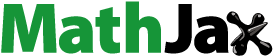
ABSTRACT
The life-limiting fitness for service of high-temperature components is of interest in design, fabrication and later assessments of remaining creep life. Of the associated indicators, strain reflects creep in design and in service, while the discontinuities like creep cavities are targeted in the in-service inspections. Microstructure and hardness can provide supporting information on the material condition. Here, we assess such indicators for the creep-associated damage, particularly at the early stages. Improvements appear possible, e.g. in microscopy to support metallographic inspections and in utilising the widening inspection experience on newer materials. The present work successfully integrated the Wilshire/LCSP creep strain and rupture models with FE analysis for predicting creep strain evolution. Since the model applies for the whole creep curve, it can be used for early stages of creep down to the limit of negligible creep and to the lower limit of the window where creep cavitation damage can be observed.
KEYWORDS:
Introduction
For design, fabrication, initial acceptance, and later assessment of fitness for service, there is recurring interest in the life-limiting material condition of high-temperature pressure equipment [Citation1–3]. Common measures of damage associated with high temperature exposure and creep have characteristic features () in responding to the service conditions and limiting criteria for design, fitness for service and life assessment. Strain is a natural indicator of the associated material condition and degradation in design, numerical analyses and sometimes in measurements, while the status of emerging and growing discontinuities like creep cavities and cracks are in focus of in-service inspections [Citation1–7]. The parallel indications of the microstructure and hardness provide supporting information for example on the initial state and operating temperatures [Citation6,Citation7]. In this paper, we aim to compare these indicator measures and their criteria for anticipated and emerging creep damage, focusing on the early stages of creep life. Examples are provided from an ex-service steam system.
Table 1. Common measures of high temperature damage, effects of service conditions and associated characteristics.
Creep cracking may initiate under creep exposure from pre-existing flaws, stress concentrations or fatigue and/or corrosion related processes [Citation8–12]. In such cases, cracks may develop relatively early in life, but, especially when assisted by fatigue or corrosion, do not purely represent creep driven damage. Otherwise, the damage can appear as stress-driven nucleation and growth of creep cavities, first in apparently scattered pattern and with gradually increasing density, size, coalescence and interlinkage until forming cracks that finally define the fracture surface [Citation13,Citation14]. As we here mainly consider the indicators and criteria for early stages of damage, assessment of such creep crack growth is beyond the present scope. For further guidance on creep cracking, see BS 7910 and R5 [Citation8,Citation9].
Materials and experimental details
To present example features for evolution of the microstructure and creep damage, a section of an ex-service steam header of a power plant was investigated, . The geometry and dimensions of the component were measured by external and internal laser scanning for numerical stress and strain analysis. The header had been in service for 290 000 h at 510°C/60 bar and was made of normalised and tempered 13CrMo4–5 (1Cr-0.5Mo) steel. The header section included a hot formed branch opening for a welded drain branch so that the joint was well outside the saddle points of the branch. As the saddle point regions and welded joints are common locations of long-term creep damage, they were inspected and sampled for microstructural features and damage along the longitudinal section lines crossing the saddle points. The chemical composition (OES) of the header material is presented in , with comparison to the specification. Section samples were ground, polished and etched following standard metallographic practices. The microstructures and indications of creep cavitation damage were assessed with light optical microscopy (LOM, Zeiss Axio Observer) and scanning electron microscopy (SEM, Zeiss Ultra Plus).
Figure 1. a) ex-service section of a steam header with a drain branch (here on top), and b) microstructure near a saddle point region (LOM, nital etch), with no indicated creep cavitation.

Table 2. Chemical composition (m-%) of the example header and its material specification (13CrMo44 [Citation15]).
The mean ± standard deviation of surface hardness was 133 ± 2.5 HV10 below the outer decarburised layer. The microstructure () showed relatively variable grain size of 20 ± 12 µm and abundant inclusions, but no cavities outside the saddle point regions.
Modelling and results
Characterizing creep with early strain information
The use of the ‘curve-family approach’ (CFA), i.e. assessing a range of time to specific strain strengths and establishing a general equation for the creep curve, has been under development in the European Creep Collaborative Committee (ECCC) for a number of years, especially in the support of the CEN TC 54 (WG CREEP) work for determining negligible creep temperature limits [Citation16,Citation17]. In this work, the methodology has been applied on virgin and service exposed 13CrMo4–5 (1Cr-0.5Mo) steel [Citation18] and the EN standard [Citation19] creep and 1% creep strength tables. The method combines the relevant parameters that are generally available when gathering data for creep assessment. In this case, the CFA has been further developed to create a full creep strain model, applicable in the ranges of interest of temperature and stress. The method is based on the logistic creep strain prediction (LCSP) using the time to failure as the limiting end point of the curve [Citation20,Citation21]. The LCSP ‘shape parameters’ are then optimised on the specific times to strain extracted from the raw data creep curves, e.g. 0.2%-10%. The model for time to failure is the Wilshire model [Citation22,Citation23] normalising the creep stress by a factor of the proof stress (at temperature). This approach makes the determination of limiting times and temperatures simple to extract at specific design or allowable stresses. The LCSP model also allows for creating synthetic time to strain data based on reached time-strain values for extrapolating interrupted or running tests without the actual failure time. The assessed raw data Wilshire plots for the curve families used in optimising the LCSP creep strain model are shown in . The equation for proof stress (Rp02), Wilshire model equations (WE) and LCSP model equations are presented in EquationEquations 1(1)
(1) -7. The parameter values for the models are presented in .
Figure 2. a) CFA example using Wilshire type plot for separately assessed strain levels, the time-temperature parameter (TTP) on x-axis and the normalized creep stress (RNu) on y-axis. Note that the 0.2% creep elongation data has large scatter and causes the model line to differ considerably from the 0.5%, 1% and failure assessment lines. The model lines are also merging at the high temperature and long duration end. b) predicted vs. measured time to strain (and failure) for the LCSP model based on true failure times (extended by β). The ECCC defined scatter factor for all levels of time to strain is Z=3.1 (2.5 standard deviations). The time to failure model only has a corresponding value of 3.0, indicating similar behaviour over the whole range of strain.

Table 3. The parameter values for models.
All the data for each pre-defined strain levels are collated and the LCSP is optimised to minimise the root mean error in logarithmic time to strain (EquationEquation 4(4)
(4) ). The LCSP parameters x0 and p are stress (σ) and temperature dependent as given in EquationEquations 5
(5)
(5) -Equation6
(6)
(6) . Note that the stress parameters (x2 and p2) are solved as a function of stress σ and not log(σ) as in the original LCSP definition [Citation21]. The β parameter in here is a constant, set at 1.2% to accommodate for the failure strain not reaching the LCSP model limit value of 100%, i.e. moving the time to failure by a negligible amount to instead have a failure strain in the range of approximately 20%-50%. For long term estimations where considerably reduced creep ductility is expected, the β parameter can be used for further lowering the predicted failure strain. The LCSP model has an additional advantage for generating synthetic data. If one point on the creep curve is known, e.g. time to 0.5% or 1% creep strain, the time to 0.2% can be solved by rearranging EquationEquation 4
(4)
(4) for time to rupture and as the rupture time is equal for the two instances on the curve, the time to strain (synthetic =
) can be solved as given in EquationEquation 7.
(7)
(7)
The LCSP ‘general’ model based full predictive creep strain model is defined by replacing the tu in EquationEquation 4(4)
(4) by the creep rupture model prediction (EquationEquation 3
(3)
(3) ). The resulting model fit plotted against observed time to strain is shown in for the curve family strain levels.
The creep strain development was assessed for cases in the vicinity of the nominal service conditions of the example component to study the sensitivity to stress and temperature.
A finite element analysis (FEA) of the header section by Abaqus® software with LCSP and WE creep subroutines indicated the tensile maximum principal stress of 72 MPa peaking on the outer surface of a saddle point region (), with the corresponding von Mises stress of 59 MPa. The predicted (uniaxial) strains and strain rates at 290 000 h of service at specified stress and temperatures levels are tabulated in and based on creep strength values from [Citation18], the ECCC data sheets [Citation24] and tables of EN 10,028–2 [Citation19], respectively. In all cases, the WE model normalisation was done by proof strength using values from EN 10,028–2. The tables show that at the stress level of 72 MPa the estimated strain, depending on the material strength of the specific heats (or code), can range at 500°C from about 0.7% to about 2.7% and a consumed life fraction from about 28% to about 61%. At the case operating temperature of 510°C, the material data of [Citation18] in would not have allowed the component to achieve its observed time in service. A similar assessment on the relatively high creep strength material heats of NIMS is expected to lower the consumed creep life further.
Table 4. LCSP estimated creep strains and strain rates at 290 000 h under specified stress and temperature values, with a WE failure model fitted on creep data of heats from [Citation18] and normalising proof strength values from EN 10,028–2.
Table 5. LCSP estimated creep strains and strain rates at 290 000 h under specified stress and temperature values, with a WE failure model fitted on ECCC creep data sheet [Citation24] and normalising proof strength values from EN 10,028–2.
Table 6. LCSP estimated creep strains and strain rates at 290 000 h under specified stress and temperature values, with a WE failure model fitted on the creep strength and proof strength values from EN 10,028–2.
Based on the models used above for creep strain evaluation, the negligible- and no-creep temperatures can be determined. The no-creep temperature (TNC) is the temperature below which the creep strain will not exceed 0.2% during the whole service life, e.g. 200 000 h (shorter than the current service time of the component studied). The negligible creep temperature (TNEC) has the same definition of 0.2% creep strain but is time specific, allowing for shorter durations/service lives at temperatures above the TNC. The estimation of the no-creep temperature (at 2/3 Rp, EN 10,028–2) for 290 000 h service is shown in in WE format for three different approaches, i.e. assessment on raw 0.2% data, raw data + synthetic LCSP 0.2% data generated from other time to strains (0.5, 1, 2, 5, 10% and failure, see EquationEquation 7(7)
(7) ), and synthetic LCSP data from standard [Citation19] strength tables only (failure and 1% creep strain). The corresponding study for the case specific 72 MPa (based on standard [Citation19] tables only) is shown in as a TNEC plot (temperature-time).
Figure 3. a) WE plot of actual 0.2% creep strain data, b) extended data set including LCSP determined 0.2% data from raw-data curve family strain levels and c) EN 10,028–2 synthetic LCSP 0.2% creep strain data from standard strength tables.

Figure 4. a) negligible creep temperature curve at 72 MPa, using the EN 10,028–2 creep rupture and 1% creep strength data for generating synthetic time to 0.2% creep strain; the temperature window between reaching the significant creep domain (>0.2% creep strain) and failure is about 45°C. b) FEA-indicated maximum principal stress distribution after 290 000 h on the outer surface of the header, peaking at the saddle point region.

The uncertainty of the mean (2.5 standard deviations) at TNC is largest for the limited 0.2% raw data, followed by the two synthetic data sets, approximately, ±19°C and ± 5°C, respectively. The estimated TNC at 290 000 h of service is from these assessments evaluated to be TNC ~447°C (2/3 Rp = 116 MPa) and for the specific case of 72 MPa stress the TNC is in the range 469–480°C depending on the chosen data in the assessment. The more conservative estimate is from assessing the data from [Citation18]. The analysis indicates that the temperature window between reaching the significant creep domain (>0.2% creep strain) and failure is only about 45°C at this stress level. To verify these numbers an iso-stress test programme is recommended according to the CEN Technical report [Citation17].
Creep cavitation damage
Steels with good creep ductility, or relatively high elongation to failure in creep testing, are usually less prone to creep cavitation. For these steels, such as 10CrMo9–10 and P91, creep cavitation can occur relatively late in creep life, with relatively low preceding cavity density, depending on conditions such as stress state and initial microstructure. For example, tensile multiaxial stress state promotes cavity formation, which should be accounted for if the reference cavity damage at different stages of creep life is defined from observations of uniaxial creep test specimens. In replica inspections or direct metallography, the LOM observations can indicate in addition to creep cavities numerous other features including carbides, sulphides and oxide inclusions, and traces of lost particles. of the example component presents a LOM image where actual grain boundary cavities (with green circles) and other non-cavity features (mostly sulphides and oxides, with red circles) were confirmed by higher resolution SEM imaging and EDS analyses. Confusion between cavities and other indications is most likely when the cavities are small and sparse, in other words with significant residual time to failure (or safe period to next inspection), and the judgement is likely to err to the conservative side.
Figure 5. a) LOM image of creep cavitation damage in 13CrMo4–5 header (base material); red circles show locations of inclusions (not cavities) and green circles locations of genuine creep cavities, confirmed by SEM inspection; b) SEM image of the confirmed cavity number 1 in .

For clear and suitably contrasting metallographic images of microstructures with creep damage, the applied techniques aim to optimise the methods and equipment, for example, in preparation for replica inspections. Recent developments have improved the performance of microscopy for other applications, and mostly for equipment that could not be used for field inspections. Some suggested methods could be exceptions, such as the laser scanning technique described in [Citation6,Citation25], which may provide improved image contrast and reduce false calls unrelated to grain boundary cavities. To assess the condition of material of interest, the classification of creep damage has been defined in some guidelines and standards [Citation26–28]. shows a comparative example of the discrete classification of the VGB and Nordic systems.
Table 7. Creep damage classes in German VGB guideline [Citation27] and corresponding Nordic guidelines [Citation26].
Although creep strain calculations for the example header indicated the component to be near end of its creep life, a gradient in the creep (cavitation) damage exist near the saddle point locations. Because of the component geometry with thinning wall from 50 mm to 23 mm towards the drain branch, there is a gradient in stress and damage. shows the cavity number densities observed from images with equal sizes to VGB-S-517 example images for cavity identification (400×) near the saddle point location of the example component. In this case, the potential identifications were confirmed with SEM investigations to be cavities. Areas with low cavity number density up to VGB class 2b and NT TR 302 class 3b were observed from the investigated samples. The number of smaller (<1 μm diameter) cavities () was larger in the areas with low cavity number density, whereas areas with higher cavity number density included more larger or coalesced cavities ().
Figure 6. Distribution of cavity area fractions and number densities observed from images (400x) near the saddle point location of the example component, in comparison to damage classification of a) NT TR 302 [Citation26,Citation28] and b) VGB-S-517 [Citation27].
![Figure 6. Distribution of cavity area fractions and number densities observed from images (400x) near the saddle point location of the example component, in comparison to damage classification of a) NT TR 302 [Citation26,Citation28] and b) VGB-S-517 [Citation27].](/cms/asset/60b7cba7-6601-4cc0-9687-e945b1f279d5/ymht_a_2295599_f0006_c.jpg)
Hardness and microstructural degradation
Hardness testing is widely used in both fabrication and maintenance of high temperature equipment. Properly calibrated portable equipment can yield reasonably repeatable hardness values at locations of interest to indicate whether the material condition remains within its specified or expected range. Hardness will usually decrease during long-term high temperature service of ferritic steels. The initial value may be poorly known, but the observed values can be compared to values from aged or creep tested materials or component statistics, or to the hardness converted from the tensile strength limits of new material, or to other material or case specific criteria [Citation29]. Although only indirectly indicative of the creep strength at high temperature, hardness is nevertheless one of the few easily accessible indicators of the local material condition and typically highlights the positions of relative weakness in welds and other locations (or materials) with potential deviations in fabrication or maintenance history [Citation30–32]. A caveat is scatter and occasional uncertainties in calibration or other deviations resulting in false alarms. The low cost and wide application of hardness measurements can under adverse conditions disproportionately increase the weight of such misleading indications. Also, less than representative indications can arise when measuring hardness from insufficiently prepared surfaces that were decarburised for example in multiple heat treatments or in long-term service.
Maintenance procedures that apply replica inspections will provide not only information on material integrity such as creep cavitation but also on microstructures and associated degradation [Citation33–35]. Most routine inspections apply light optical microscopy (LOM) that has limited resolution but nevertheless shows the microstructural status on features such as grains, grain boundaries, inclusions, carbides, and other details. The important indications of in-service and other changes include degradation of the eutectoid grain structure and growth of carbides on grain boundaries and within grains [Citation36,Citation37].
Both for the observed reduction in hardness and extent in the microstructural degradation, the scales do not directly indicate absolute criticality because the limiting state is also dependent on loading factors (stress, temperature) of the component and location of interest and on its integrity status. Nevertheless, for maintenance programs the results from hardness and degradation assessments can be used also as compounding risk indicators [Citation36,Citation37]. In general, however, these measures may inform on crossing of warning limits to substandard material condition after fabrication or repair () and hence provide qualitative rather than quantitative measures for in-service life assessment.
Table 8. Approximate warning limits (typical acceptable range) of hardness and microstructure after fabrication or repairs.
Discussion and conclusive remarks
In this paper, the indicators and criteria for expected and emerging creep-associated damage, such as strain, creep cavitation, hardness and microstructural degradation, have been considered with relation to early damage for the potential to assess low life fractions. All these indicators include characteristic challenges and uncertainties, such as metadata availability, accuracy and inconvenience of in-service measurement for strain, and resolution, accuracy and interpretation of the results for microstructure, hardness and cavitation damage. The presented strain assessments for the example component indicate that consumed life fractions could vary from about 50% to failure depending on the data set used to calibrate the models. Regarding the observation and identification of early creep cavitation damage, some false cavity-like features such as off-fallen or removed grain boundary precipitates could be interpreted as actual creep cavities in the usual inspection by light optical microscopy. Although the interpretation is likely to be conservative, it would reduce the potential for extended life or period to next inspection. While guidelines exist for evaluating in-service creep damage [Citation26–28], they have only modest support from common international standards for non-destructive evaluation (NDE) methods [Citation34,Citation35]. As a result, the observed indications of early creep damage are likely to be less consistently observed and interpreted than more extensive damage such as larger cracks detected in conventional NDE. Also, the interpretation of findings from hardness testing and microstructural assessment remain largely outside such standards, although these methods are widely used at least as qualitative input for life or risk assessments.
These indicators and criteria, however, may provide beneficial methods to assess creep damage and its early evolution. The above presented Wilshire/LCSP modelling approach may allow for predicting the rupture relatively accurately from early stages of e.g. 0.2% and 0.5% strain levels, when the material condition fulfils the usual quality requirements. For backup, successful identification of the extent of early creep cavitation damage may provide suitable means to predict creep life with appropriate models, such as the approaches presented in [Citation2] and [Citation38].
Of the potential indicators to help predicting creep life at its early stages, strain is a natural candidate that can show considerable sensitivity to material-specific details and loading conditions. The selected examples of a header component, subjected to FEA and subsequent creep analysis, showed this sensitivity at low strains corresponding to conditions between the actual service history and no-creep and negligible creep temperatures. The experimental scatter that tends to increase towards early stages of creep can be managed for design purposes by using supporting methods such as the ‘curve family approach’ and ‘synthetic’ data from model-based extension of standard materials information. In overall, the raw data and synthetic data assessments, with large extrapolations in time and interpolations in temperature and stress, for estimating strain accumulated creep strain or negligible creep temperatures are naturally sensitive to the data available and models applied. The Wilshire model fit proved to be somewhat sensitive to the used normalisation, generally giving a better fit for normalisation by the tensile strength than for proof strength. There are other rupture models that can produce smaller scatter factors (better fits) than the WE for the same sets of data; however, the benefit of having the possibility to rearrange the same equation for all creep relevant parameters, including temperature and activation energy, has great value for evaluating and testing the extrapolation model. For determination of negligible creep temperatures at a specific ratio of tensile properties, it is especially suitable as the model is based on normalisation by the temperature dependent tensile properties. The same kind of strengths can be claimed for the LCSP, enabling extraction time to strain, strain at time, strain rate at specified time, and the here utilised generation of synthetic time to strain data by only knowing one point on the creep curve.
Regarding strain as an indicator, limits to life fraction and attained strain in operating pipework appear in EN 13,480–3 standard (Annex R), although these limits mostly do not represent early stages of life [Citation39]. Furthermore, measuring local strain (or displacement) of life-limiting locations remains challenging for both early and later stages of creep. This limits the direct application of strain for in-service assessment of the remaining life. However, creep cavitation damage from in-service inspections can serve as a proxy indicator of strain. Because such damage is also driven by processes that will form the final fracture surface, the damage development is related to the remaining creep life, when the loading conditions are within the window of the appropriate type of creep damage. The associated definitions, quantifying and criteria for creep cavitation damage remain less completely standardised than for conventional types of larger defects detected by the usual NDE. Therefore, the extant guidelines and standards on creep damage are likely to be applied with regional and other variation, particularly for materials with limited long-term inspection experience. The resulting conclusions and recommendations after observing a given level of damage will also vary accordingly, perhaps increasingly towards earlier stages of damage. More consistent approach is expected for increasing damage levels including cracking, although with reduced warning time before failure. For example, the recent leaps of improved resolution of microscopy have not materialised as new breakthrough tools for field observation of earlier creep damage. Examples, including low end of the cavity size and density, are given above from an ex-service steam system made of 1Cr-0.5Mo (13CrMo4–5) steel.
Although not directly related to mechanisms of creep (like strain) or creep fracture (like cavitation damage), effects of thermal exposure and positions and levels of local weakness can be indicated by microstructural features and hardness. Such material-specific features can provide information on both the initial condition and its subsequent thermal and other changes to support the assessment of the remaining life fraction.
The combined LCSP and Wilshire models for the creep curve and rupture, implemented to FE analysis of the example header with EN standard materials data, predicted a strain of at least 2% after 290,000 h of service at the saddle point region where significant creep cavitation damage was also observed. It was shown how the same approach can predict the time to given strain from early stages of creep life and contain the scatter due to uncertainties in the materials data even when approaching the limits of negligible creep. This can be done also even with relatively new materials with limited creep and other materials data and with very limited inspection experience.
Figure captions.docx
Download MS Word (13.7 KB)Acknowledgments
The authors wish to acknowledge the CEN/TC 54 WG59 for providing data for the study and Academy of Finland for financial support, via the EARLY project No. 325108.
Disclosure statement
No potential conflict of interest was reported by the author(s).
Supplemental data
Supplemental data for this article can be accessed online at https://doi.org/10.1080/09603409.2023.2295599.
Additional information
Funding
References
- Di Gianfrancesco A. The fossil fuel power plants technology, materials for ultra-supercritical and advanced ultra-supercritical power plants. A. Di Gianfrancesco, ed. London, UK: Elsevier/Woodhead Publishing; 2017. p. 1–47.
- Pohja R, Auerkari P, Vilaça P. Modelling for creep cavitation damage and life of three metallic materials. Mater High Temp. 2022;39(1):86–96. doi: 10.1080/09603409.2021.2024420
- Pohja R, Holmström S, Auerkari P, et al. Predicted life of P91 steel for cyclic high temperature service. Mater High Temp. 2017;34:301–310. doi: 10.1080/09603409.2017.1383710
- Ragab R, Parker J, Li M, et al. Requirements for and challenges in developing improved creep ductility-based constitutive models for tempered martensitic CSEF steels. J Mater Res Technol. 2022;17:3337–3360. doi: 10.1016/j.jmrt.2022.02.047
- Auerkari P, Salonen J, Holmström S, et al. Creep damage and long term life modelling of an X20 steam line component. Eng Fail Anal. 2013;35:508–515. doi: 10.1016/j.engfailanal.2013.05.008
- Siefert J, Parker J. Evaluation of the creep cavitation behaviour in grade 91 steels. Int J Pres Ves Pip. 2016;138:31–44. doi: 10.1016/j.ijpvp.2016.02.018
- Webster G. Trends in high temperature structural integrity assessment. J ASTM Int. 2005;3(2):1–18. doi: 10.1520/JAI13229
- BS 7910. Guide to methods for assessing the acceptability of flaws in metallic structures. The British Standards Institution; 2019. ISBN 978 0 580 52086 0.
- Ainsworth RA. R5 procedures for assessing structural integrity of components under creep and creep–fatigue conditions. Int Mater Rev. 2006;51(2):107–126. doi: 10.1179/174328006X79463
- Shibli A, Le Mat Hamata N. Creep crack growth in P22 and P91 welds — overview from SOTA and HIDA projects. Int J Pres Ves Pip. 2001;78(11–12):785–793. doi: 10.1016/S0308-0161(01)00091-6
- Webster GA, Ainsworth RA. High temperature component life assessment. London, UK: Chapman & Hall; 1994. p. 327.
- Maleki S, Zhang Y, Nikbin K. Prediction of creep crack growth properties of P91 parent and welded steel using remaining failure strain criteria. Eng Fract Mech. 2010;77(15):3035–3042. doi: 10.1016/j.engfracmech.2010.04.022
- Neubauer B, Wedel U, Restlife estimation of creeping components by means of replicas, ASME International Conference on Advances in Life Prediction Methods, editors Woodford DA Whitehead JR, New York/ASME 1983, p. 307–314.
- Riedel H. Fracture at high temperatures. Berlin, Germany: Springer-Verlag; 1987. p. 67–129.
- DIN 17175-1. Seamless tubes of heat-resistant steels; Technical conditions of delivery. Berlin, Germany: DIN; 1959.
- Baylac G, Bullough C, Holmström S, et al. New approaches to determine negligible creep. Mater High Temp. 2022;39(6):668–677. doi: 10.1080/09603409.2022.2135735
- CEN Technical Report. New approaches to determine negligible creep of steels for EN 13445, CEN/TC 54 WG59, submitted to TC54, under review, 2022.
- Ennis PJ, Schuster H, Bendick W. A comparison of the creep rupture behaviour of new and service exposed low alloy steels. Mater High Temp. 1995;13(2):87–92. doi: 10.1080/09603409.1995.11689504
- EN 10028-2:2017. Flat products made of steels for pressure purposes. Part 2: non-alloy and alloy steels with specified elevated temperature properties, CEN; 2017.
- Holmström S, Auerkari P. Robust prediction of full creep curves from minimal data and time to rupture model. Energy Mater. 2006;1(4):249–255. doi: 10.1179/174892406X173594
- Holmström S. Engineering tools for robust creep modeling [ A doctoral thesis]. Aalto University, Laboratory of Engineering Materials; 2010, ISBN: 978-951-38-7379-0.
- Wilshire B, Scharning PJ, Hurst R. A new approach to creep data assessment. Mater Sci Eng A. 2009;510–511:3–6. doi: 10.1016/j.msea.2008.04.125
- Wilshire B, Scharning PJ. Extrapolation of creep life data for 1Cr–0.5Mo steel. Int J Pres Ves Pip. 2008;85(10):739–743. doi: 10.1016/j.ijpvp.2008.04.002
- ECCC DATA SHEETS. Low alloy ferritic steels, steel 13CrMo4-5, WG3.2. EUROPEAN CREEP COLLABORATIVE COMMITTEE (ECCC); 1996.
- Keyence, Laser Scanning Microscopes. [cited 2023 Feb 15]. https://www.keyence.eu/products/microscope/laser-microscope/
- Auerkari P, Salonen J, Borggreen K. Guidelines for evaluating in-service creep damage. Nordtest Report NT TR 302. VTT, Espoo; 1995. p. 15.
- VGB-S-517-00-2014-11. VGB standard guidelines for rating the microstructural composition and creep rupture damage of creep-resistant steel for high-pressure pipelines and boiler components and their weld connections. VGB Powertech, Essen. 71 p + app.
- Program for life assessments of pressure equipment with limited lifetime (in Danish). Danish Working Environment Authority (WEA) guideline B.4.12. 2011, p. 27.
- ISO 18265. Metallic materials - conversion of hardness values. Geneva: ISO; 2013. p. 161.
- Eggeler G, Ramteke A, Coleman M, et al. Analysis of creep in a welded ‘P91’ pressure vessel. Int J Pres Ves Pip. 1994;60(3):237–257. doi: 10.1016/0308-0161(94)90125-2
- Parker J. In-service behavior of creep strength enhanced ferritic steels grade 91 and grade 92 – Part 1 parent metal. Int J Pres Ves Pip. 2013;101:30–36. doi: 10.1016/j.ijpvp.2012.10.001
- Parker J. In-service behaviour of creep strength enhanced ferritic steels grade 91 and grade 92 – Part 2 weld issues. Int J Pres Ves Pip. 2014;114–115:76–87. doi: 10.1016/j.ijpvp.2012.11.004
- Segle P, Tu S, Storesund J, et al. Some issues in life assessment of longitudinal seam welds based on creep tests with cross-weld specimens. Int J Pressure Vessels Piping. 1996;66(1–3):199–222. doi: 10.1016/0308-0161(95)00096-8
- ASTM E1351-01. Standard practice for production and evaluation of field metallographic replicas. West Conshohocken, PA: ASTM International; 2012. p. 6. Reapproved.
- ISO 3057. Non-destructive testing – metallographic replica techniques of surface examination. Geneva: ISO; 1998. p. 2.
- Toft L, Marsden R. Structural processes in creep. Special Report No. 70. London: The Iron and Steel Institute; 1961, p. 276–294.
- Salonen J, Auerkari P, Microstructural degradation of boiler tube steels under long term exposure to high temperature. Report No. 280. Espoo: VTT Manufacturing Technology; 1996.
- Schubert J. Kriech- und Schädigungsverhalten der Mischverbindung G911 gegen P91 bei 600 °C. VDE Seminar. Düsseldorf; 2007 November 30.
- EN 13480-3. 2017 metallic industrial piping. Part 3: design and calculation, Annex R: surveillance of components operating in the creep range. CEN; 2017.