ABSTRACT
High collagenolytic activity has been detected in pathogenic bacteria. Collagenase plays an essential role in the invasion step in animals and humans. In this study, we characterized collagenase found in the nonpathogenic bacterium Lysinibacillus sphaericus VN3, which was isolated from soil in Vietnam. The collagenase activity of the purified enzyme was strongly inhibited by Cu2+, but it was significantly increased by Zn2+. The purified enzyme with a molecular mass of approximately 110 kDa exhibited collagenolytic, gelatinolytic, and caseinolytic activity. The kinetic studies showed that this enzyme had greater hydrolyzing activity toward collagen and gelatin compared with casein. Based on the ratio Vmax/Km, collagen is likely to be the best substrate among three proteins. We found that this collagenase could digest small pieces of bovine skin and tendon into a collagen solution. Interestingly, at pH 6.0–8.0, the soluble collagen could form a collagen membrane, which is useful as a wound-healing biomaterial.
Graphical abstract
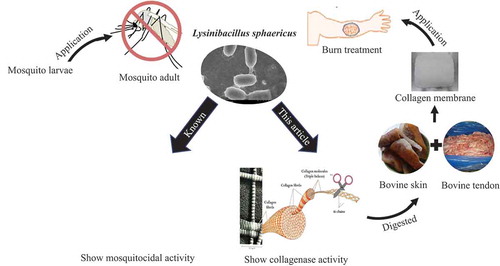
New collagenase found in the soil bacterium Lysinibacillus sphaericus VN3 could form a collagen membrane which is useful as a wound-healing biomaterial.
Bacterial collagenase is an enzyme in pathogenic bacteria that is essential to penetrating the host. The Clostridium sp. collagenase has been extensively studied from 1958 up to the present. The pathogenic role of the clostridial collagenase has been known to damage the cells and help Clostridium sp. to spread in the tissue of the host [Citation1]. Other collagenases of Vibrio parahaemolyticus and Fusobacterium nucleatum [Citation2,Citation3] have been reported as virulence factors for invading and damaging tissue. The collagenolytic activity of nonpathogenic and pathogenic bacteria was detected in Vibrio B-30 ATCC 21,250, V. vulnificus CYK279H và Achromobacter iophagus [Citation4], Pseudomonas aeruginosa [Citation5], Streptomyces lavendulae [Citation6], Bacillus subtilis F2 [Citation7], Enterococcus faecalis [Citation8], V. alginolyticus [Citation9], Streptococcus gordonii [Citation10], Thermoactinomycetes sp [Citation11]., Peptostreptococcus magrisu [Citation12], and other microorganisms such as Bacteroides melaninogenicus, Streptomyces sp., B. cereus MBL13, and Aeromonas veronii [Citation13–Citation16]. However, the collagenase of these microorganisms has not been enzymatically or structurally characterized yet.
In terms of industrial applications, the Clostridium collagenase has been widely used in the medical sector. However, for it to be applied in the food and nutrition sector, nonpathogenic and extracellular collagenase-producing microorganisms are needed to avoid pathogenicity and address safety concerns. Microorganisms involved in the degradation of animal waste/by-products may be able to produce the extracellular collagenase. Marine microorganisms have recently emerged as excellent sources of collagenase.
Since the 1960s, Lysinibacillus sphaericus has been applied for environmental remediation. It is believed that a component of biological pesticides could control the development of mosquitoes, cockroaches, and moths [Citation17–Citation19]. L. sphaericus is a Gram-positive bacterium, which belongs to the Lysinibacillus genus, and is also known as Bacillus sphaericus. It has a rod shape and produces elliptical or spherical colony [Citation19]. L. sphaericus cells do not have metabolized polysaccharides, due to a lack of specific enzymes and transport systems for polysaccharides. However, this bacterium contains abundant proteolytic enzymes, specific transporters, and a metabolic pathway to utilize a series of organic compounds and amino acids. L. sphaericus is known not to contain any metabolic carbohydrate, but the reason for the absence of an energy metabolism has not been elucidated. By 2008, the completed genome sequence of L. sphaericus C3-41 was announced [Citation20,Citation21]. The genome sequence of this bacterium is useful because it provides additional information about the existence of collagenase, which is used for bacterial pathogens in humans and animals. However, to newly develop a bacterial insecticide, the physiological role of collagenase from L sphaericus must be investigated.
The present work focuses on the characterization and application of collagenase from L. sphaericus VN3 (LS collagenase). We examined the various properties of LS collagenase using the purified enzyme. We also attempted to produce a collagen wound membrane from the purified LS collagenase for an in vivo thermal injury model.
Materials and methods
Sample collection, isolation, and screening for collagenolytic bacteria
The soil and wastewater samples used in this study were collected from Nam Son landfill located in Soc Son Commune, Hanoi, Vietnam. A serial dilution was made by taking 1 g of each sample. Then, the sample was diluted up to 10−6 and cultured in the mineral medium ISP9 containing CuSO4.5H2O 0.64 µg/L, FeSO4.7H2O 0.11 µg/L, MnCl2.4H2O 0.79 µg/L, ZnSO47H2O 0.15 µg/L, KH2PO4 2.38 g/L, K2HPO4 5.65 g/L, MgSO4 1 g/L, agar 20 g/L, and (NH4)2SO4 2.64 g/L as the inorganic nitrogen source. Gelatin (Tokyo Chemical Industry, Tokyo, Japan) 10 g/L (pH of 7.5) was used as the organic nitrogen source. Then, the agar plate was incubated at 37°C for 48 h. Bacterial cells, which were able to grow and generated a clear zone around colonies after the addition of 35% (w/v) trichloroacetic acid (TCA), were selected and cultivated on the mineral medium ISP9 containing casein 10 g/L and bovine collagen (Sigma-Aldrich, St. Louis, MO, USA) 5 g/L. For the second screening of extracellular collagenase production, the selected strain was grown on Luria-Bertani (LB) medium containing yeast extract 5 g/L, tryptone 10 g/L, and NaCl LB for 24 h at 37°C. The cultivated medium was centrifuged for 15 min at 8,000 rpm, and the supernatant was used to measure quantitative collagenase, gelatinase, and protease activity on agar medium containing 1% casein, gelatin, and bovine collagen, respectively, and qualitative collagenase activity.
Bacterial identification by morphological and biochemical characterization
The isolated strain cultivated on ISP9 and LB medium-containing casein 10 g/L was tested by microscopic viewing and by the Gram staining technique for the colony and cell morphology identification. The form, size, elevation, border, surface, and color of the selected colonies were observed under a light microscope as described by Bergey’s Manual of Systematic Bacteriology [Citation22]. The isolated strain was tested for the carbohydrate fermentation, catalase, motility, Congo red, methyl-red, H2S production, citrate utilization, and Voges-Proskauer by standard protocol [Citation22].
Bacterial identification by 16S-rDNA
To analyze the genotypic characterization, the selected strain was grown on LB medium at 37°C for 24 h. The cells were collected and used to extract the genome using a QIAamp DNA Mini Kit (Qiagen; Hilden, Germany). The extracted DNA was used as a template to amplify 16S rDNA with primers 27 F (5ʹ-AGA GTT TGA TCC TGG CTC AG-3ʹ) and 1492 R (5ʹ-GGT TAC CTT GTT ACG ACT T-3ʹ). Each 25 µL polymerase chain reaction (PCR) mixture consisted of genomic DNA (50 ng), 1 mL forward and reverse primer (10 mM) and 22 mL premixed solution containing Ex Tag polymerase (Takara Bio, Kyoto, Japan). PCR was performed in the GeneAmp PCR System 9700 (Applied Biosystems, Waltham, MA, USA) with the following cycling conditions: an initial denaturation at 98°C for 2 min, 30 cycles of denaturation at 98°C for 30 sec, annealing at 50°C for 30 sec and extension at 72°C for 1 min. A final extension was performed at 72°C for 10 min. The size of the amplified 16S rDNA analyzed by 1% agarose gel electrophoresis was approximately 1.5 kb. The 16S rDNA product was purified using a QIAquick Gel Extraction Kit (Qiagen). The purified DNA was sequenced by using an ABI Prism BigDyeTM Terminator Cycle Sequencing Ready Reaction Kit (Applied Biosystems) and ABI PrismTM 3730 DNA Sequencer (Applied Biosystems). The obtained 16S rDNA gene sequences were aligned and compared with the known 16S rDNA gene sequences in the GenBank database using Basic Local Alignment Search Tool (BLAST) at the National Center for Biotechnology Information (http://www.ncbi.nlm.nih.gov/BLAST/) to determine the sequence similarity. The obtained gene sequences in this study were finally submitted to Genbank under accession number KX863497 and KX863498.
Purification of collagenase
L. sphaericus VN3 cells were grown in 20 mL of LB medium at 37°C for 12 h. The final preculture was added to 150 mL of mineral medium containing 1% gelatin and incubated at 37°C for 48 h. Cells were removed by centrifugation at 10,000 rpm for 20 min at 4°C. The culture supernatant was added to 55% saturated ammonium sulfate, and the precipitate was collected by centrifugation at 7,000 rpm for 30 min at 4°C. The pellet was dissolved in 50 mL of 50 mM Tris-HCl buffer (pH 7.5), and the sample was dialyzed against the same buffer. The dialyzed solution was purified through two steps of column chromatography, i.e. ion exchange and gel exclusion using dimethylaminoethyl (DEAE) cellulose (Sigma-Aldrich) or Sephadex G-100 (Pharmacia; Uppsala, Sweden) and equilibrated with 50 mmol/L Tris-HCl (pH 7.5), containing 4 mmol/L CaCl2. The column was washed with the same buffer followed by a linear gradient of 0.8 mol/L NaCl at a flow rate of 0.5 mL/min. Active fractions from the Sephadex G-100 column were pooled and concentrated by ultrafiltration.
Sodium dodecyl sulfate (SDS)-polyacrylamide gel electrophoresis (SDS-PAGE)
The proteins were dissolved in a solubilization buffer containing 1% (w/v) SDS, 2.5% (v/v) β-mercaptoethanol, 20% (v/v) glycerol, and 16 mM Tris-HCl (pH 6.8), and were incubated in a boiling water bath for 5 min. Fifteen µL of purified collagenase (1.23 mg/mL) and crude extracts (6.7 mg/mL) were loaded on a 10% SDS-polyacrylamide gels. The gel was stained with 0.5% (w/v) Coomasive Blue R-250 (Sigma-Aldrich), 40% (v/v) methanol, and 10% (v/v) acetic acid.
Gelatin zymography
We analyzed the active form of collagenase by gelatin zymography. A 10% SDS-PAGE gel was copolymerized with 1% gelatin. The sample proteins were mixed with non-reducing 2 x sample buffer [0.625 M Tris, 20% glycerol, 4% SDS, and 0.004% bromophenol blue (pH 6.8)]. Gel was washed two times with 2.5% (v/v) Triton X-100 to remove SDS and then incubated in developing buffer [50 mM Tris, 1 mM CaCl2, and 50 mM NaCl (pH 7.0)] at 37°C for 24 h. After incubation, gels were stained with 0.5% Coomassie Blue R-250 and then destained with 40% methanol and 10% acetic acid. Collagenase activity was detected as clear bands against the stained Coomassie Blue background.
Assay of enzymatic activities
To detect the enzymatic activity of LS collagenase, three substrates were used in this study. Bovine tendon collagen was used as a native collagen to determine the extracellular collagenase activity of L. sphaericus VN3 from culture supernatant and purified enzyme. Moreover, gelatin and azocoll, which are digested by collagenase, were used as denatured collagen and denatured gelatin, respectively.
The collagenase activity was measured by the modified method of Doi et al. [Citation23]. The reaction contained 0.5 mL of 1% (w/v) bovine tendon collagen (Sigma-Aldrich) in 50 mM Tris buffer (pH 7.0), 1 mM CaCl2, and 0.5 mL of supernatant medium or purified enzyme. The reaction mixture was incubated at 37°C for 5 h. The collagenase activity was stopped by adding 100 µL of 0.1 M ethylenediaminetetraacetic acid (EDTA) and kept on ice for 5 min. An aliquot (0.5 mL) of the reaction mixture was withdrawn and 500 µL of ninhydrin reagent was added, and the mixture was then heated at 100°C for 5 min. Finally, the amount of released leucine was measured at 570 nm. One unit of enzyme activity was defined as the amount of enzyme that releases 1 µmol of Leu per min. Protein concentrations were determined using the Bio-Rad Protein Assay Kit (Hercules, CA, USA) with bovine serum albumin (BSA) as the standard protein. To detect the digestion of gelatin according to the method described by Nagano and Kim [Citation24], the reaction mixture that contained 300 μL of 0.2% gelatin (Sigma-Aldrich-9361), 200 µL of 100 mM Tris–HCl (pH7.5) in the presence of 50 mM CaCl2, and 100 µL of the enzyme solution was incubated at 45°C for 30 min. Then, 0.6 mL of 0.01 N HCl was added to stop the enzymatic reaction. The released amino group was quantitated by the ninhydrin method. The caseinolytic activity of the purified enzyme of L. sphaericus VN3 was assayed at 37°C by the method of Chavira et al. [Citation25]. The increase in absorbance at 520 nm was measured in the reaction mixture (final volume, 1 mL) containing 0.5 mL of 0.15% azocoll (Sigma-Aldrich) in 50 mM Tris buffer (pH 7.5) and 0.5 mL of enzyme solution. The reaction was stopped by placing it in chilling ice for 3 min. The unreacted azocoll was removed by spinning at 10,000 rpm for 10 min.
Characterization of collagenase
The effects of pH, temperature, and metal ions on collagenase activity were studied as follows: the dialyzed LS collagenase was used to determine the enzyme activity at various pH levels (4, 5, 6, 7, 8, 9, and 10) and temperatures (4°C, 10°C, 20°C, 30°C, 37°C, and 45°C), and in the presence of 5 mM metal ions (BaSO4, CaCl2, CuSO4, FeCl2, LiCl, MgCl2, MnCl2, PbCl2, and ZnCl2) in the reaction mixture containing 0.5 mL of 1% (w/v) bovine tendon collagen (Sigma-Aldrich) in 50 mM Tris buffer (pH 7.0). The collagenase activity was measured as described above.
Preparation of wound-dressing collagen membrane
Bovine tendon and skin (200 g), after the removal of fat, connective tissue, and hair, were washed with cold 100% (v/v) ethanol and then washed twice with sterilized water. The sterilized bovine tendon and skin were ground into small species and soaked in 200 mL of 20 mM sodium phosphate buffer pH 7.0 and 5 mM ZnSO4. Fifteen micrograms of the purified enzyme were used to hydrolyze this material at 30°C for 48 h. A hydrolyzed collagen solution was incubated at different pH levels from 2.0 to 8.0 to form collagen membrane in a sterilized room. The collagen membrane was observed using scanning electron microscopy.
In vivo test for wound-healing effect by collagen membrane
A total of 12 healthy rabbits were randomly divided into two groups and exposed to heat treatment on their backs at 80°C for 15 sec. The heatstroke area was measured before and after treatment with a collagen membrane. For sterilization, all dressings were placed in 96% (v/v) alcohol solution before treatment with the membrane, and then washed twice with sterilized 0.9% NaCl to remove ethanol. The control group was covered with a dressing containing sterilized 0.9% NaCl. Each layer of the membrane was fixed and protected by a polymer membrane to ensure that the rabbit could not touch it during the treatment. This test was conducted with the formal approval of the local animal care committees in Vietnam Academy of Science and Technology.
Histopathological evaluation
The burned skin (1 cm2) was obtained from the site of injuries at 0, 7, and 21 days after wounding. The samples were washed twice with sterilized 0.9% NaCl and fixed in 10% neutral buffered formalin for 72 h. The tissue specimens were processed with the paraffin technique and stained with hematoxylin and eosin. Epithelialization, inflammatory cell infiltration, fibroblast proliferation, neovascularization, and collagen deposition were observed under a light microscope (Olympus BX51; Olympus, Tokyo, Japan; magnification: x 100).
Results and discussion
Identification of collagenolytic bacteria
On the selective agar medium, the colony of strain VN3 was gray, dry, and crinkly on the surface, and round in shape after 24 h of incubation at 37°C (,)). Under a light microscope, cells were Gram-positive ()), 1.3–1.4 μm in length and 0.7–0.9 μm in diameter, and they had blunt ends, short rods, and mesogenous spores ()). Biochemical tests showed that strain VN3 was negative for indol, citrate, urease, oxidase, hydrogen sulfide, and Voges-Proskauer, but positive for catalase and methyl red (data not shown). This bacterium could not use any carbon sources such as glucose, lactose, sucrose, maltose, mannitol, rhamnose, and trehalose, but utilizes d-alanine, cytosine, l-isoleucine, l-leucine, l-serine, and l-theonine as a nitrogen source (data not shown). Strain VN3 was tentatively identified as L. sphaericus based on its biochemical and physiological characteristics. To confirm this identification, a full 16S rDNA sequence of 1,513 bp in length was amplified and determined (Accession nos. KX863497.1, KX863498.1). The result of 16S rDNA sequence blasting indicated that strain VN3 had a 98% homology with L. sphaericus (Accession no CP014643.1, and the other approximately 22 sequences in GenBank).
Figure 1. Morphology of isolated L. sphaericus VN3. L. sphaericus VN3 cells were spread on mineral agar medium containing 1% casein (a) and 1% gelatin (b) and incubated for 48 h at 30°C. A clear halo was formed around the colony due to hydrolysis of casein. The clear zone of hydrolyzed gelatin was measured after 48 h of incubation with mercuric chloride solution. (c) Gram staining (10 x magnification) and (d) scanning electron microscopy (SEM) (20 x magnification) of L. sphaericus VN3 cells. For scanning electron microscopy, cell suspensions were sputter coated with gold, on plain uncoated filters.
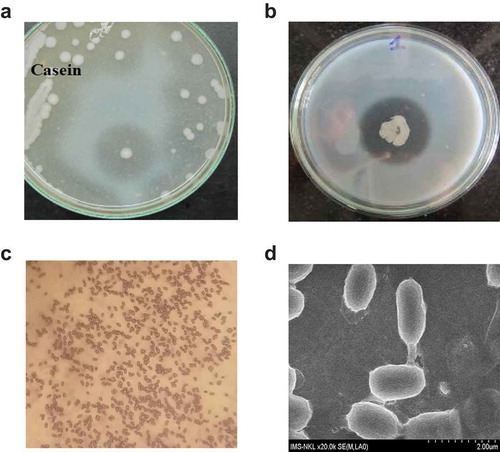
Strain VN3 coincides with the properties of the genus Lysinibacillus in terms of its colony morphology and cultural characteristic. Most of the biochemical and physiological data suggested that strain VN3 is L. sphaericus according to Bergey’s Manual of Systematic Bacteriology, except that it used casein, gelatin, and collagen as the carbon source (,)). Moreover, the blast search for the 16S rDNA gene of strain VN3 in GenBank also confirmed this conclusion. Strain VN3 could grow at pH 5.5–8.5 with optimal growth at pH 7.0, and it stopped growing at pH 5.0. The temperature range extended from 16°C to 45°C and it grew optimally at 37°C. Strain VN3 could not grow at >50°C, and it grew slowly at 16°C. L. sphaericus could not use any sugars due to the lack of genes encoding enzymes and transport systems for the carbon source. Instead, this bacterium utilizes proteins such as casein, gelatin, and collagen. The physiological role of collagenase is to decompose proteins into amino acids for growing L. sphaericus cells. However, the question still remains what kind of collagenase is involved in saprophagy and pathogenesis of L. sphaericus. Bacterial collagenase (EC 3.4.24.3) could be found in both pathogenic and nonpathogenic species. Collagenase is an important factor in the invasiveness and transmission of pathogenic bacteria. In the case of nonpathogenic bacteria, collagenase is an essential enzyme needed to utilize collagen as a source of nutrients. This case is similar to L. sphaericus, which can grow in minimal medium containing collagen. Collagen can be utilized as a carbon and nitrogen sources in the culture medium of L. sphaericus VN3.
Purification of collagenase from L. sphaericus VN3
The supernatant of bulk-cultured L. sphaericus VN3 was concentrated with gradient ammonium sulfate (30% and 75% saturation level), and the precipitate was dissolved in 50 mM Tris-HCl buffer. The solution was then dialyzed against 50 mM Tris-HCl buffer. Subsequently, the dialyzate was applied to a Sephadex G-100 column. The proteins were eluted in two separate peaks. The collagenolytic activity was detected at the first peak. The active fraction was pooled and concentrated by Amicon® Ultra 0.5 mL filters and stored at −20°C. summarizes the results of the enzyme purification. According to a three-step procedure, collagenase from L. sphaericus VN3 (LS collagenase) was purified about 20-fold with a yield of about 25% from the crude extract. On SDS-PAGE, the purified LS collagenase gave a single band corresponding to a molecular mass of approximately 110 kDa ()), although we cannot exclude the possibility that the minor or heterogeneous proteases are coexistent with LS collagenase. However, judging from the hydrolyzed zone formed by the purified enzyme on a zymogram, the molecular mass was estimated to be higher than 135 kDa ()). Most of the pathogenic collagenases from the M9 family have a large molecular mass (90–130 kDa) such as 120 kDa (C. perfringens) [Citation26]. However, nonpathogenic collagenases from the S8 family have been reported to have various molecular mass as follows: 125 kDa (B. subtilis FS-2) [Citation7], 97 kDa and 116 kDa (Streptomyces sp. 3B) [Citation27], 33 kDa and 19.8 kDa (Pseudomonas sp.) [Citation28], 120 kDa and 29 kDa (B. licheniformis N22) [Citation29]. Some of the nonpathogenic collagenases with low molecular mass, such as 50 kDa (Thermoactinomyces sp. 21E) [Citation11] and 20 kDa (Aspergillus oryzae) [Citation30], have not been classified yet.
Table 1. Purification of collagenase from L. sphaericus VN3.
Figure 2. Purification of collagenase from L. sphaericus VN3 by (a) SDS-PAGE and (b) active staining. Fifteen µL of sample was loaded on a 10% SDS-polyacrylamide gel. Molecular mass markers in kilodaltons (Color-coded Prestained Protein Marker, Broad Range #14,208, Cell Signaling Technology, Danvers, MA, USA), dialyzed supernatant after ammonium sulfate precipitation, concentrated DEAE-cellulose fraction, and concentrated Sephadex G-100 fracton are shown from left lane to right lane. The arrow shows the position of LS collagenase.
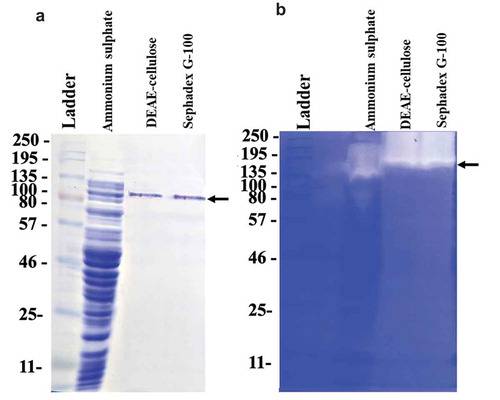
Characteristics of collagenase from L. sphaericus VN3
To characterize the purified LS collagenase, we examined the effects of temperature, pH, metal ions, and reagents on its activity. The optimum temperature and pH for the activity were approximately 37°C and pH 7.0, respectively (,)). The collagenase was not stable from 50°C to 60°C, at which temperature the activity was almost lost. Consequently, this collagenase belongs to the group of neutral proteases. After incubation between pH 6.0 and 8.0, the activity was decreased to 60–70% of full activity. The stability was lower at pH <5.0 or >9.0, and the activity dramatically dropped by over 85%. This is similar to the optimum temperature (30–42°C) reported for C. perfringens [Citation27] and C. historicum [Citation31]. The collagenase was thermally unstable, and its activity was lost more than 70% at 45°C. It is less thermostable than most known collagenases of Bacillus sp. The optimized collagenolytic activity was observed at pH 7.0. The result was similar to most collagenase of Clostridium sp. at pH 7.0–7.5 [Citation31], but lower than those of B. subtilis FS2 [Citation7] and Bacillus sp. MO-1 at pH 9.0 [Citation32], and Thermoactinomyces sp. 21E at pH 9.0–9.5 [Citation11] and A. oryzae [Citation30]. While the pH stability of the collagenase is in the range of pH 6.0–8.0 at 37°C for 30 min, the enzyme retained above 70% of its full activity. This is consistent with the characteristic of the neutral proteinases.
Figure 3. Effect of (a) temperature and (b) pH on activity of collagenase from L. sphaericus VN3. The values are the means and standard deviations of results from three independent experiments.
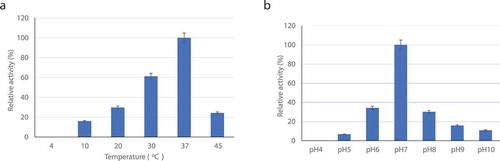
The effect of various metal ions is shown in . Most of the metal ions exhibited either no effect or a slight stimulatory effect. Some of the metal ions, Mg2+ and Zn2+, increased and stabilized the activity. In particular, the activity was greatly increased in the presence of Zn2+. On the other hand, Mn2+ and Pb2+ showed obvious inhibition of the activity. Interestingly, the activity was completely inhibited by Cu2+. Most metal ions including Li+, Mn2+, and Ba2+ did not greatly affect the activity. Ca2+ and Fe2+ resulted in a 20–25% decrease in the activity, whereas collagenases of Streptomyces sp. 3B [Citation27] and Photorhabdus luminescens [Citation33] were almost completely inhibited by Fe2+ and Ca2+, respectively. Interestingly, Zn2+ strongly enhanced its activity, but Mg2+ only slightly enhanced it. These results indicate that LS collagenase is a typical metalloproteinase like many other bacterial enzymes containing an essential Zn2+.
Table 2. Effect of metal ions on collagenase activity.
In bacteria, it is hard to distinguish collagenases from gelatinases or other proteases. However, a bona fide collagenase should degrade native and denatured collagen in correspondence with its collagenolytic and gelatinolytic activity, respectively. Collagenolytic activity can be detected with several substrates such as native collagen, the synthetic peptide 2-furanacryloyl-Leu-Gly-Pro-Ala (FALGPA), gelatin, and casein. In the present study, the purified collagenase showed hydrolyzing activities toward casein (8.33 U/mg enzyme) and gelatin (3.97 U/mg), in addition to collagen (13.97 U/mg). In order to calculate the kinetic constants of LS collagenase, we measured the hydrolytic activity of the purified enzyme toward different concentrations of collagen, gelatin, and casein as substrates (). The apparent Km and Vmax values of the enzyme for collagen and gelatin were significantly higher than those for casein, suggesting that LS collagenase showed a high affinity and high hydrolyzing activity for collagen and gelatin compared with those for casein. Judging from the ratio Vmax/Km, collagen is likely to the best substrate among three proteins tested.
Table 3. Kinetic constants of collagenase from L. sphaericus VN3.
The Clostridium and Vibrio collagenases are the most studied metalloproteinases [Citation34,Citation35]. Clostridium sp. is a pathogenic anaerobe that produces collagenase to invade the host and possibly to degrade host proteins for nutritional purposes [Citation34]. In contrast, the Vibrio collagenase is not an invasive tool for pathogenic bacteria that causes disease in humans. This enzyme is used to break down collagen in fish in order to provide nutrients [Citation35]. The LS collagenase is similar to the Vibrio collagenase that can be induced by the addition of collagen to the medium [Citation36]. The molecular mass of the Clostridium collagenase and the Vibrio collagenase is 116–130 kDa [Citation1,Citation26] and ca. 82 kDa [Citation2], respectively. In contrast, the molecular mass of LS collagenase is 110 kDa, which is similar to those of Clostridium collagenase and the Vibrio collagenase. Both pathogenic and nonpathogenic collagenases fully exhibit collagenolytic, gelatinolytic, and caseinolytic activity, which leads to multiple scissions in the triple helix of collagen due to the collagenolytic activity and complete digestion of these initial fragments into a mixture of small peptides due to the gelatinolytic and caseinolytic activity. The Clostridial collagenase might be used for tissue dissociation and to treat Dupuytren’s disease without surgical operation. On the other hand, the Vibrio collagenase could be applied for the removal of necrotic tissues from burns, ulcers, and decubitus ulcers because of its high and specific activity against native collagen. In the following section, we describe how, in this study, we investigated the ability of LS collagenase to treat burns by creating collagen materials.
Preparation of wound-dressing collagen membrane
To prepare a dried collagen membrane for the evaluation of wound healing, a wet collagen solution is required. A total of 200 g ground tendon and skin mixture was digested with 2,500 U of purified LS collagenase. Previous results showed that this collagenase could hydrolyze native collagen from ground bovine tendon and skin with relative cleavage rates of 50.72% and 62.56%, respectively (). After hydrolysis by an enzyme, the reaction solution was mixed using a homogenous tool. When the pH in the reaction solution was changed, we found that the solubility of hydrolyzed collagen was high at acidic pH levels, but low at alkaline pH levels. This physical property of collagen could affect the structure of the wound membrane.
Figure 4. The bovine tendon and skin before and after digestion with collagenase from L. sphaericus VN3. Pretreatment of small species of bovine tendon and skin. (a) Two-hundred g of ground bovine tendon and skin were soaked in 50 mL of 0.02 M sodium phosphate buffer pH 7 and 5 mM ZnSO4. (b) The insoluble bovine collagen was observed under SEM (5,000 x magnification). (c) Fifteen µg of purified collagenase were used to hydrolyze the materials at 30°C for 48 h. (d) The soluble collagen was observed under SEM (5,000 x magnification).
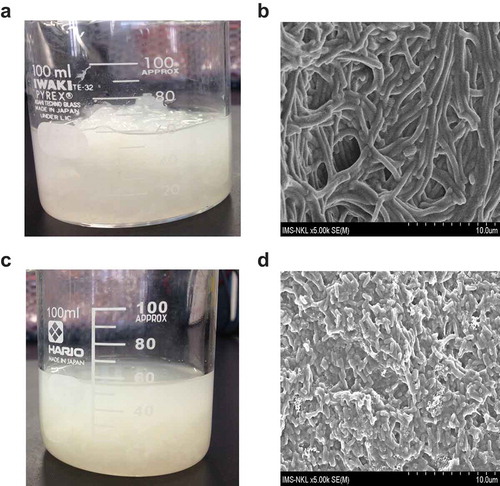
The wet collagen solution was lyophilized at 10% by weight under different pH conditions, and we then evaluated the formation of the membrane. The results showed that at pH 6.0 to 8.0, a collagen membrane could be formed. At pH 7.0, the collagen membrane formed its most homogeneous and toughest surface (,)). The thickness of the collagen membrane obtained was 0.4 mm. This is likely to be a desirable feature when applying LS collagenase to digest bovine skin and tendon into a viscous and soluble solution, such as that used to make wound membrane collagen ()). When the pH of this solution was changed to <5.0 or >8.0, the collagen membrane was difficult to form. Thus, the optimum pH for forming a collagen membrane is 6.0–8.0. The most prominent characteristic of fibrillar collagen is self-assembly at neutral pH, which is suitable for the preparation of enzymatic wound debridement [Citation37,Citation38].
In vivo test for wound-healing effect of collagen membrane
Collagen types I and III of porcine or bovine tissue are the most common types of xenogeneic collagen for wound treatment. Bovine collagen has been reported to exert lower cytotoxicity than porcine collagenase in mononuclear cells [Citation39]. In this study, we used bovine skin and tendon to make the collagen membrane ()), because they contain mainly collagen types I and III. At the initial stage, the healing of wounds in the rabbits in group I and II proceeded similarly. All rabbit models survived and did not show any signs of adverse effects after the healing period. After the heat treatment, the control group was in the phase of inflammation ()). In contrast, the collagen-membrane-treated group exhibited a faster recovery than the control group. The area of burned skin exhibited epidermal restoration in the experimental group ()). The burned skin in the treatment group was regenerated and epithelialized peripherally under the collagen wound dressing. This result could also be observed in the experimental group after three-weeks of treatment ()). After the removal of the collagen wound dressing, the regenerated parts were free of follicles and appeared to be fully covered by the hair; this was due to the residual contracture of the regenerated parts and the proximal approach of the wound edges.
Figure 5. Histological changes during the wound-healing process of rabbit skin. (a) Wound dressing made from soluble and insoluble bovine tendon and skin. (b) Burned rabbit skin after heat treatment at 80°C for 15 sec. Wound-dressing collagen membrane of burned skin (c) after one week and (d) after three weeks. 1: The burned and necrotic skin area. 2: The epithelial layer recovered after burns. The tissue specimens were processed with the paraffin technique, sectioned at 5–7 μm, and stained with hematoxylin and eosin and observed under light microscope at 100 x magnification.
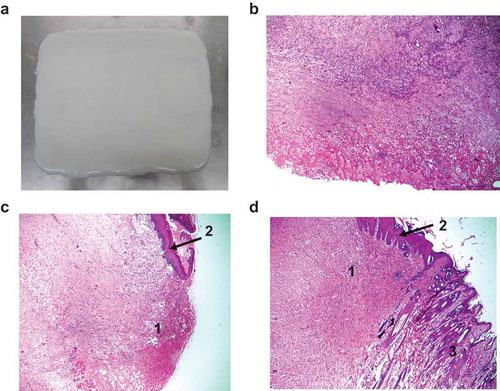
Wound healing proceeds via overlapping phases of inflammation, epidermal restoration, wound contraction, and remodeling. The inflammation can be rapidly resolved to allow the secretion of fibroblasts and keratinocytes, as well as the expression of matrix metalloproteinase, leading to the promotion of re-epithelialization and tissue remodeling [Citation40]. Despite lacking its native triple helix structure, the hydrolyzed collagen membrane has been proved to promote the recruitment of neutrophils and to stabilize the macrophage concentration and inflammatory response so that healing could take place [Citation38].
Author contribution
T.M.H.B. and H. T. conceived the study and designed the experiments. T.M.H.B., T.H.P., and T.S.D. performed the experiments and analysed the data. T.M.H.B. and H. T. wrote the manuscript.
Disclosure statement
No potential conflict of interest was reported by the authors.
Additional information
Funding
References
- Drancourt M, Bollet C, Carlioz A, et al. 16s Ribosomal DNA sequence analysis of a large collection of environmental and clinical unidentifiable bacterial isolates. J Clin Microbiol. 2000;38(10):3623–3630. .
- Yu MS, Lee CY. Expression and characterization of the prtV gene encoding a collagenase from Vibrio parahaemolyticus in Escherichia coli. Microbiol. 1999;145:143–150.
- Uitto VJ, Baillie D, Qiang W, et al. Nucleatum increases collagenase 3 production and migration of epithelial cells. Infect Immun. 2003;73:1171–1179.
- Merkel J, Dreisbach J, Ziegler H. Collagenolytic activity of some marine bacteria. Appl Microbiol. 1975;29(2):145–151.
- Carrick L, Berk R. Purification and partial characterization of a collagenolytic enzyme from Pseudomonas aeruginosa. Biochim Biophys Acta. 1975;391(2):422–434.
- Demina N, Lysenko S. Collagenase from Streptomyces lavendulae. Microbiol. 1996;65:661–664.
- Nagano H, To KA. Purification of collagenase and specificity of its related enzyme from Bacillus subtilis FS-2. Biosci Biotech Biochem. 2000;64(1):181–183.
- Makinen PL, Makinen KK. The Enterococcus faecalis extracellular metalloendopeptidase (EC 3.4.24.30; coccolysin) inactivates human endothelin at bonds involving hydrophobic amino acid residues. Biochem Biophys Res Commun. 1994;200(2):981–985.
- Takeuchi H, Shibano Y, Morihara K, et al. Structural gene and complete amino acid sequence of Vibrio alginolyticus collagenase. Biochem J. 1992;281(3):703–708. .
- Juarez Z, Stinson MW. An extracellular protease of Streptococcus gordonii hydrolyzes type IV collagen and collagen analogues. Infect Immun. 1999;67:271–278.
- Petrova DH, Shishkov SA, Vlahov SS. Novel thermostable serine collagenase from Thermoactinomyces sp.21E: purification and some properties activity. J Basic Microbiol. 2006;46:275–285.
- Krepel CJ, Gohr CM, Edmiston CE Jr, et al. Anaerobic pathogenesis: collagenase production by Peptostreptococcus magnus and its relationship to site of infection. J Infect Dis. 1991;163:1148–1150.
- Hausmann E, Kaufman E. Collagenase activity in a particulate fraction from Bacteroides melaninogenicus. Biochim Biophys Acta. 1969;194:612–615.
- Carey J, Motyl M, Perlman DC. Catheter-related bacteremia to Streptomyces in a patient receiving holistic in fusions. Emerg Infect Dis. 2001;7:1043–1045.
- Liu L, Ma M, Cai Z, et al. Purification and properties of a collagenolytic protease produced by Bacillus cereus MBL13 strain. Food Technol Biotechnol. 2010;48:151–160.
- Welton RL, Woods DR. Pathogenic potential of a collagenase gene from Aeromonas veronii. J General Microbiol. 2008;75:191–196.
- Kellen WR, Clark TB, Lindegren JE, et al. Bacillus sphaericus Neide as a pathogen of mosquitoes. J Invertebr Pathol. 1965;7:442–448.
- Porter AG, Davidson EW, Liu JW. Mosquitocidal toxins of bacilli and their genetic manipulation for effective biological control of mosquitoes. Microbiol Rev. 1993;57:838–886.
- Ahmed I, Yokota A, Yamazoe A, et al. Proposal of Lysinibacillus boronitolerans gen. nov. sp. nov., and transfer of Bacillus fusiformis to Lysinibacillus fusiformis comb. nov. and Bacillus sphaericus to Lysinibacillus sphaericus comb. Int J Syst Evol Microbiol. 2007;57(Pt5):1117–1125. .
- Hu X, Fan W, Han B, et al. Complete genome sequence of the mosquitocidal bacterium Bacillus sphaericus C3-41 and comparison with those of closely related Bacillus species. J Bacteriol. 2008;190(8):2892–2902. .
- Jonge H, Jonge DE, Sima YM, et al. Genome sequence of Lysinibacillus sphaericus strain KCTC 3346T. Genome Announc. 2013;1(4):e00625–13.
- Buchanan RE, Gibbons NE, eds. Bergey’s manual of determinative bacteriology. 8th ed. Baltimore (MD): Williams & Wilkins Co; 1974.
- Doi E, Shibagemta D, Matoba T. Modified colorimetric ninhydrin method for peptidase assay. Anal Biochem. 1954;118:173–184.
- Nagano H, Kim A. Purification of collagenase and specificity of its related enzyme from Bacillus subtilis FS-2. Biosci Biotechnol Biochem. 1999;63:181–183.
- Chvira R, Burnett TJ, Hageman JH. Assaying proteinase with azocoll. Anal Biochem. 1984;136:446–450.
- Matsushita O, Yoshihara K, Katayama S, et al. Purification and characterization of Clostridium perfringens 120-kilodalton collagenase and nucleotide sequence of the corresponding gene. J Bacteriol. 1994;176:149–156.
- Petrova D, Derekova A, Vlahov S. Purification and properties of individual collagenases fromStreptomyces sp. strain 3B. Folia Microbiol (Praha). 2006;51(2):93–98.
- Hisano T, Abe S, Wakashiro M. Isolation and properties of a collagenase with caseinolytic activity from a Pseudomonas sp. J Ferment Bioeng. 1989;68:399–403.
- Asdornnithee S, Akiyama K, Sasaki T, et al. Isolation and characterization of a collagenolytic enzyme from Bacillus licheniformis N22. J Ferment Bioeng. 1994;78:283–287.
- Nordwig A, Jann WF. Collagenolytic enzyme from Aspergillus oryzae: purification and properties. Eur J Biochem. 1968;3:519–529.
- Yang GY, Zhang YZ. Collagenolytic proteases from bacteria. High Technol Lett. 2004;10:35–38.
- Okamoto M, Yonejima Y, Tsujimoto Y, et al. A thermostable collagenolytic protease with a very large molecular mass produced by thermophilic Bacillus sp. strain MO-1. Appl Microbial Biotechnol. 2001;57:103–108. .
- Marokházi J, Kóczán G, Hudecz F, et al. Enzymic characterization with progress curve analysis of a collagen peptidase from an enthomopathogenic bacterium, Photorhabdus luminescens. Biochem J. 2004;379(Pt 3):633–640. .
- Van Wart HE. Clostridum collagenase. 2013;1:607–611. Handb Proteolytic Enzymes.
- Fukushima J, Shimura Y, Okuda K. Vibrio collagenase. Handb Proteolytic Enzymes. 2013;1:604–606.
- Keil-Dlouha V, Misrahi R, Keil B. The induction of collagenase and neutral proteinase by their high molecular weight substrates in Achromobacter iophagus. J Mol Biol. 1976;107:293305.
- Schneider LA, Korber A, Grabbe S. Influence of pH on wound-healing: a new perspective for wound-therapy? Arch Dermatol Res. 2016;298:413–420.
- Eming SA, Krieg T, Davidson JM. Inflammation in wound repair: molecular and cellular mechanisms. J Invest Dermatol. 2007;27:514–525.
- Moura CCC, Soare PBF, Carneiro KF, et al. Cytotoxicity of bovine and porcine collagen membranes in mononuclear cells. Braz Dent J. 2012;23:39–44.
- Elgharably H, Roy S, Khanna S, et al. A modified collagen gel enhances healing outcome in a preclinical swine model of excisional wounds. Wound Repair Regen. 2013;21:473–481.