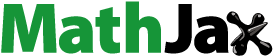
ABSTRACT
The availability of clean and safe water is a fundamental necessity for the sustenance of life and the well-being of our planet. Photocatalytic water treatment using nanomaterials has emerged as a promising and cutting-edge approach to address this pressing environmental challenge. In this work, a co-precipitation method was employed to synthesize europium (Eu3+) doped gadolinium metavanadate (GdVO4). The GdVO4:Eu3+ was encapsulated in polyvinyl alcohol (PVA). The GdVO4:Eu3+ and PVA-GdVO4:Eu3+ were characterized by X-ray diffraction (XRD), transmission electron microscopy (TEM), scanning electron microscopy (SEM), Fourier-transform infrared (FTIR) spectroscopy, Raman spectroscopy, thermogravimetric analysis (TGA), photoluminescence (PL), and UV–visible spectroscopy (UV-Vis). The XRD, FTIR, and Raman spectroscopy results confirmed the formation of both GdVO4:Eu3+ crystals and PVA-GdVO4:Eu3+ nanocomposite. Both compounds’ photoluminescence spectra demonstrated effective energy transfer from the GdVO4 host to the Eu3+. Results obtained from the TGA indicate that PVA-GdVO4:Eu3+ nanocomposite is stable at a temperature of 330°C. Under UV irradiation, materials’ photocatalytic efficiency was examined regarding their ability to degrade Eosin Y dye in water. Results from the photocatalytic studies of the synthesized PVA-GdVO4:Eu3+ showed improved photocatalytic activity compared to GdVO4:Eu3+ under the same experimental conditions. When operating under ideal conditions of pH = 2, initial dye concentration of 30 ppm, a catalyst dosage of 200 mg, the degradation of Eosin Y surpassed 95% within 150 min of exposure to light.
1. Introduction
When it comes to dying, printing, leather, paper products, and luminous pigments, Eosin Y, a heterocyclic dye with bromine atoms, is frequently utilized (Yadav et al., Citation2022). Due to its vibrant hue, Eosin Y is utilized in painting and colouring companies (Sabnis, Citation2017). Anthropogenic activities that lead to the direct discharge of wastewater that contains Eosin Y can pose serious environmental effects due to its toxicity and carcinogenicity and its resistance to conventional chemical and biological treatment methods (Zhu et al., Citation2016). It is therefore important to eliminate this dye in water using a simple and cost-effective technique.
Most techniques such as coagulation, flocculation, membrane separation and adsorption on activated carbon, which are only based on the phase transfer mechanism of pollutants, are employed for the removal of dyes from water (Afroze & Kanti Sen, Citation2018). However, these methods have a lot of drawbacks such as high operational costs and the generation of high amounts of sludges. Photocatalysis includes the degradation of contaminants from complex compounds into simple and safer chemicals, contrary to other traditional techniques of environmental remediation (Ameta et al., Citation2018). As a result, there is no sediment or waste left behind from the operation, and the catalyst was unaltered continuously, allowing it to be reused (Karthik et al., Citation2017). Rare earth orthovanadates RVO4 (R = Y, Nd, Gd) have dominated recent studies due to their versatile applications (Jing et al., Citation2016). Trivalent lanthanide ions have been used as hosts in many light-emitting materials because of their greater spectral properties including narrow-band emission and long lifetime (Singh et al., Citation2022). There has been a wide range of applications of Eu3+ because of the increased luminescent efficiency it possesses (Pravinraj et al., Citation2017). Luminescent BiVO4:Eu3+ has been reported to be a promising photocatalyst for water treatment. Highly luminescent red emitting GdVO4:Eu3+ has also been applied in many fields such as cathode ray tubes, X-Ray detectors, and lamps (Thakur et al., Citation2021). Its great ultraviolet light absorption, efficient energy transfer from light VO43- to Eu3+, and efficient stimulation of Eu3+ by the 450 nm intense emission of VO43- are what cause the material to glow red (Huang et al., Citation2013). Similar to YVO4:Eu3+ and LuVO4:Eu3+, GdVO4:Eu3+ has luminous intensity. Of all the rare earth-doped vanadates, it is said that GdVO4:Eu3+ has the highest luminescence intensity (González-Penguelly et al., Citation2022).
Composites of intrinsically conducting polymers have drawn considerable interest for their worldwide applications (Xu et al., Citation2021). Composites of conducting polymers are made of conjugated polymers and at least one inorganic or organic material (Sardana et al., Citation2022). Alternatives to traditionally filled polymers include polymer nanocomposites. The filler’s size leads to better distribution, which enhances the synergistic effect of the different components’ features in the nanocomposite material.
Several efforts have been made to find ways to disseminate the nanomaterials in water and make them mobile since they must be in a non-agglomerated state to be employed in water contamination cleanup. Several biopolymer-based composites such as starch (Rao et al., Citation2014), polylactic acid delivery vehicles (Bhattarai et al., Citation2017) and modified cellulose and various polymers have been tested and proven to be extremely effective. PVA is a biopolymer that has been used extensively as a host for many nanosized materials due to its high mechanical and thermal properties, conductivity, and biocompatibility (Jiang & Dawei, Citation2022; Rol et al., Citation2019).
In this study, we report an easy and environmentally friendly method for synthesizing GdVO4:Eu3+ nanoparticles. The synthesized GdVO4:Eu3+ nanoparticles were then encapsulated in a PVA polymer matrix to form PVA-GdVO4:Eu3+. Moreover, the photocatalytic activities of GdVO4:Eu3+ and PVA-GdVO4:Eu3+ in the degradation of Eosin Y were also discussed.
2. Experimental
None of the chemicals used for the experiment were subjected to additional processing; they were all of analytical grade. Gadolinium nitrate hexahydrate (Gd(NO3)3·6 H2O), ammonia solution, Eosin Y, Vanadium pentoxide(V2O5), europium nitrate hexahydrate (Eu(NO3)3·6 H2O), and polyvinyl alcohol (PVA) were obtained from Sigma Aldrich, Germany.
2.1. Preparation of GdVO4:Eu3+
V2O5 (0.091 g) was dissolved in 10 mL ammonia solution (5% v/v) and heated at 80°C on a magnetic stirrer to dissolve. Gd(NO3)3·6 H2O (0.5 mmol) was dissolved into 10 mL dilute HNO3. In another beaker, Eu(NO3)3·6 H2O (2.5 mL of 0.01 M) solution was added to it. After stirring for 30 mins, this solution was added to the V2O5-ammonia solution, and the pH of the mixture was raised to 8.0 using NH4OH. The yellow precipitate formed was centrifuged and washed sequentially with ethanol and distilled water. The resultant precipitates were heated at 150°C for 6 hrs.
2.2. Synthesis of PVA-GdVO4:Eu3+
PVA (0.9 g) was slowly dispersed into distilled water (10 mL) whilst on a hot plate magnetic stirrer. The pre-prepared GdVO4:Eu3+ (0.1 g) was added to the reaction mixture and was heated for 30 mins and sonicated for 45 minutes. The mixture was then cast on a cleaned glass plate, dried at 50°C and pulverized.
2.3. Testing photocatalytic activity of materials
Using a Camag UV-Cabinet II (= 366 nm) at room temperature, a suspension of 100 mg of the catalyst in 100 mL of an aqueous solution of Eosin Y (30 mg/L) was put under mechanical stirring in order to study the photocatalytic activity of the materials. The lamp is operated at 12 V which is internally converted to 25–30 kHz at a high UV lamp long-wave at 2 × 8 W. The pH of the dye solution was adjusted in the range of 2.0 to 7.0 using dilute HNO3 or NaOH to obtain the optimum pH for the degradation reaction of the samples. One hour before light illumination, the materials were magnetically stirred in the dark to enable adsorption equilibrium. A disposable syringe was used to remove aliquots of the suspension (5 mL) at regular time intervals. Centrifugation was used to separate the mixture from the supernatant for 15 minutes at 3000 rpm. Using a Shimadzu UV-2450 spectrophotometer set to a wavelength of 515 nm, the amount of Eosin Y left in the supernatant solution after irradiation was measured. Dye degradation efficiency was determined as stated in Equation 1 (Chen et al., Citation2020).
Where Co and C represent, respectively, the initial and remaining dye concentrations at a particular time.
2.4. Sample characterization
The samples’ X-ray diffractograms were captured using a Rigaku Ultima IV X-ray diffractometer with Cu K radiation, while their TEM images were captured using a field emission electron microscope (JEM-2100F). The SEM analysis was performed using a TESCAN (Vega 3 XMU) to determine the surface morphology and particle size. FT-IR spectra were obtained using a PerkinElmer Spectrum 100 FT-IR spectrometer, the Raman spectra were taken using a PerkinElmer Raman microscope (Raman Micro 200), optical absorption spectra were recorded using a Shimadzu UV–Visible spectrophotometer (UV-2450), photoluminescence studies were performed using PerkinElmer fluorescence spectrometer (LS:45), and TGA measurements were undertaken using PerkinElmer thermogravimetric analyzer (TGA 4000).
3. Results and discussion
3.1. XRD analysis
Figure shows the XRD pattern of the as-prepared Eu3+ doped GdVO4. All the peaks matched with the standard diffraction data for the bulk GdVO4 with a tetragonal gadolinium structure (JCPDS, No. 17–0260). There were no further peaks, indicating that the tetragonal gadolinium structure was formed in a single crystal and that the Eu3+ ions had been incorporated into the GdVO4 host crystal lattice. The values measured from the XRD patterns for the as-prepared GdVO4:Eu3+ powder and those given in JCPDS No. 17–0260 are listed in Table . The enlarged d values of the strongest peaks show that the Eu3+ ions in the host material partially replaced Gd3+ ions. This is due to the relatively large ionic radius of Eu3+ ions (94.7 pm) compared to that of Gd3+ ions (93.8 pm). A similar report was reported by other researchers (Szczeszak et al., Citation2014) while studying the structural and spectroscopic properties of Eu3+ doped GdVO4.
Table 1. d, values measured by XRD diffraction
3.2. FTIR analysis
The FTIR data of materials provide good information about the functional groups and surface chemistry. The FTIR spectra of GdVO4:Eu3+ and PVA-GdVO4:Eu3+ nanocomposite are shown in Figure . There were four major peaks observed at 792, 1024, 1387, and 3650 cm−1 for GdVO4:Eu3+ (). The bulk information about lattice vibration of GdVO4 nanoparticle is indicated by its strong absorption at ~800 cm−1, which is assigned to the V-O vibration stretch of the host lattice (Szczeszak et al., Citation2014). Because nanocomposites are typically impacted by adsorbed water molecules, which have various distinctive IR bands as established by several researchers, the IR spectrum also reveals the surface chemistry of the nanocomposites. The characteristic broad peaks of the GdVO4:Eu3+ at 3650 cm−1 and the sharp peak at 1024 cm−1 may be attributed to O-H and C-O stretch of ethanol used for washing the samples, respectively. The peaks observed at 792 and 1387 cm−1 correspond to V-O and bending vibrations of CH2.
The FT-IR spectrum of Figure shows the characteristic absorption bands of PVA-GdVO4:Eu3+ located at 841 and 3673 cm−1 which is attributed to V-O stretching vibrations and O-H stretches, indicating the formation of the nanocomposite. The V-O stretch shifted from 792 to 842 cm−1 and in addition, the stretching vibration of O-H at 3650 cm−1 shifted to 3673 cm−1, indicating that the GdVO4:Eu3+ nanoparticle was bound to the OH– functional groups of PVA (Mansur et al., Citation2004; Sudhamani et al., Citation2003). The absorption band at 2919 cm−1 is due to the C-H symmetric stretch. The bands at 1733 and 1427 cm−1 can be assigned to C=O and C=C stretches, respectively. The weak peak at ~2161 cm−1 corresponds to the combination frequency of (C-H + C-C), whereas the strong peak at ~1343 cm−1 was due to the combination frequency of (C-H+O-H) group (Abral et al., Citation2019).
3.3. Raman spectroscopy
The measured Raman spectra of zircon-type GdVO4:Eu3+ and PVA-GdVO4:Eu3+ nanocomposite are shown in Figure . The Raman spectrum for the GdVO4:Eu3+ in Figure showed only at 8 of 11 Raman active modes, which were in accord with other studies (Pudkon et al., Citation2016). With the zircon-type configuration, the interior stretching and bending vibrations of the VO4 tetrahedra are detected in the regions in the range of 260–1000 cm−1, whereas exterior modes are seen below 250 cm−1. The weak bands at 122 and 153 cm−1 correspond to B1 g and Eg external modes. The 153 cm−1 absorption peak is due to O-Gd-O bends. The strong and sharp peak at 384 cm−1 (υ2) indicates O-V-O bends. The absorption bands at 825 cm−1 correspond to a combined frequency of (Eg and B1 g) modes, which can be assigned to VO4 stretches. The absorption peak at 883 cm−1 (υ1) corresponds to VO4 asymmetric stretch (Bespalova et al., Citation2017). The weak peak 2807 is assigned to C-H stretches.
Figure shows the Raman spectrum of PVA- GdVO4:Eu3+ nanocomposite. The absorption bands at 1366 and 2827 cm−1 are ascribed to C-H stretches, and the broad and strong peak at 1440 cm−1 is due to –CH2 deformation. The peak at 1150 cm−1 corresponds to the C=C stretch. The results from the Raman spectrum of the nanocomposite also confirm the incorporation of the GdVO4:Eu3+ nanoparticle into the PVA. The weak absorption peak at 883 cm−1 can be assigned to A1 g(υ1) band (Mansur et al., Citation2008), which corresponds to VO4 asymmetric stretch. The results from Raman studies therefore collaborate with the FTIR results, showing the presence of GdVO4:Eu3+ nanocomposite in PVA.
3.4. TEM and SEM analysis
The morphology of the prepared GdVO4:Eu3+ powder and PVA-GdVO4:Eu3+ nanocomposites was observed by TEM and SEM. Figure shows the TEM images of GdVO4:Eu3+ grains. The particles are rice-like in structure and uniform in shape with an average size of 37 nm in width and 96 nm in length with good dispersity. The crystals were not tightly aggregated. Li et al. (Citation2016), reported a similar effect while studying the photoluminescence properties of Dy 3+, Eu3+, and doped GdVO4. SEM images of the PVA-GdVO4:Eu3+ nanocomposite are shown in Figure . PVA coatings are responsible for the rise in particle diameter shown in the photograph. The enlarged size of the PVA coated GdVO4:Eu3+ showed no agglomeration which will enhance its photocatalytic performance and the recovery of the material after photocatalysis. The elemental composition of the GdVO4:Eu3+ nanoparticles was investigated by EDX analysis. The signals observed in Figure show that the main elemental components are Gd, V, O, and Eu, confirming the formation of GdVO4:Eu3+.
3.5. Thermogravimetric analysis
The representative TGA spectra for PVA-GdVO4:Eu3+ and GdVO4:Eu3+ in a nitrogen-free environment are shown in Figure . According to Figure , the weight loss did not significantly change across the full temperature range of 50°C to about 800°C. The evaporation of the surface that absorbed moisture is what causes the weight loss between 50°C and 100°C. The unstable nature of the VO4 subunits in the complex is what causes the steady decline in weight loss at elevated temperatures (Sudhamani et al., Citation2003). The PVA-GdVO4:Eu3+ nanocomposite’s TGA spectrum is depicted in Figure . Water loss, caused by the loss of moisture and perhaps other volatile organic molecules in the composites, is the first step of weight loss between 50°C and 120°C. At approximately 120°C and 240°C, where active pyrolysis began, little weight loss was seen. PVA is decomposing at temperatures between 120°C and 330°C, which causes a weight loss of roughly 67%. The temperature range between 400°C and 540°C shows the last stage of pyrolysis of all organic compounds in the PVA-GdVO4:Eu3+ nanocomposite and the gradual reduction in the weight at higher temperatures is due to the instability of VO4 groups (Huang et al., Citation2013, Citation2012; Sudhamani et al., Citation2003). This shows that PVA-GdVO4:Eu3+ is stable up to approximately 330°C, whereas pure PVA is known to be stable at 340°C (Liang et al., Citation2018; Shandilya et al., Citation2019; Song et al., Citation2017).
3.6. Photoluminescence excitation
The luminescence properties of GdVO4:Eu3+ and PVA-GdVO4:Eu3+ were measured by their fluorescence spectrum. The peak shapes of both samples are almost similar. The emission wavelength of 616 nm (Yi et al., Citation2006), at which excitation occurs is shown in Figure . The excitation consists of a narrow band with a strong and sharp peak center at about 312 nm and another peak at 280 nm. This indicates that there is a complete transfer of energy from the GdVO4 host to the Eu3+ ions (Tang et al., Citation2012). The strong and sharp peak at 312 nm in both GdVO4:Eu3+ and PVA-GdVO4:Eu3+ can be ascribed to the general f-f transitions of Eu3+. These sharp peaks observed between 200 and 350 nm can be attributed to the charge transfer band of Eu-O and V-O (Jovanović et al., Citation2013). The peaks centered at 280 nm can be assigned to the GdVO4 host (VO43-) excitation band and the 8S-6D transitions, respectively (Thakur et al., Citation2021).
Figure also shows the emission spectra of GdVO4:Eu3+ and PVA-GdVO4:Eu3+ excited at 260 nm. Both spectra show a strong and sharp red emission peak at 520 nm and another peak at 571 nm. These narrow and sharp peaks observed in the spectrum can be attributed to the shielding of the 4f electrons by the 5s and 5p electrons in the outer shells in the Eu3+ ions, and the emission peaks observed at about 521 nm are due to Eu3+ 5D1-7F1 transitions (Chung et al., Citation2009; Shim et al., Citation2007).
When the as-synthesized materials were exposed to 254 (365) nm UV light, the materials emit bright red light. The relevant UV–vis absorption spectra for GdVO4:Eu3+ and PVA-GdVO4:Eu3+ are depicted in Figure . There are both clearly strong UV and weak visible light absorption peaks in the entire absorption spectrum of the samples. The major peak in the UV region for the GdVO4:Eu3+ may be as a result of charge transfer band between V5+ and O2-. These strong UV absorption bands may also originate from the vanadate (VO4)3- group, which acts as a self- activated host (Chung et al., Citation2009; Shim et al., Citation2007).
3.7. Photocatalytic experiment
The photocatalytic experiments on the degradation of Eosin Y were carried out using GdVO4:Eu3+ and PVA-GdVO4:Eu3+ nanocomposite. The photocatalytic activity of the PVA-GdVO4:Eu3+ was found to degrade about 95% of the dye within 150 min (k = 1.70 × 10−2 min−1) than the GdVO4:Eu3+ (k = 8.47 × 10−3 min−1) (Figure ). The percentage degradation obtained is similar to what was earlier reported with similar material (Table ).
Figure 9. (a) Percentage removal vs irradiation time and (b) kinetics of degradation of Eosin Y using PVAGdVO4 and GdVO4.

Table 2. Comparison of photocatalytic activity of material with other works
Various pH values (2, 3, 4, 5, 6, and 7) using GdVO4:Eu3+ for optimization at constant concentration of Eosin Y (30 mg/L) and different catalyst loading (50, 100, 150, and 200 mg) using PVA-GdVO4:Eu3+ for 150 mins were determined. The results of photodegradation are depicted in Figures .
Figure 10. (a) Effect of pH on the degradation of Eosin Y and (b) percentage removal of Eosin Y at different pH using PVAGdVO4:Eu3+. Catalyst amount = 200 mg/100 mL of 30 ppm Eosin Y.

Figure 11. (a) Effect of catalyst concentration on the degradation of Eosin Y (30 ppm) using PVA-GdVO4:Eu3+ at pH=2 and (b) percentage removal vs irradiation time as a function of catalyst loading.

3.7.1. Effect of pH on the degradation of Eosin Y using PVA-GdVO4:Eu3+ catalyst
From Figure , it was obvious that the rate of degradation increased significantly in Eosin Y with a decrease in pH. This is because Eosin Y (anionic dye) degrades faster at lower pH. This may be due to the creation of positive charges on the surface of the catalyst which leads to the attraction of the anionic dye for enhanced degradation.
3.7.2. Effect of catalyst concentration on the degradation of Eosin Y using PVA-GdVO4:Eu3+ at pH = 2
The effect of Eosin Y dye concentration on PVA-GdVO4:Eu3+ loading on the rate of degradation was performed by varying the amount of PVA-GdVO4:Eu3+ catalyst loading from 50 mg to 200 mg at pH = 2 with fixed dye concentration (30 ppm) (Agorku et al., Citation2014; Maksimchuk et al., Citation2020; Oppong et al., Citation2018). Figure shows the effect of the loading of the catalyst on the rate of photodegradation of the dye. From Figure , it can be observed that the amount of the PVA-GdVO4:Eu3+ influences the degradation rate of Eosin Y. The rate increased with an increasing amount of the catalyst and the concentration of the Eosin Y dye inclined from 85% (50 mg) to 92% (200 mg) after 150 min of illumination. The rise in the rate of photodegradation can be attributed to OH. radicals formed which has a great impact on the process of degradation (Oppong et al., Citation2021). A rise in catalyst mass corresponds to an increase in the formation of OH. radicals, leading to a rise in the rate of degradation of the dye molecule. Active charge carrier transfer, a narrower band gap, and delayed charge carrier recombination as a result of Gd and Eu trapping electrons in the conduction band could all account for the higher photocatalytic activity (Figure ).
In general, it can be noted that the rate of degradation of the dye did not change much with catalyst loading at 150 mg (91%) and 200 mg (92%) and this may be due to an increase in light scattering (Agorku et al., Citation2014). As a result, using more catalysts may not be beneficial due to the possibility of clumping as well as the lower irradiation field resulting from a rise in dispersion (Sakthivel et al., Citation2003).
4. Conclusion
Luminescent GdVO4:Eu3+ was prepared by co-precipitation method and PVA-GdVO4:Eu3+ nanocomposite by the simple wet-processing method. The TEM and SEM results of the GdVO4:Eu3+ powder showed a rice-like structure and are uniform in shape with an average size of 37 nm in width and 96 nm in length with good dispersity. XRD, FTIR, and Raman spectroscopy results confirmed the formation of both GdVO4:Eu3+ and PVA-GdVO4:Eu3+ nanocomposite. Both materials displayed visible red-light emission under UV excitation. TGA results showed insignificant weight loss for GdVO4:Eu3+ powder over the entire temperature range between 50°C and 800°C, whereas the PVA-GdVO4:
Eu3+nanocomposite exhibited significant weight loss (67%) between 120°C and 240°C, and this may be due to pyrolysis of PVA in the nanocomposite. The photocatalytic activities of the PVA-GdVO4:Eu3+ decreased markedly with a decrease in pH. The PVA-GdVO4:Eu3+ showed a high rate of Eosin Y degradation at low catalyst loading, which suggests that low catalyst loading may be more useful than high loading. The optimum catalyst dosage required for the degradation of 30 mg/L of Eosin Y in 150 min at a pH of 2 was 200 mg.
Data accessibility
The data used in this research article came from our experiments. The data used to support the study’s findings are included in the article and can also be obtained upon request from the corresponding author.
Ethical statement
This article does not address analysis with ethical concerns.
Acknowledgements
The authors will like to thank the Faculty of Science, University of Johannesburg, South Africa, and the Nanotechnology and Applications Centre, University of Allahabad, Allahabad, India.
Disclosure statement
No potential conflict of interest was reported by the author(s).
Additional information
Funding
References
- Abral, H., Mahardika, M., Handayani, D., Sugiarti, E., & Novi Muslimin, A. (2019). Characterization of disintegrated bacterial cellulose nanofibers/PVA bionanocomposites prepared via ultrasonication. International Journal of Biological Macromolecules, 135, 591–12. https://doi.org/10.1016/j.ijbiomac.2019.05.178
- Afroze, S., & Kanti Sen, T. (2018). A review on heavy metal ions and dye adsorption from water by agricultural solid waste adsorbents. Water, Air, and Soil Pollution, 229, 1–50. https://doi.org/10.1007/s11270-018-3869-z
- Agorku, E. S., Mittal, H., Mamba, B. B., Pandey, A. C., & Mishra, A. K. (2014). Fabrication of photocatalyst based on Eu3±doped ZnS–SiO2 and sodium alginate core shell nanocomposite. International Journal of Biological Macromolecules, 70, 143–149. https://doi.org/10.1016/j.ijbiomac.2014.06.037
- Ameta, R., Solanki, M. S., Benjamin, S., & Ameta, S. C. (2018). Photocatalysis. In Advanced oxidation processes for waste water treatment (pp. 135–175). Elsevier. https://doi.org/10.1016/B978-0-12-810499-6.00006-1
- Bespalova, I. I., Yefimova, S. L., Tkacheva, T. N., Hubenko, K. А., Sorokin, А. V., & Mateychenko, Р. V. (2017). GdVO: Еu nanoparticles Û embedded CaCO microspheres: Synthesis and characterization. Functional Materials, 3, 393. https://doi.org/10.1016/j.cis.2003.07.002
- Bhattarai, R. S., Das, A., Alzhrani, R. M., Kang, D., Bhaduri, S. B., & Boddu, S. H. (2017). Comparison of electrospun and solvent cast polylactic acid (Pla)/poly (vinyl alcohol)(PVA) inserts as potential ocular drug delivery vehicles. Materials Science & Engineering C, 77, 895–903. https://doi.org/10.1016/j.msec.2017.03.305
- Chen, Q., Yan, Z., Guo, L., Zhang, H., Zhang, L., Kim, K., Li, X., & Wang, W. (2020). Enhancing the acid orange dye degradation efficiency of mg-based glassy alloys with introducing porous structure and zinc oxide. Journal of Alloys and Compounds, 831, 154817. https://doi.org/10.1016/j.jallcom.2020.154817
- Chung, J. W., Yang, H. K., Moon, B. K., Choi, B. C., Jeong, J. H., Bae, J. S., & Kim, K. H. (2009). The dependence of temperature synthesis of GdVO4: Eu3+ nanoparticle phosphors by solvothermal method. Current Applied Physics, 9(3), S222–S5. https://doi.org/10.1016/j.cap.2009.01.046
- González-Penguelly, B., Pérez-Sánchez, G. G., Medina-Velázquez, D. Y., Martínez-Falcón, P., & de Jesús Morales-Ramírez, A. (2022). Luminescence properties and energy transfer of Eu3+, Bi3+ co-doped LuVO4 films modified with pluronic F-127 obtained by sol–gel. Materials, 16(1), 146. https://doi.org/10.3390/ma16010146
- He, Y., Cai, J., Li, T., Wu, Y., Lin, H., Zhao, L., & Luo, M. (2013). Efficient degradation of RhB over GdVO4/g-C3N4 composites under visible-light irradiation. Chemical Engineering Journal, 215-216, 721–730. https://doi.org/10.1016/j.cej.2012.11.074
- Huang, S., Cheng, Z., Kang, X., Dai, Y., & Lin, J. (2013). Luminescent GdVO4: Eu 3+ functionalized mesoporous silica nanoparticles for magnetic resonance imaging and drug delivery. Dalton Transactions, 42(18), 6523–6530. https://doi.org/10.1039/C3DT33114H
- Huang, Z., Huang, S., Gang, O., & Pan, W. (2012). Systhesis, phase transformation and photoluminescence properties of Eu: La 1− x gdx VO4 nanofibers by electrospinning method. Nanoscale, 4(16), 5065–5070. https://doi.org/10.1039/C2NR31135F
- Jiang, H. E., & Dawei, W. A. N. G. (2022). Modification and research progress of cellulose materials. Acta Materiae Compositae Sinica, 39(7), 3121–3130. https://doi.org/10.5755/j02.ms.25485
- Jing, L., Liu, X., Yuntong, L., & Wang, Y. (2016). Green-to-red tunable luminescence of Eu 3+-doped K 3 Y (VO 4) 2 phosphors. Journal of Materials Science, 51, 903–910. https://doi.org/10.1007/s10853-015-9418-x
- Jovanović, D. J., Antić, Ž., Krsmanović, R. M., Mitrić, M., Đorđević, V., Bártová, B., & Dramićanin, M. D. (2013). Annealing effects on the microstructure and photoluminescence of Eu3+-doped GdVO4 powders. Optical Materials, 35(10), 1797–1804. https://doi.org/10.1016/j.optmat.2013.03.012
- Karthik, R., Govindasamy, M., Chen, S.-M., Cheng, Y.-H., Muthukrishnan, P., Padmavathy, S., & Elangovan, A. (2017). Biosynthesis of silver nanoparticles by using camellia japonica leaf extract for the electrocatalytic reduction of nitrobenzene and photocatalytic degradation of Eosin Y. Journal of Photochemistry and Photobiology B, Biology, 170, 164–172. https://doi.org/10.1016/j.jphotobiol.2017.03.018
- Li, H., Liu, G., Wang, J., Dong, X., & Wensheng, Y. (2016). Dual-mode, tunable color, enhanced upconversion luminescence and magnetism of multifunctional BaGdF5: Ln3+(Ln= Yb/Er/Eu) nanophosphors. Physical Chemistry Chemical Physics, 18(31), 21518–21526. https://doi.org/10.1039/C6CP03743G
- Liang, Y., Mi Noh, H., Heum Park, S., Chun Choi, B., & Hyun Jeong, J. (2018). Colloidal GdVO4: Eu3+@ SiO2 nanocrystals for highly selective and sensitive detection ofCu2+ions. Applied Surface Science, 433, 3817. https://doi.org/10.1016/j.apsusc.2017.10.058
- Long, M., Cai, W., Cai, J., Zhou, B., Chai, X., & Wu, Y. (2006). Efficient photocatalytic degradation of phenol over Co3O4/BiVO4 composite under visible light irradiation. The Journal of Physical Chemistry, 110(41), 20211–20216. https://doi.org/10.1021/jp063441z
- Maksimchuk, P. O., Yefimova, S. L., Hubenko, K. O., Omielaieva, V. V., Kavok, N. S., Klochkov, V. K., Sorokin, O. V., & Malyukin, Y. V. (2020). Dark reactive oxygen species generation in ReVO4: Eu3+ (Re= Gd, Y) nanoparticles in aqueous solutions. The Journal of Physical Chemistry, 124(6), 3843–3850. https://doi.org/10.1021/acs.jpcc.9b10143
- Mansur, H. S., Oréfice, R. L., & Mansur, A. A. (2004). Characterization of poly (vinyl alcohol)/poly (ethylene glycol) hydrogels and PVA-derived hybrids by small-angle X-ray scattering and FTIR spectroscopy. Polymer, 45(21), 7193–7202. https://doi.org/10.1016/j.polymer.2004.08.036
- Mansur, H. S., Sadahira, C. M., Souza, A. N., & Mansur, A. A. (2008). FTIR spectroscopy characterization of poly (vinyl alcohol) hydrogel with different hydrolysis degree and chemically crosslinked with glutaraldehyde. Materials Science & Engineering, 28(4), 539–548. https://doi.org/10.1016/j.msec.2007.10.088
- Mondal, M., Banerjee, S., Halder, M., Das, S., & Pradhan, S. (2022). Synthesis of a novel visible-light active Gd2O3/GdVO4/V2O5 photocatalyst for rapid purification of industrial wastewater containing organic dyes and bacteria. Journal of Molecular Liquids, 368, 120512. https://doi.org/10.1016/j.molliq.2022.120512
- Oppong, S. O.-B., Opoku, F., Wilson Anku, W., & Govender, P. P. (2021). Insights into the complementary behaviour of Gd doping in GO/Gd/ZnO composites as an efficient candidate towards photocatalytic degradation of indigo carmine dye. Journal of Materials Science, 56, 8511–8527. https://doi.org/10.1007/s10853-021-05846-w
- Oppong, S. O., Wilson Anku, W., Opoku, F., Kumar Shukla, S., & Poomani Govender, P. (2018). Photodegradation of Eosin yellow dye in water under simulated solar light irradiation using La–doped ZnO nanostructure decorated on graphene oxide as an advanced photocatalyst. ChemistrySelect, 3(4), 1180–1188. https://doi.org/10.1002/slct.201702470
- Pravinraj, S., Vijayakumar, M., & Marimuthu, K. (2017). Enhanced luminescence behaviour of Eu3+ doped heavy metal oxide telluroborate glasses for laser and LED applications. Physica B, Condensed Matter, 509, 84–93. https://doi.org/10.1016/j.physb.2017.01.008
- Pudkon, W., Kittiwachana, S., Thongtem, T., Thongtem, S., & Kaowphong, S. (2016). Influence of mixed solvents on characteristics and photoluminescence of GdVO4: Eu3+ nanophosphors synthesized by solvothermal method. Science of Advanced Materials, 8(1), 138–143. https://doi.org/10.1166/sam.2016.2617
- Rao, M. G., Bharathi, P., & Akila, R. M. (2014). A comprehensive review on biopolymers. Science Reviews Chemical Communication, 4(2), 61–68.
- Rol, F., Naceur Belgacem, M., Gandini, A., & Bras, J. (2019). Recent advances in surface-modified cellulose nanofibrils. Progress in Polymer Science, 88, 241–264. https://doi.org/10.1016/j.progpolymsci.2018.09.002
- Sabnis, R. W. (2017). Manufacture of dye intermediates, dyes, and their industrial applications. Handbook of Industrial Chemistry and Biotechnology, 581–676. https://doi.org/10.1007/978-3-319-52287-6_9
- Sakthivel, S., Neppolian, B., Shankar, M. V., Arabindoo, B., Palanichamy, M., & Murugesan, V. (2003). Solar photocatalytic degradation of azo dye: Comparison of photocatalytic efficiency of ZnO and TiO2. Solar Energy Materials and Solar Cells, 77(1), 65–82. https://doi.org/10.1016/S0927-0248(02)00255-6
- Sardana, S., Gupta, A., Singh, K., Maan, A. S., & Ohlan, A. (2022). Conducting polymer hydrogel based electrode materials for supercapacitor applications. Journal of Energy Storage, 45, 103510. https://doi.org/10.1016/j.est.2021.103510
- Shandilya, P., Mittal, D., Sudhaik, A., Soni, M., Raizada, P., Saini, A. K., & Singh, P. (2019). GdVO4 modified fluorine doped graphene nanosheets as dispersed photocatalyst for mitigation of phenolic compounds in aqueous environment and bacterial disinfection. Separation and Purification Technology, 210, 804–816. https://doi.org/10.1016/j.seppur.2018.08.077
- Shim, K. S., Yang, H. K., Jeong, Y. R., Moon, B. K., Choi, B. C., Jeong, J. H., Bae, J. S., Yi, S. S., & Kim, J. H. (2007). Enhanced luminescent characteristics of laser-ablated GdVO4: Eu3+ thin films by li-doping. Applied Surface Science, 253(19), 8146–8150. https://doi.org/10.1016/j.apsusc.2007.02.078
- Singh, K., Rajendran, M., Devi, R., & Vaidyanathan, S. (2022). Narrow-band red-emitting phosphors with high color purity, trifling thermal and concentration quenching for hybrid white LEDs and Li3Y3BaSr (MoO4) 8: Sm3+, Eu3+-based deep-red LEDs for plant growth applications. Inorganic Chemistry, 61(6), 2768–2782. https://doi.org/10.1021/acs.inorgchem.1c02836
- Song, Y., Shao, B., Feng, Y., Lü, W., Huo, J., Zhao, S., Liu, M., Liu, G., & You, H. (2017). Emission enhancement and color tuning for GdVO4: Ln3+ (Ln= dy, Eu) by surface modification at single wavelength excitation. Inorganic Chemistry, 56(1), 282–291. https://doi.org/10.1021/acs.inorgchem.6b02125
- Sudhamani, S. R., Prasad, M. S., & Udaya Sankar, K. (2003). DSC and FTIR studies on gellan and polyvinyl alcohol (PVA) blend films. Food Hydrocolloids, 17(3), 245–250. https://doi.org/10.1016/S0268-005X(02)00057-7
- Szczeszak, A., Grzyb, T., Śniadecki, Z., Andrzejewska, N., Lis, S., Matczak, M., Nowaczyk, G., Jurga, S., & Idzikowski, B. (2014). Structural, spectroscopic, and magnetic properties of Eu3+-doped GdVO4 nanocrystals synthesized by a hydrothermal method. Inorganic Chemistry, 53(23), 12243–12252. https://doi.org/10.1021/ic500354t
- Tang, S., Huang, M., Wang, J., Fuda, Y., Shang, G., & Jihuai, W. (2012). Hydrothermal synthesis and luminescence properties of GdVO4: Ln3+ (Ln= Eu, sm, dy) phosphors. Journal of Alloys and Compounds, 513, 474–480. https://doi.org/10.1021/ic500354t
- Thakur, H., Kumar Singh, R., & Gathania, A. K. (2021). Synthesis and optical properties of GdVO4: Eu3+ phosphor. Materials Research Express, 8(2), 026201. https://doi.org/10.1088/2053-1591/abe221
- Xu, S., Shi, X.-L., Dargusch, M., Chongan, D., Zou, J., & Chen, Z.-G. (2021). Conducting polymer-based flexible thermoelectric materials and devices: From mechanisms to applications. Progress in Materials Science, 121, 100840. https://doi.org/10.1016/j.pmatsci.2021.100840
- Yadav, S. K., Dhakate, S. R., & Pratap Singh, B. (2022). Carbon nanotube incorporated eucalyptus derived activated carbon-based novel adsorbent for efficient removal of methylene blue and eosin yellow dyes. Bioresource Technology, 344, 126231. https://doi.org/10.1016/j.biortech.2021.126231
- Yi, S. S., Bae, J. S., Shim, K. S., Moon, B. K., Seo, H. J., Jeong, J. H., & Kim, J. H. (2006). Photoluminescence behaviors of Eu-doped GdVO4 thin film phosphors grown by pulsed laser ablation. Journal of Alloys and Compounds, 408, 890–893. https://doi.org/10.1016/j.jallcom.2004.12.107
- Zhu, T., Song, Y., Ji, H., Xu, Y., Song, Y., Xia, J., Yin, S., Li, Y., Xu, H., Zhang, Q., & Li, H. (2015). Synthesis of g-C3N4/Ag3VO4 composites with enhanced photocatalytic activity under visible light irradiation. Chemical Engineering Journal, 271, 96–105. https://doi.org/10.1016/j.cej.2015.02.018
- Zhu, X., Zhang, K., Wang, C., Guan, J., Yuan, X., & Baikun, L. (2016). Quantitative determination and toxicity evaluation of 2, 4-dichlorophenol using poly (Eosin Y)/hydroxylated multi-walled carbon nanotubes modified electrode. Scientific Reports, 6(1), 38657. https://doi.org/10.1038/srep38657