79. doi: 10.5640/insc.010165
- Feng L, Huang Z, Ren J, et al. Toward site-specific, homogeneous and highly stable fluorescent silver nanoclusters fabrication on triplex DNA scaffolds. Nucleic Acids Res. 2012;40(16):22. doi: 10.1093/nar/gks387
- Rotaru A, Dutta S, Jentzsch E, et al. Selective dsDNA-templated formation of copper nanoparticles in solution. Angew Chem. 2010;49(33):5665–5667. doi: 10.1002/anie.200907256
- Su Y-T, Lan G-Y, Chen W-Y, et al. Site-specific DNA-Programmed growth of fluorescent and functional silver nanoclusters. Chem – Eur J. 2011;17(13):3774–3780.
- Su Y-T, Lan G-Y, Chen W-Y, et al. Detection of copper ions through recovery of the fluorescence of DNA-Templated copper/silver nanoclusters in the presence of mercaptopropionic AcidAnal. Anal Chem. 2010;82(20):8566–8572. doi: 10.1021/ac101659d
- Tolaymat TM, El Badawy AM, Genaidy A, et al. An evidence-based environmental perspective of manufactured silver nanoparticle in syntheses and applications: a systematic review and critical appraisal of peer-reviewed scientific papers. Sci Total Environ. 2010;408(5):999–1006.
- Hebeish A, Shaheen TI, Mehrez E. Solid state synthesis of starch-capped silver nanoparticles int. J Biol Macromol. 2016;87:70–76. doi: 10.1016/j.ijbiomac.2016.02.046
- Shankar SS, Ahmad A, Pasrichaa R, et al. Bioreduction of chloroaurate ions by geranium leaves and its endophytic fungus yields gold nanoparticles of different shapes. J Mater Chem. 2003;13(7):1822–1826. doi: 10.1039/b303808b
- Shankar SS, Rai A, Ahmad A, et al. Controlling the optical properties of lemongrass extract synthesized gold nanotriangles and potential application in infrared-absorbing optical coatings. Chem Mater. 2005;17(3):566–572. doi: 10.1021/cm048292g
- Huang J, Li Q, Sun D, et al. Biosynthesis of silver and gold nanoparticles by novel sundried Cinnamomum camphora leaf. Nanotechnology. 2007;18(10):105104–105114. doi: 10.1088/0957-4484/18/10/105104
- Shankar SS, Rai A, Ahmad A, et al. Rapid synthesis of Au, Ag, and bimetallic Au core–ag shell nanoparticles using neem (Azadirachta indica) leaf broth. J Colloid Interf Sci. 2004;275(2):496–502. doi: 10.1016/j.jcis.2004.03.003
- Ankamwar B, Chaudhary M, Sastry M. Gold nanotriangles biologically synthesized using tamarind leaf extract and potential application in vapor sensing. Synth React Inorg Metal-Org Nano-Metal Chem. 2005;35(1):19–26. doi: 10.1081/SIM-200047527
- Ankamwar B, Damle C, Ahmad A, et al. Biosynthesis of gold and silver nanoparticles using Emblica officinalis fruit extract, their phase transfer and transmetallation in an organic solution. J Nanosci Nanotechnol. 2005;5(10):1665–1671. doi: 10.1166/jnn.2005.184
- Armendariz V, Gardea-Torresdey JL, Jose-Yacaman M, et al. Gold nanoparticles formation by oat and wheat biomasses. Proceedings – Waste Research Technology Conference. Kansas City, Missouri; 2002 July 30–Aug 1.
- Gardea-Torresdey JL, Gomez E, Peralta-Videa JR, et al. Alfalfa sprouts: a natural source for the synthesis of silver nanoparticles. Langmuir. 2003;19(4):1357–1361. doi: 10.1021/la020835i
- Lopeza ML, Parsonsb JG, Videab JRP, et al. An XAS study of the binding and reduction of Au(III) by hop biomass. Microchem J. 2005;81(1):50–56. doi: 10.1016/j.microc.2005.01.011
- Ghule K, Ghule AV, Liu JY, et al. Microscale size triangular gold prisms synthesized using Bengal gram beans (Cicer arietinum L.) extract and HAuCl4·3H2O: a green biogenic approach. J Nanosci Nanotechnol. 2006;6(12):3746–3751. doi: 10.1166/jnn.2006.608
- Gardea-Torresdey JL, Parsons JG, Gomez E, et al. Formation and growth of Au nanoparticles inside live alfalfa plants. Am Chem Soc. 2002;2:397–401.
- Parveen K, Banse V, Ledwani L. Green synthesis of nanoparticles: their advantages and disadvantages. 2016. doi: 10.1063/1.4945168
- Kolya H, Maiti P, Pandey A, et al. Green synthesis of silver nanoparticles with antimicrobial and azo dye (Congo red) degradation properties using amaranthus gangeticus Linn leaf extract. J Anal Sci Technol. 2015;6(1):33. doi: 10.1186/s40543-015-0074-1
- Roy P, Das B, Mohanty A, et al. Green synthesis of silver nanoparticles using Azadirachta indica leaf extract and its antimicrobial study. Appl Nanosci. 2017;7(8):843–850. doi: 10.1007/s13204-017-0621-8
- Kahandal A, Chaudhary S, Methe S, et al. Galactomannan polysaccharide as a biotemplate for the synthesis of zinc oxide nanoparticles with photocatalytic, antimicrobial and anticancer applications. Int j biol macromol. 2023;253:126787. doi: 10.1016/j.ijbiomac.2023.126787
- Saha J, Begum A, Mukherjee A, et al. A novel green synthesis of silver nanoparticles and their catalytic action in reduction of methylene blue dye. Sustain Environ Res. 2017;27(5):245–250. doi: 10.1016/j.serj.2017.04.003
- Uddin I, Ahmad K, Khan AA, et al. Synthesis of silver nanoparticles using matricaria recutita (Babunah) plant extract and its study as mercury ions sensor. Sens Biosens Res. 2017;16:62–67. doi: 10.1016/j.sbsr.2017.11.005
- Vanaja M, Paulkumar K, Baburaja M, et al. Degradation of methylene blue using biologically synthesized silver nanoparticles. Bioinorgan Chem Appl. 2014;1–9. Article ID 742346. doi: 10.1155/2014/742346
- Patel R, Mehta M. Green synthesis of silver nanoparticles by using fruit and vegetable waste: a review. Int J Eng Res Appl. 2019;9:78–85.
- Khedkar MA, Nimbalkar PR, Chavan PV, et al. Cauliflower waste utilization for sustainable biobutanol production: revelation of drying kinetics and bioprocess development. Bioprocess Biosyst Eng. 2017;40(10):1493–1506. doi: 10.1007/s00449-017-1806-y
- Cartea ME, Francisco M, Soengas P, et al. 2011Phenolic compounds in Brassica vegetables. Molecules. 16(1):251–280 doi: 10.3390/molecules16010251
- Tamileswari R, Nisha MH, Jesurani SS. Analysis of antimicrobial activity silver nanoparticle from the Brassicaceae family vegetables. Int J Eng Sci Res Technol 2015;4:804–811.
- Singh J, Mehta A, Rawat M, et al. Green synthesis of silver nanoparticles using sun dried tulsi leaves and its catalytic application for 4-nitrophenol reduction. J Environ Chem Eng. 2018;6(1):1468–1474. doi: 10.1016/j.jece.2018.01.054
- Ranjitham M, Suja R, Caroling G, et al. In vitro evaluation of antioxidant, antimicrobial, anticancer activities and characterisation of Brassica oleracea. Var. Bortrytis. L synthesized silver nanoparticles. Int J Pharm Pharm Sci. 2013;5:239–251.
- Joseph S, Mathew B. Microwave assisted biosynthesis of silver nanoparticles using the rhizome extract of Alpinia galanga and evaluation of their catalytic and antimicrobial activities. J Nanoparticles. 2014;2014:1–9. doi: 10.1155/2014/967802
- Dhand V, Soumya L, Bharadwaj S, et al. Green synthesis of silver nanoparticles using coffea arabica seed extract and its antibacterial activity. Mater Sci Eng C Mater Biol Appl. 2016;58:36–43. doi: 10.1016/j.msec.2015.08.018
- Mukunthan KS, Elumalai EK, Patel TN, et al. Catharanthus roseus: a natural source for the synthesis of silver nanoparticles. Asian Pacific J Trop Biomed. 2011;1(4):270–274. doi: 10.1016/S2221-1691(11)60041-5
- Birla SS, Tiwari VV, Gade AK, et al. Fabrication of silver nanoparticles by Phoma glomerata and its combined effect against Escherichia coli, Pseudomonas aeruginosa and Staphylococcus aureus. Lett Appl Microbiol. 2009;48:173–179. doi: 10.1111/j.1472-765X.2008.02510.x
- GnanaJobitha GD, Annadurai G, Kannan C. Green synthesis of silver nanoparticle using Elettaria cardamomom and assessment of its antimicrobial activity. Int J Pharma Sci Res. 2012; 3:323–330
- Zhiqiang W, Cheng F, Mallavarapu M. Characterization of Iron−Polyphenol nanoparticles synthesized by three plant extracts and their Fenton oxidation of Azo Dye. ACS Sustain Chem Eng. 2014;2(4): 1022–1025 doi: 10.1021/sc500021n
- Dodoo-Arhin D, Asiedu T, Agyei-Tuffour B, et al. Photocatalytic degradation of Rhodamine dyes using zinc oxide Nanoparticles. Mater Today Proc. 2020;38:809–815. doi: 10.1016/j.matpr.2020.04.597
- Mariselvama R, Ranjitsingh AJA, Thamaraiselvi C, et al. Degradation of AZO dye using plants-based silver nanoparticles through ultraviolet radiation. J King Saud Univ Sci. 2019;31(4):1363–1365. doi: 10.1016/j.jksus.2019.07.001
- Murugadoss G, Dinesh Kumar D, Rajesh Kumar M, et al. Silver decorated CeO2 nanoparticles for rapid photocatalytic degradation of textile rose bengal dye. Sci Rep. 2021;11(1):1080. doi: 10.1038/s41598-020-79993-6
- Muhammad ID, Maria T, Zaib H, et al. Single step green synthesis of nickel and nickel oxide nanoparticles from hordeum vulgare for photocatalytic degradation of methylene blue dye. Inorg Nano-Metal Chem. 2020;50(4)292–297. doi: 10.1080/24701556.2019.1711401
- Anshu B, Satyesh RA, Gunture K, et al. Sunlight-induced photocatalytic degradation of pollutant dye by highly fluorescent red-emitting Mg-N-embedded carbon dots. ACS Sustainable Chem Eng. 2018;6:1–26.
- Ijaz F, Shahid S, Khan SA, et al. Green synthesis of copper oxide nanoparticles using abutilon indicum leaf extract: antimicrobial, antioxidant and photocatalytic dye degradation activitie. Trop J Pharm Res. 2017;16(4):743–753. doi: 10.4314/tjpr.v16i4.2
- Norzahir S, Mohd AR, Muhammad HHM, et al. A novel approach of in-situ electrobiosynthesis of metal oxide nanoparticles using crude plant extract as main medium for supporting electrolyte. Mater Today Proc. 2019;19:1441–1445. doi: 10.1016/j.matpr.2019.11.166
- Abdolhossien M, Seyedeh RM, Mina S, et al. Using biebersteinia multifida aqueous extract, and the photocatalytic activity of synthesized silver nanoparticles. Orient J Chem. 2018;34(3):1513–1517. doi: 10.13005/ojc/340342
- Swati R, Arpita R, Navneeta B. Degradation of dyes using biologically synthesized silver and copper nanoparticles. Environ Nanotechnol Monit Manage. 2020;13:100278. doi: 10.1016/j.enmm.2019.100278
- Ganesan S, Ganesh Babu I, Mahendran D, et al. Green engineering of titanium dioxide nanoparticles using Ageratina altissima, King L & robines HE. Medicinal plant aqueous leaf extracts for enhanced photocatalytic activity. Ann Phytomed. 2017;5(2):69–75. doi: 10.21276/ap.2016.5.2.8
- Shakeel AK, Sammia S, Basma S, et al.Green synthesis of MnO nanoparticles using abutilon indicum leaf extract for biological, photocatalytic and adsorption activities. Biomolecules. 2020;10(5):785. doi: 10.3390/biom10050785
- Suresh CM, Anita D, Chanda KG, et al. Green synthesis of copper nanoparticles using Celastrus paniculatus Willd. leaf extract and their photocatalytic and antifungal properties. Biotechnol Reports. 2020;27:e00518. doi: 10.1016/j.btre.2020.e00518
- Kishore C, Dianxue C, Diaa EF, et al. Green synthesis, characterization and photocatalytic application of silver nanoparticles synthesized by various plant extracts. Arab J Chem. 2020;9:1878–5352.
- Adeel A, Muhammad U, Qian-Ying L, et al. Plant mediated synthesis of copper nanoparticles by using camelia sinensis leaves extract and their applications in dye degradation. Ferroelectrics. 2019;549(1) 61–69. doi: 10.1080/00150193.2019.1592544
- Luminita D, Bianca M. Green synthesis of biogenic silver nanoparticles for efficient catalytic removal of harmful organic dyes. Nanomaterials. 2020;10(2):202. doi: 10.3390/nano10020202
- Zahra S, Mohammad S, Mohammad SA, et al. 3D printed antimicrobial PLA constructs functionalised with zinc-coated halloysite nanotubes-Ag-chitosan oligosaccharide lactate. Mater Technol. 2020;37(1):28–35. doi: 10.1080/10667857.2020.1806188
- Abdulmohsen A, Maqsood AM, Zaheer K, et al. Biofabrication of Fe nanoparticles in aqueous extract of hibiscus sabdariffa with enhanced photocatalytic activities. Royal Soc Chem Adv. 2017;7(40):25149–25159. doi: 10.1039/C7RA01251A
- Shahwan T, Sirriah SA, Nairat M, et al. Green synthesis of iron nanoparticles and their application as a Fenton-like catalyst for the degradation of aqueous cationic and anionic dyes. Chem Eng J. 2011;172(1):258–266. doi: 10.1016/j.cej.2011.05.103
- Nadagouda MN, Castle AB, Murdock RC, et al. In vitro biocompatibility of nanoscale zerovalent iron particles (NZVI) synthesized using tea polyphenols. Green Chem. 2010;12(1):114–122. doi: 10.1039/B921203P
- Mishra P, Ray S, Sinha S, et al. Facile bio-synthesis of gold nanoparticles by using extract of hibiscus sabdariffa and evaluation of its cytotoxicity against U87 glioblastoma cells under hyperglycemic condition. Biochem Eng J. 2016;105:264–272.
- Lee SY, Kang D, Jeong S, et al. Photocatalytic degradation of rhodamine B dye by TiO2 and gold nanoparticles supported on a floating porous polydimethylsiloxane sponge under ultraviolet and visible light irradiation. ACS Omega. 2020; 5(8):4233–4241. doi: 10.1021/acsomega.9b04127
- Singh RK, Behera SS, Singh KR, et al. Biosynthesized gold nanoparticles as photocatalysts for selective degradation of cationic dye and their antimicrobial activity. J Photochem Amp Photobiol A Chem. 2020;400:112704. doi: 10.1016/j.jphotochem.2020.112704
- Shahana B, Aarti K, Raja S. Facile synthesis of magnetic iron oxide nanoparticles using inedible cynometra ramiflora fruit extract waste and their photocatalytic degradation of methylene blue dye. Mater Res Bull. 2017;8:040.
- Ebrahim SM, Mina S, Ali R, et al. Plant-mediated synthesis of zinc oxide and copper oxide nanoparticles by using ferulago angulata (schlecht) boiss extract and comparison of their photocatalytic degradation of Rhodamine B (RhB) under visible light irradiation. J Mater Sci Mater Electron. 2017;29 (2):1333–1340. doi: 10.1007/s10854-017-8039-3
- Rasheed T, Bilal M, Li C, et al. Catalytic potential of bio-synthesized silver nanoparticles using Convolvulus arvensis extract for the degradation of environmental pollutants. J Photochem Photobiol. 2018;181:44–52. doi: 10.1016/j.jphotobiol.2018.02.024
- Tayyaba S, Maria Z, Tauheeda R, et al. Synthesis of eco-friendly cobalt nanoparticles using celosia argentea plant extract and their efficacy studies as antioxidant, antibacterial, hemolytic and catalytical agent. Arab J Sci Eng. 2019;44(7):6435–6444. 10.1007/s13369-019-03937-0
- Singh J, Kumar V, Jolly SS, et al. Biogenic synthesis of silver nanoparticles and its photocatalytic applications for removal of organic pollutants in water. J Ind Eng Chem. 2019;80:247–257. doi: 10.1016/j.jiec.2019.08.002
- Seerangaraj V, Selvam S, Mythili S, et al. Synthesis of eco-friendly copper oxide nanoparticles for fabrication over textile fabrics: characterization of antibacterial activity and dye degradation potential. J Photochem Photobiol, B. 2019;191:143–149. doi: 10.1016/j.jphotobiol.2018.12.026
- Lalitha K, Jong CA, Esrat JR, et al. Synthesis of panos extract mediated ZnO nano-flowers as photocatalyst for industrial dye degradation by UV illumination. J Photochem Photobiol B Biol. 2019;199:111588. doi: 10.1016/j.jphotobiol.2019.111588
- Khalida N, Muhammad ZUR, Awais A, et al. Plant extract induced biogenic preparation of silver nanoparticles and their potential as catalyst for degradation of toxic dyes. Coatings. 2020;10(12):1235. doi: 10.3390/coatings10121235
- Ismat B, Nosheen N, Sadia A, et al. Green synthesis of iron oxide nanoparticles using pomegranate seeds extract and photocatalytic activity evaluation for the degradation of textile dye. 2019;8 (6):6115–6124 J Mater Res Technol 10.1016/j.jmrt.2019.10.006
- Volesky B. In removal and recovery of heavy metals by Biosorption: Boca Raton, FL: CRC Press, 1990 Vol. 7: p. 7–43.
- Reimann C, Banks D. Settings action levels for drinking water: are we protecting our health or our economy. Sci Total Environ. 2004;332(1–3):13–21. doi: 10.1016/j.scitotenv.2004.04.007
- Jain CK, Ali I. Arsenic: occurrence, toxicity and speciation techniques water res. Water Res. 2000;34(17):4304–4312. doi: 10.1016/S0043-1354(00)00182-2
- Skerfving S, Gerhardsson L, Schütz A, et al. Lead – biological monitoring of exposure and effects.J Trace Elem Exp Med 1998;11(2–3):289–301. doi: 10.1002/(SICI)1520-670X(1998)11:2/3<289:AID-JTRA17>3.0.CO;2-N
- Soylak M, Elci L, Akkaya Y, et al. On-line preconcentration system for lead determination in water and sediment samples by flow injection-flame atomic absorption spectrometry Anal Lett. 2002;35(3):487–499. doi: 10.1081/AL-120002682
- Gong Z, Chan HT, Chen Q, et al. Application of nanotechnology in analysis and removal of heavy metals in food and water resources. Nanomater (Basel). 2021;11(7): 1792.
- Rathod PB, Ajish Kumar KS, Athawale Anjali A, et al. Cadmium(ii)-loaded Fe3O4@MPTS Nanoparticles: Preparation and application as catalyst for C-N coupling reactions. ChemistrySelect. 2019;4(40):11796–11800. doi: 10.1002/slct.201902432
- Naaz S, Pandey SN. Effects of industrial waste water on heavy metal accumulation, growth and biochemical responses of lettuce (Lactuca sativa L.). J Environ Biol Growth. 2010;31: 273–276.
- Singh A, Rajeshkumar S, Madhoolika A, et al. Risk management of heavy metal toxicity through contaminated vegetables from wastewater irrigated area of Varanasi, India. Trop Ecol. 2010;51:375–387.
- Brynhildsen L, Rosswall T. Effects of metals on the microbial mineralization of organic acids. water, Air Soil Pollut. 1997;94(1–2):45–57. doi: 10.1007/BF02407092
- Sterritt RM, Lester JN. Interactions of heavy metals with bacteria. Sci Tot Environ. 1980;14(1):5–17. doi: 10.1016/0048-9697(80)90122-9
- Cu NX (2015) The effects of heavy metals, phosphate, lime and sawdust on plant growth and heavy metal accumulation by lettuce. ARPN J Agri Biol Sci. 10:241–246.
- Aydinalp C, Marinova S. The effects of heavy metals on seed germination and plant growth on alfalfa plant (Medicago sativa). Bulg J Agri Sci. 2009;15: 347–350.
- Nazar R, Iqbal N, Masood A, et al. Cadmium toxicity in plants and role of mineral nutrients in its alleviation. Amer J Plant Sci. 2012;3(10):1476–1489. doi: 10.4236/ajps.2012.310178
- Mahmood T, Islam KR, Muhammad S. Toxic effects of heavy metals on growth and tolerance of cereal crops. Pak J Bot. 2007;39:451–462.
- Rout GR, Das P.Effect of metal toxicity on plant growth and metabolism: I. Zinc Agronomie. 2003;23(1):3–11. doi: 10.1051/agro:2002073
- Fayigaa AO, Maa LQ, Caoa X, et al. Effects of heavy metals on growth and arsenic accumulation in the arsenic hyper accumulator Pteris vittata L. Environ Pollut. 2004;132(2):289–296. doi: 10.1016/j.envpol.2004.04.020
- Coppola S, Dumontet S, Portonio M, et al. Effect of cadmium bearing sewage sludge on crop plants and microorganisms in two different soils. Agric Ecosyst Environ. 1988;20(3):181–194. doi: 10.1016/0167-8809(88)90110-7
- Baccouch S, Chaovi A, El Ferjani E. Nickel toxicity: effects on growth and metabolism of maize. J Plant Nutr. 1998;21(3):577–588. doi: 10.1080/01904169809365425
- Qdais HA, Moussa H. Removal of heavy metals from wastewater by membrane processes: a comparative study. Desalination. 2004;164(2):105–110. doi: 10.1016/S0011-9164(04)00169-9
- Doble Amin MT, Alazba AA, Manzoor U. A review of removal of pollutants from water/wastewater using different types of nanomaterials. Advances In Materials Science And Engineering. 2014;2014:1–24. doi: 10.1155/2014/825910
- Ganzagh MAA, Yousefpour M, Taherian Z. The removal of mercury (II) from water by Ag supported on nanomesoporous silica. J Biol Chem. 2016;9(4): 127–142. doi: 10.1007/s12154-016-0157-5
- Hashem Stietiya M, Wang JJ. Zinc and cadmium adsorption to aluminum oxide nanoparticles affected by naturally occurring ligands. J Environ Quality. 2016;43(2):498–506. doi: 10.2134/jeq2013.07.0263
- Wu N, Wei H, Zhang L. Efficient removal of heavy metal ions with biopolymer template synthesized mesoporous titania beads of hundreds of micrometers size. Environ Sci Technol. 2011;46(1):419–425. doi: 10.1021/es202043u
- Kadirvelu K, Thamaraiselvi K, Namasivayam C. Removal of heavy metals from industrial wastewaters by adsorption onto activated carbon prepared from an agricultural solid waste. Biores Technol. 2001;76(1):63–65. doi: 10.1016/S0960-8524(00)00072-9
- Kobya M, Demirbas E, Senturk E, et al. Adsorption of heavy metal ions from aqueous solutions by activated carbon prepared from apricot stone. Biores Technol. 2005;96(13):1518–1521. doi: 10.1016/j.biortech.2004.12.005
- Lin S-H, Juang R-S. Heavy metal removal from water by sorption using surfactant-modified montmorillonite. J Hazard Mater. 2002;92(3):315–326. doi: 10.1016/S0304-3894(02)00026-2
- Abollino O, Aceto M, Malandrino M, et al. Adsorption of heavy metals on na-montmorillonite. Effect of pH and organic substances. Water Res. 2003;37(7):1619–1627. doi: 10.1016/S0043-1354(02)00524-9
- Šćiban M, Radetić B, Kevrešan Ž, et al. Adsorption of heavy metals from electroplating wastewater by wood sawdust. Biores Technol. 2007;98(2):402–409. doi: 10.1016/j.biortech.2005.12.014
- Munaf E, Zein R. The use of rice husk for removal of toxic metals from waste water. Environ Technol. 1997;18(3):359–362. doi: 10.1080/09593331808616549
- Srinivasan K, Balasubramanian N, Ramakrishna TV. Studies on chromium removal by rice husk carbon. Indian J Environ Health. 1988;30: 376–387.
- Suemitsu R, Uenishi R, Akashi I, et al. The use of dyestuff-treated rice hulls for removal of heavy metals from waste water. J Appl Polym Sci. 1986;31(1):75–83. doi: 10.1002/app.1986.070310108
- Tan WT, Ooi ST, Lee CK. Removal of chromium (VI) from solution by coconut husk and palm pressed fibres. Environ Technol. 1993;14(3):277–282. doi: 10.1080/09593339309385290
- Mohan D, Singh KP. Single- and multi-component adsorption of cadmium and zinc using activated carbon derived from bagasse – an agricultural waste. Water Res. 2002;36(9):2304–2318. doi: 10.1016/S0043-1354(01)00447-X
- Ayub S, Ali SI, Khan NA, et al. Treatment of wastewater by agricultural waste. Environ Prot Control J. 1998;2:5–8.
- Lesmana SO, Febriana N, Soetaredjo FE, et al. Studies on potential applications of biomass for the separation of heavy metals from water and wastewater. Biochem Eng J. 2009;44(1):19–41. doi: 10.1016/j.bej.2008.12.009
- Ahluwalia SS, Goyal D. Microbial and plant derived biomass for removal of heavy metals from wastewater. Biores Technol. 2007;98(12):2243–2257. doi: 10.1016/j.biortech.2005.12.006
- Srivastava SK, Tyagi R, Pant N. Adsorption of heavy metal ions on carbonaceous material developed from the waste slurry generated in local fertilizer plants. Water Res. 1989;23(9):1161–1165. doi: 10.1016/0043-1354(89)90160-7
- Kapoor A, Viraraghavan T. Fungal biosorption – an alternative treatment option for heavy metal bearing wastewaters: a review. Bioresource Technology. 1995;53(3):195–206. doi: 10.1016/0960-8524(95)00072-M
- Li J, Wang X, Zhao G, et al. Metal–organic framework-based materials: superior adsorbents for the capture of toxic and radioactive metal ions. Chem Soc Rev. 2018;47(7):2322–2356. doi: 10.1039/C7CS00543A
- Singh J, Dutta T, Kim KH, et al. ‘Green’ synthesis of metals and their oxide nanoparticles: applications for environmental remediation. J Nanobiotechnol. 2018;16(84). doi: 10.1186/s12951-018-0408-4
- Arthiga D, Anthony SP. Selective colorimetric sensing of toxic metal cations by green synthesized silver nanoparticles over a wide pH range. RSC Adv. 2013;3(37):16765–167174. doi: 10.1039/c3ra42308e
- Abegunde S, Idowu K, Sulaimon A. Plant-mediated iron nanoparticles and their applications as adsorbents for water treatment – A review. J Chem Rev. 2020;2(2): 103–113. doi: 10.33945/SAMI/JCR.2020.2.3
- Herlekar M, Barve S, Kumar R. Plant-mediated green synthesis of iron. Nanopart J Nanopart. 2014;2014:1–9. doi: 10.1155/2014/140614
- Rao A, Bankar A, Kumar AR, et al. Removal of hexavalent chromium ions by yarrowia lipolytica cells modified with phyto-inspired FeO/Fe3O4 nanoparticles. J Contam Hydrol. 2013;146:63–73. doi: 10.1016/j.jconhyd.2012.12.008
- Madhavi V, Prasad TN, Reddy AV, et al. Application of phytogenic zerovalent iron nanoparticles in the adsorption of hexavalent chromium. Spectrochim Acta A. 2013;116:17–25. doi: 10.1016/j.saa.2013.06.045
- Savasari M, Emadi M, Bahmanyar MA, et al. Optimization of Cd(II) removal from aqueous solution by ascorbic acid-stabilized zero valent iron nanoparticles using response surface methodology. J Ind Eng Chem. 2015;21:1403–1409. doi: 10.1016/j.jiec.2014.06.014
- Mystrioti C, Xenidis A, Papassiopi N. Reduction of hexavalent chromium with polyphenol-coated nano zero-valent iron: column studies. Desalin Water Treat. 2014;56(5):1162–1170. doi: 10.1080/19443994.2014.941298
- Shaidatul M, Mohammad Hilni H, Ai Ling T, et al. Plant-extract-mediated SnO2 nanoparticles: synthesis and applications. ACS Sustainable Chem Eng. 2020;8(8):3040–3054.
- Nikam A, Pagar T, Ghotekar S, et al.A review on plant extract mediated green synthesis of zirconia nanoparticles and their miscellaneous applications. J Chem Rev. 2019;1(3):154–163.
- Pirtarighat S, Ghannadnia M, Baghshahi S. Green synthesis of silver nanoparticles using the plant extract of Salvia spinosa grown in vitro and their antibacterial activity assessment. J Nanostruct Chem. 2019;9(1):1–9. doi: 10.1007/s40097-018-0291-4
- Önal ES, Yatkın T, Aslanov T, et al. Biosynthesis and characterization of iron nanoparticles for effective adsorption of Cr (VI). Int J Chem Eng. 2019;2019:1–13. Article ID 2716423. doi: 10.1155/2019/2716423
- Badruddoza AZM, Shawon ZBZ, Rahman MT, et al. Ionically modified magnetic nanomaterials for arsenic and chromium removal from water. Chem Eng J. 2013;225:607–615. doi: 10.1016/j.cej.2013.03.114
- Badruddoza AZM, Shawon ZBZ, Rahman MT. Ionically modified magnetic nanomaterials for arsenic and chromium removal from water. Chem Eng J. 2013;225:607–615. doi: 10.1016/j.cej.2013.03.114
- Ngomsik A, Bee A, Talbot D, et al. Magnetic solid–liquid extraction of Eu(III), La(III), Ni(II) and Co(II) with maghemite nanoparticles. Sep Purif Technol. 2012;86:1–8. doi: 10.1016/j.seppur.2011.10.013
- Tan L, Xu J, Xue X, et al., (2014) Multifunctional nanocomposite Fe3O4@SiO2–mPD/SP for selective removal of Pb(ii) and Cr(vi) from aqueous solutions. RSC Adv. 4: 45920–45929. 10.1039/C4RA08040H 86
- Ehrampoush MH, Miria M, Salmani MH, et al. Cadmium removal from aqueous solution by green synthesis iron oxide nanoparticles with tangerine peel extract. J Environ Health Sci Eng. 2015;13(84):1–7. PMID: 26682059; PMCID: PMC4682273. doi: 10.1186/s40201-015-0237-4.
- Rathod Prakash B, Ajish Kumar KS, Athawale Anjali A, et al. Poly(ethylenimine) functionalized magnetic nanoparticles for sorption of Pb, Cu, and Ni: potential application in catalysis. Separation Sci Technol. 2018;54(10) : 1588–1598. doi: 10.1080/01496395.2018.1520731
- Nithya K, Sathish A, Kumar PS, et al. Fast kinetics and high adsorption capacity of green extract capped superparamagnetic iron oxide nanoparticles for the adsorption of Ni (II) ions. J Ind Eng Chem. 2018;59:230–241. doi: 10.1016/j.jiec.2017.10.028
- Gupta VK, Nayak A. Cadmium removal and recovery from aqueous solutions by novel adsorbents prepared from orange peel and Fe2O3 nanoparticles. Chem Eng J. 2012;180:81–90. doi: 10.1016/j.cej.2011.11.006
- Mahmoud ME, El-Sharkawy RM Promoted adsorptive removal of chromium(vi) ions from water by a green-synthesized hybrid magnetic nanocomposite (NFe3O4Starch-Glu-NFe3O4ED), RSC Adv. 2021;11(24):14829–14843. doi: 10.1039/D1RA00961C