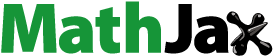
Abstract
The calcitonin gene-related peptide (CGRP) has been demonstrated relating to vascular and inflammatory regulations not only the nerve systems. As the anti-inflammation factor and the most potent vasodilator, the CGRP holds therapeutic potentials for the treatment of cardiovascular diseases which was, however, limited by its peptide nature and short half-life. With advantages in improving the stability, circulation time and protection from degradation, the nanoparticles were promising as delivery carriers for the peptide. Nevertheless, few nanoparticulate systems were developed to deliver the CGRP peptide for the modulation of vascular or inflammatory functions instead of neural regulation. In this study, the CGRP was encapsulated into the poly (lactic-co-glycolic acid) (PLGA) nanoparticle for sustained release of CGRP in vivo. The nanoparticles recovered the systemic level of CGRP and the vascular inflammatory factors in the CGRP+/− rats comparing to the administration of (Dulbecco's Phosphate Buffered Saline) DPBS or peptide only. With the decrease of vascular wall thickness and the attenuation of the T cell infiltration in the lung, the polymer based CGRP delivery system showed potentials to facilitate the therapeutic effects of the CGRP which may help for the development of CGRP-based therapy in vascular and inflammatory disorder related diseases.
Introduction
As a 37 amino acid neuropeptide, the CGRP was mainly secreted by central and peripheral nervous systems and was known playing important roles in neurotransmission [Citation1]. The CGRP has two known isoforms (α-CGRP and β-CGRP) whereas the α-CGRP is the most abundant form (>80%) in the nervous systems [Citation2]. Besides its neural functions, the α-CGRP was also known functioning in vascular and inflammatory regulations since the wide distribution of its receptor including in the endothelial cells [Citation3], smooth muscle cells [Citation4], dendritic cells [Citation5], bone marrow cells [Citation6], macrophages [Citation7] and lymphocyte cells [Citation8]. The CGRP is the most potent endogenous vasodilator identified to date [Citation9]. After stimulated by the CGRP, the endothelial cells activated the eNOS enzyme to release NO via the Gαs-adenylate cyclase-cAMP-PKA pathway and the free diffusion of NO activated the NO/cGMP/PKG signaling pathway in the smooth muscle cells, which led to the relaxation of blood vessels [Citation10]. The CGRP could promote the proliferation of endothelial cells and apoptosis of smooth muscle cells, which lead to the beneficial effects in ischemia and hyperplasia [Citation11–13]. Encapsulated in the chitosan-strontium-calcium phosphate cement, the CGRP could promote the proliferation of endothelial cells in vitro, suggesting the therapeutic potentials to regulate the vascular systems [Citation3].
The CGRP was also considered to be the synthetic product of the immune system, playing a corresponding immunomodulatory role in macrophages [Citation14], neutrophils [Citation15], dendritic cells [Citation16], B lymphocyte [Citation17], T lymphocyte [Citation18], and other immune cells [Citation19]. Having the effects to inhibit the pro-inflammatory factors such as TNF-α, IFN-γ, IL-2, IL-1β or IL-8 and up regulate the IL-10 and IL-4, the CGRP acts as an anti-inflammatory factor [Citation20–22]. After the administration of exogenous CGRP, the inflammatory cytokines such as the TNF-α, IL-1β, IL-8 and IL-6 were decreased and the therapeutic effects were found in the rats with the severe necrotizing pancreatitis [Citation23,Citation24]. Besides, the CGRP can also prevent inflammatory liver injury in mice [Citation25]. All that indicated the CGRP as therapeutics for the treatment of inflammation-related diseases.
Despite the potentials in modulating vascular and inflammatory functions, the short half-life of the CGRP (<10 min) with peptide nature hampered its translational applications [Citation26]. To prolong the half-life, an α-CGRP analogue acylated with an albumin-binding fatty acid moiety allowing reversible albumin binding was developed which showed protective effects against end-organ damage in hypertension, cardiac hypertrophy and heart failure [Citation27]. Nevertheless, the functional mechanism of the albumin-binding α-analogue, e.g. the binding ability to the vascular CGRP receptor, comparing to its native form still needed to be elucidated [Citation28]. Nanoparticulate systems avoid the chemical modification of peptides and keep their natural properties, having been known to improve the stability, half-life and the distribution of drugs in vivo. The polyethylenimine (PEI) linked chitosan was utilized to deliver the plasmid CGRP for fracture healing [Citation23]. A Tat peptide-decorated gelatin-siloxane (Tat-GS) nanoparticle was demonstrated to encapsulate the plasmid DNA of CGRP to attenuate cerebral vasospasm and improve neurological outcomes in a rat model of subarachnoid hemorrhage [Citation29]. However, the nanoparticle-based delivery systems to exert the function of CGRP peptide itself have less been developed.
Therefore, the study aimed to investigate the potential of nanoparticulate system to deliver the CGRP peptide with sustainable release property to prolong the interaction of the peptide with biological surroundings in vivo. Having been approved by the FDA, the poly(lactic-co-glycolic acid) (PLGA) was widely used for the delivery of drugs [Citation30–33]. The polyethylene glycol (PEG) was demonstrated to prevent the nanoparticles from ingestion and clearance by macrophages, prolong circulation time, increase the distribution of diseased tissue and block protein adsorption and cellular influence [Citation34–36]. In this study, using the nanoprecipitation method, a facial fabrication strategy was developed to encapsulate the CGRP in the PLGA modified with PEG for sustained release of the peptide in vivo. To preliminarily demonstrate the efficiency of the delivery system, the nanoparticles were intravenously administered to the CGRP+/− deficient rats and the functions to regulate the immune response and vascular structure were investigated. Using a conventional nanoparticulate system, the study demonstrated the translational potentials of the CGRP peptide as drug for the treatment of cardiovascular or inflammatory related diseases.
Materials and methods
Materials
DPBS was obtained from Hyclone (Logan, Utah, America). PLGA (50:50) (RG502H) and PLGA-b-PEG were from Sigma. 4% polyformaldehyde (PFA) was purchased from the Servicebio (Wuhan, China). α-CGRP was purchased from GL Biochem (Shanghai, China) Ltd. DMSO was obtained from Beyotime Biotechnology (Shanghai, China). Dialysis bags (MWCO: 10000 Da) were purchased from Shanghai Yuanye Bio-Technology Co., Ltd (Shanghai, China). Other chemicals and instruments used were specified in each experiment.
Fabrication of nanoparticles
After dissolving the CGRP with PLGA in the DMSO, the oil phase was dropwise added into the water to form nanoparticles. To optimize the fabrication process, the volume ratio of oil phase to water phase, the weight ratio of CGRP to PLGA and the time of evaporation were adjusted. The final conditions to avoid the formation of aggregates were utilized for following experiments. The PLGA-PEG nanoparticles with or without the CGRP loading were fabricated as the PLGA nanoparticles.
Characterization of nanoparticles
The particle size and polymer dispersity index (PDI) were measured using the dynamic light scattering (DLS) method of the LiteSizer (Anton Paar, Shanghai, China). The zeta potential was measured by diluting NPs appropriately with distilled water, which was then analyzed using the electrophoretic mobility method on the same instrument. To investigate the stability of nanoparticles, the sizes and zeta-potentials of 1 mg/mL nanoparticle solutions were analyzed at indicated time points after the fabrication. Statistical analysis was calculated from 3 duplicate samples.
The morphology of NPs was examined with a FEI 200 kV transmission electron microscopy (TEM, Tecnai G2, America) after negative staining with 1 wt% uranyl acetate Briefly, 5 μL of nanoparticle suspension (10 mg/mL) was deposited onto a 400-mesh carbon-coated copper grid (Beijing Zhongjingkeyi Technology Co., Ltd). Ten minutes after the sample was deposited, the grid was rinsed with 5 drops of distilled water, followed by staining with a drop of 1 wt% uranyl acetate. The grid was subsequently dried and visualized using the FEI 200 kV Sphera microscope.
Loading efficiency and cumulative release
The CGRP loaded nanoparticles were first frozen at −80 °C and then freeze-dried with a lyophilizer. Then, the nanoparticles lyophilized powder was dissolved in DMSO, and the absorbance was measured with Nanodrop 2000 spectrophotometer (Thermo Fisher Scientific, US) at 260 nm. According to the preestablished standard curve of CGRP in DMSO, the drug loading efficiency (LE) and drug encapsulation efficiency (EE) were calculated as follows:
(1)
(1)
(2)
(2)
where MCGRP is the mass of CGRP loaded in the NPs, MPLGA is the mass of polymer in the formulation and Madded is the mass of CGRP added. Three independent replicates were used for each group.
The drug (CGRP) released from PLGA-CGRP (PC) and PLGA-PEG-CGRP (PPC) nanoparticles was studied separately using a dialysis method. Briefly, 1 mL PC and PPC solutions (containing 100 μg CGRP) were added to dialysis bags (MWCO: 10000 Da). The dialysis bags were then immersed in 5 mL of phosphate-buffered saline (PBS) solution (release medium, pH 7.4) at 37 °C on a constant temperature oscillator (SHA-C, Changzhou Guohua electrics co., ltd, China) with shaking speed of 120 rpm. 500 μL of release medium was collected for analysis at different time intervals and replaced with an equivalent volume of fresh PBS at 37 °C. The cumulative amount of the released CGRP was quantified by Nanodrop 2000 spectrophotometer (Thermo Fisher Scientific, US) at 260 nm and statistical results were analyzed from three measurements for each sample.
Treatment of nanoparticles in the CGRP+/− rats
All the animal care and experimental protocols were performed in accordance with protocols reviewed and approved by the Animal Ethical and Experimental Committee of the Fujian Medical University. Animals were housed in rat cages under a temperature-controlled environment (20–22 °C) with 12:12-h light/dark cycles, with ad libitum access to drinking water and standard rat chow. Male Sprague-Dawley wild-type (WT) rats (200–250 g, 8–10 w) were obtained from GemPharmatech Co., Ltd. The α-CGRP knockout (α-CGRP+/−) rat model was generated as described [Citation37]. After the fertilization, the heterozygous α-CGRP ± rats were utilized for animal studies. All animals were weighed and then randomly assigned to receive: DPBS, free CGRP, PLGA-CGRP and PEG-PLGA-CGRP nanoparticles with the dose of 50 nmol/kg of CGRP and injection volume of 500 μL/200 g. After the intravenous administration once a week for four times, the rats were euthanized and the blood and organs were extracted at 1 week post the final injection of nanoparticles.
Hematoxylin-eosin and immunohistochemistry staining
The main organs (heart, lung, liver, kidney, spleen and pancreas) were excised and immersion-fixed in 4% PFA for at least 12 h. Then, the cross-sections were prepared and stained with hematoxylin and eosin (H&E) (G1003, Servicebio, Wuhan, China). To investigate the distribution of T cells in the lung, the CD3 immunohistochemical staining was analyzed. After deparaffinzing, rehydrating, antigen retrieval, blocking endogenous peroxidase activity and serum sealing, the sections were incubated with CD3 primary antibody (1:200 dilution, Abcam, Cambridge, England) and secondary antibody (HRP labeled) (Servicebio, Wuhan, China). All the sections were imaged under an Upright optical microscope (Nikon Eclipse E100, Japan) and Imaging system (Nikon, Nikon DS-U3), following the DAB chromogenic reaction, nucleus counterstaining dehydration and mounting.
Elisa
The concentrations of CGRP, IL-6, IL-8, TNF-α, IL-1β, IL-4, IL-10 and VEGF from the serum and the lung samples were analyzed using the ELISA kits according to the manufacturer’s instructions (Jiangsu Meimian industrial Co., Ltd). Briefly, after the adding of standard, sample and enzyme solutions successively, the plates were incubated for 60 min at 37 °C. After the chromogen solution was added to the well and reacted at 37 °C for 15 minutes, the absorbance of the plate was read at 450 nm within 15 minutes after the termination solution was added.
qPCR
The total RNA of lungs was extracted using the Eastep® Super Total RNA Extraction Kit (Shanghai Promega Biotech Co., Ltd, Beijing China), followed by reverse transcription into cDNA using 5× FastKing-RT SuperMix (TIANGEN Biotech (Beijing) Co., Ltd). Then the gene expression levels were analyzed using the SYBR Green real-time PCR kit (TIANGEN Biotech (Beijing) Co., Ltd) and quantified with the Bio-Rad CFX96 Real time PCR System (Bio-Rad, Hercules, CA). The gene expression levels were normalized to GAPDH values respectively and the relative expression was measured by the comparative CT method. The mean gene expression level was calculated from 3 duplicate samples. The showed the primers used.
Table 1. The sequences of primers used in this study.
Statistical analysis
Statistical comparisons among above three groups were analyzed by one-way ANOVA analysis followed by Tukey’s multiple comparison test. All statistical tests were conducted using GraphPad Prism software. p < .05 was considered to be statistically significant.
Results and discussion
Fabrication and characterization of nanoparticles
The PLGA nanoparticles (P NPs) were obtained via a nanoprecipitation method. After dissolving the CGRP with PLGA in the DMSO, the oil phase was added into the water to form nanoparticles. To optimize the fabrication process, the volume ratio of oil phase to water phase, the weight ratio of CGRP to PLGA and the time of evaporation were adjusted and the increase of these parameters led to the formation of aggregates. At 1:10 volume ratio of oil to water phase and 1:100 weight ratio of CGRP to PLGA, almost no aggregates were found in the solution (Supplemental Table 1) which could be observed and demonstrated by the photo of the final nanoparticle solutions and the distribution width of the diameters (Supplemental Figure 1). The optimized conditions were utilized to fabricate the following P NPs with (P-C NPs) or without the loading of CGRP and the PLGA-PEG nanoparticles (PP NPs) with (PP-C NPs) or without the CGRP loading. After the loading of the CGRP, the UV-VIS spectrum of P-C NPs showed a peak at 260 nm, like the CGRP, while the spectrum of P NPs didn’t show the same peak (Supplemental Figure 2), demonstrating the successful loading of the CGRP in the P-C NPs. The encapsulation efficiency and the loading efficiency of CGRP in the P-C NPs were 41.16 ± 3.90% and 0.41 ± 0.04% respectively. With the PEGylation of PLGA, the encapsulation efficiency and the loading efficiency increased to 60.24 ± 11.76% and 0.60 ± 0.12% respectively. Through the interfacial turbulences occurring at the interfaces of solvent and the non-solvent which resulted from the complex and cumulated process such as flow, diffusion and surface tension variations, the nanoprecipitation offered a rapid and facile strategy to fabricate small nanoparticles [Citation38,Citation39]. Due to the resistance forces between hydrophilic peptide and hydrophobic PLGA molecules, the diffusion of CGRP was very fast when contacted with the water phase which may result in the relatively lower loading efficiency of the peptide comparing to the double emulsion method [Citation40–42]. However, the final loading efficiency, in this study, was comparable to other studies using the nanoprecipitation strategy with loading efficiencies ranging from 0.2% to 0.5% [Citation43–46]. The PEG with hydrophilicity may retard the diffusion of the peptide which may lead to the higher loading efficiency comparing to the PLGA without modification.
Under the TEM imaging, the nanoparticles showed the round shapes which were not changed by the loading of CGRP (). The encapsulation of CGRP increased the hydrodynamic diameter of P NPs from 101 ± 1.6 nm to 150 ± 2.7 nm and the diameter of PP NPs from 122 ± 0.2 nm to 131 ± 1.5 nm (). The PDI below 20% demonstrated the uniform sizes of the nanoparticles (). Having a positive charge (2.7 ± 0.2), the CGRP raised the zeta-potentials of P-C NPs and PP-C NPs comparing to P NPs and PP NPs respectively (). When the nanoparticles were stored in the water, little alterations of diameters ()) or zeta-potentials () occurred, suggesting the stability of the nanoparticles.
Figure 1. (A) TEM images, (B) diameter and (C) zeta-potentials of nanoparticles. Long term stability of the (D) diameter and (E) zeta-potential of nanoparticles in the water. (F) The sustained-release profile of CGRP from P-C NPs and PP-C NPs in the PBS under 37 °C for 120 h. (Mean ± SD, n = 3). (scale bar in magnified image, 100 nm).

As shown in , there was an initial burst release of CGRP during the first 24 h for both P-C NPs and PP-C NPs in the PBS buffer which may result from the CGRP molecules embedding at the outermost layers and absorbing to the surfaces of the nanoparticles. After the first initial burst, the nanoparticles showed the sustained-release pattern and had an overall about 20% and 40% of CGRP release from P-C NPs and PP-C NPs respectively within 120 h. The diffusion of drugs, erosion and swelling of the polymer matrix and degradation of the polymer could all contribute to the release of the drugs from nanoparticles [Citation47]. In this study, the slow degradation rate of PLGA and diffusion of the CGRP may lead to the sustained-release profile of CGRP [Citation48]. It was worthy to mention that the release of CGRP from PP-C NPs was higher and faster than P-C NPs. The PEGylation increased the hydrophilicity of the nanoparticles to allow more water molecules to incorporate into the nanoparticles which may accelerate the degradation of the polymer [Citation49]. Besides, the particle size, porosity and hardness of the PEGylated nanoparticles may also contribute to the release characteristics of CGRP [Citation50].
The regulation of factors in serum by the nanoparticles
To investigate the efficiency of the PLGA loaded CGRP, the nanoparticles were administered to the CGRP+/− rats in which the expression of CGRP was deficient (). After the treatment for one month, the administration of PP-C NPs recovered CGRP in serum of CGRP+/− rats to the level of wild type (WT) rats, comparing to the treatment of DPBS, CGRP peptide only and P-C NPs (). It demonstrated the ability of PEGylated nanoparticles to protect the CGRP from fast elimination and the sustainable release maintained the CGRP concentration in serum at a comparable level to the wild type. To elucidate the effects of the recovery of CGRP level, the pro- and anti-inflammatory factors in serum were analyzed. Interestingly, as shown in , the deficiency of CGRP increased the levels of pro-inflammatory factors (IL-1β and IL-8), anti-inflammatory factors (IL-10 and IL-4) and the vascularization related factor (VEGF) in circulation. The administration of P-C NPs and PP-C NPs showed the tendency to down regulate all of the factors in serum while the treatment of CGRP had lower or no down-regulation effects. For the regulation of IL-8 and VEGF, the P-C NPs and PP-C NPs decreased the levels to the similar extents. However, the reduction of the IL-1β, IL-10 and IL-4 levels in the PP-C NPs group were higher than the P-C NPs. All that suggested the ability of PP-C NPs to recover the CGRP in long-term and regulate the systemic inflammatory factors to the normal level.
Figure 2. (A) Scheme of experiment design to investigate the function of CGRP loaded nanoparticles to modulate inflammatory and vascular factors in CGRP+/− rats. (B) Concentration of the CGRP and other factors in serum post the administration in the CGRP+/− rats comparing to the wild type. (Mean ± SEM, n = 3; *, p < .05; **, p < .01; ***, p < .001).

The inflammatory and vascular modulation in the lung by the nanoparticles
The CGRP was previously found to reduce the thickness of the vascular wall and ameliorate the function of vasculature in the artery injury model or cardiovascular diseases such as hypertension or ischemia [Citation11,Citation13,Citation27,Citation29]. In the CGRP+/− rats, the thickness of the vascular wall in the lung was increased comparing to the wild type () whereas no changes occurred in other organs including the liver, spleen, kidney, heart and pancreas (Supplemental Figure 3). Previous studies have demonstrated the ability of CGRP to induce the pulmonary vasodilation and increase the diameter of pulmonary blood vessels [Citation4,Citation51–53]. In this study, the administration of P-C NPs or PP-C NPs reduced the wall thickness but the CGRP peptide only did not recover the vascular structures, suggesting the superiority of CGRP encapsulated nanoparticles to modulate pulmonary vasculatures comparing to free CGRP peptide (). In addition to the pulmonary vessels, the CGRP has also been demonstrated relating to the immunomodulation in lung tissue [Citation2]. Corresponding to previous study, the study here found the accumulation of T cells around the pulmonary blood vessels in the CGRP+/− rats. Comparing to the treatments of DPBS, CGRP and P-C NPs, the PP-C NPs decreased the T cell number around the vessels to the WT level () which proved the efficiency of PP-C NPs to modulate the pulmonary inflammation.
Figure 3. (A) Hematoxylin-eosin (HE) staining and (B) CD3+ T cell immunohistochemistry staining of the lung sections after different treatments. The outer and inner surfaces of the blood vessels in (A) were outlined by the black dashed lines and the thicknesses of the vascular walls were indicated by the distances from the outer surfaces to the inner surfaces which were marked out by the black full lines.

To further demonstrate the inflammatory and vascular modulation of nanoparticles, the lung tissues were homogenized and the expression of these factors at protein and mRNA level was investigated. The P-C NPs and PP-C NPs increased the CGRP to the WT level while the administration of peptide only did not (). Previous in vitro analysis has shown the diphasic controlled release ability of CGRP loaded nanoparticles despite only 20% and 40% of CGRP released in the P-C NPs and PP-C NPs respectively within 120 h. Actually, the release rate of the peptide in vivo would be accelerated due to the complex environment such as the enzymes, interstitial fluid and local pH [Citation54,Citation55]. The study demonstrated the superiority of nanoparticles to control the release and maintain the high levels of CGRP in vivo which may result in the therapeutic effects of the drug-loaded nanoparticles. Corresponding to the analysis in serum, the administration of PP-C NPs showed the greatest ability to restore the IL-1β, IL-10 and IL-4 proteins to the normal WT level in the lung of the CGRP+/− rats (). The expression of VEGF, which was related to the proliferation of endothelial cells and angiogenesis [Citation56,Citation57], was also up-regulated to the WT level after the administration of P-C NPs and PP-C NPs comparing to the injection of DPBS or peptide only (). It was interesting that the VEGF showed an opposite change in the serum and the lung after the deficiency of CGRP in the DPBS control group comparing to the WT rats which may be related to the complicated roles of VEGF. Previous studies have also reported that, in the lung injury, the serum VEGF was increased to enhance the vascular permeability and infiltration of inflammatory cells but the VEGF in the lung was decreased to induce pulmonary endothelial cells death both of which aggravated the preexisting lung injury [Citation58,Citation59]. The treatment of P-C NPs or PP-C NPs recovered the VEGF to the normal balance in the serum and lung tissue due to the recovery of CGRP level. All that suggested the superiority of nanoparticles comparing to CGRP treatment at the protein level. However, at the mRNA level, the CGRP group showed similar effects with the P-C NPs and PP-C NPs groups (). With the deficiency of CGRP, the mRNA expression of IL-1β, IL-6 and IL-4 were increased while the IL-10 was decreased. After the treatment of CGRP, P-C NPs and PP-C NPs, the mRNA of IL-1β, IL-6 and IL-4 was down-regulated to the WT level. The CGRP was reported to activate the PKA pathway, promote the expression of eNOS and release of NO in endothelial cells, which activated the PKG pathways in the smooth muscle cells and led to the relaxation of blood vessels [Citation12,Citation60,Citation61]. In this study, the administration of CGRP, P-C NPs or PP-C NPs all similarly decreased the mRNA expression of PKA and PKG to the WT level comparing to the DPBS group. The expression of eNOS and VEGF at the mRNA level after the treatment of PP-C NPs were increased comparing to the administration of CGRP and P-C NPs, which may indicate the ability of PP-C NPs to increase the pulmonary vasodilation.
Figure 4. (A) Contents of CGRP and other factors in the lungs after the treatment of nanoparticles in the CGRP+/− rats comparing to wild type rats. (B) mRNA expression of factors in the CGRP+/− rats comparing to wild type rats post the treatments. (Mean ± SEM, n = 3; *, p < .05; **, p < .01, ****, p < .0001).

Conclusion
To sustainably release the CGRP for prolonging the interaction of CGRP with surrounding cells, the PLGA/PLGA-PEG nanoparticles were fabricated via a facial nanoprecipitation method. The nanoparticles containing CGRP showed the reduction of the thickness of vascular wall, decrease of T cell infiltration and adjustment of anti- or pro-inflammatory factors to the WT level in the lung of CGRP+/− rats, demonstrating the potentials of CGRP loaded nanoparticles to modulate the vascular and inflammatory functions in diseases. The study showed the ability of nanoparticles to facilitate the function of CGRP in vivo which may have translational significance for CGRP in vascular and inflammatory disorders related diseases.
Ethics approval
All the animal care and experimental protocols were performed in accordance with protocols reviewed and approved by the Animal Ethical and Experimental Committee of the Fujian Medical University.
Supplemental Material
Download ()Disclosure statement
The authors declare no competing interests.
Additional information
Funding
References
- Edvinsson L, Haanes KA, Warfvinge K, et al. (2018). CGRP as the target of new migraine therapies – successful translation from bench to clinic. Nat Rev Neurol 14:338–50.
- Dakhama A, Larsen GL, Gelfand EW. (2004). Calcitonin gene-related peptide: role in airway homeostasis. Curr Opin Pharmacol 4:215–20.
- Lv T, Liang W, Li L, et al. (2019). Novel calcitonin gene-related peptide/chitosan-strontium-calcium phosphate cement: enhanced proliferation of human umbilical vein endothelial cells in vitro. J Biomed Mater Res B Appl Biomater 107:19–28.
- Springer J, Amadesi S, Trevisani M, et al. (2004). Effects of alpha calcitonin gene-related peptide in human bronchial smooth muscle and pulmonary artery. Regul Pept 118:127–34.
- Carucci JA, Ignatius R, Wei Y, et al. (2000). Calcitonin gene-related peptide decreases expression of HLA-DR and CD86 by human dendritic cells and dampens dendritic cell-driven t cell-proliferative responses via the type I calcitonin gene-related peptide receptor. J Immunol 164:3494–9.
- Mullins MW, Ciallella J, Rangnekar V, et al. (1993). Characterization of a calcitonin gene-related peptide (CGRP) receptor on mouse bone marrow cells. Regul Pept 49:65–72.
- Abello J, Kaiserlian D, Cuber JC, et al. (1991). Characterization of calcitonin gene-related peptide receptors and adenylate cyclase response in the murine macrophage cell line P388 D1. Neuropeptides 19:43–9.
- McGillis JP, Humphreys S, Reid S. (1991). Characterization of functional calcitonin gene-related peptide receptors on rat lymphocytes. J Immunol 147:3482–9.
- Wimalawansa SJ. (1997). Amylin, calcitonin gene-related peptide, calcitonin, and adrenomedullin: a peptide superfamily. Crit Rev Neurobiol 11:167–239.
- Kumar A, Potts JD, DiPette DJ. (2019). Protective role of α-calcitonin gene-related peptide in cardiovascular diseases. Front Physiol 10: 821.
- Wang W, Sun W, Wang X. (2004). Intramuscular gene transfer of CGRP inhibits neointimal hyperplasia after balloon injury in the rat abdominal aorta. Am J Physiol Heart Circulat Physiol 287:H1582–H1589.
- Chattergoon NN, D'Souza FM, Deng W, et al. (2005). Antiproliferative effects of calcitonin gene-related peptide in aortic and pulmonary artery smooth muscle cells. Am J Physiol Lung Cell Mol Physiol 288:L202–L211.
- Zheng S, Li W, Xu M, et al. (2010). Calcitonin gene-related peptide promotes angiogenesis via AMP-activated protein kinase. Am J Physiol Cell Physiol 299:C1485–C1492.
- Duan J-X, Zhou Y, Zhou A-Y, et al. (2017). Calcitonin gene-related peptide exerts anti-inflammatory property through regulating murine macrophages polarization in vitro. Mol Immunol 91: 105–13.
- Pinho-Ribeiro FA, Baddal B, Haarsma R, et al. (2018). Blocking neuronal signaling to immune cells treats streptococcal invasive infection. Cell 173:1083–97.e22.
- Assas BM, Wakid MH, Zakai HA, et al. (2016). Transient receptor potential vanilloid 1 expression and function in splenic dendritic cells: a potential role in immune homeostasis. Immunology 147:292–304.
- McGillis JP, Humphreys S, Rangnekar V, et al. (1993). Modulation of B lymphocyte differentiation by calcitonin gene-related peptide (CGRP). Cell Immunol 150:391–404.
- Xing L, Guo J, Wang X. (2000). Induction and expression of β-calcitonin gene-related peptide in rat T lymphocytes and its significance. J Immunol 165:4359–66.
- Umeda Y, Arisawa M. (1989). Inhibition of natural killer activity by calcitonin gene-related peptide. Immunopharmacol Immunotoxicol 11:309–20.
- Li W-j, Wang T-k, Wang X. (2006). Calcitonin gene-related peptide inhibits interleukin-1β-induced interleukin-8 secretion in human type II alveolar epithelial cells. Acta Pharmacol Sin 27:1340–5.
- Holzmann B. (2013). Antiinflammatory activities of CGRP modulating innate immune responses in health and disease. Curr Protein Pept Sci 14:268–74.
- Holzmann B. (2013). Modulation of immune responses by the neuropeptide CGRP. Amino Acids 45:1–7.
- Li C-L, Qin F, Li R-r, et al. (2019). Preparation and in vivo expression of CS-PEI/pCGRP complex for promoting fracture healing. Inter J Polymer Sci 2019:9432194.
- Schneider L, Hartwig W, Flemming T, et al. (2009). Protective effects and anti-inflammatory pathways of exogenous calcitonin gene-related peptide in severe necrotizing pancreatitis. Pancreatology 9:662–9.
- Kroeger I, Erhardt A, Abt D, et al. (2009). The neuropeptide calcitonin gene-related peptide (CGRP) prevents inflammatory liver injury in mice. J Hepatol 51:342–53.
- Kraenzlin ME, Ch'ng JLC, Mulderry PK, et al. (1985). Infusion of a novel peptide, calcitonin gene-related peptide (CGRP) in man. Pharmacokinetics and effects on gastric acid secretion and on gastrointestinal hormones. Regul Pept 10:189–97.
- Aubdool Aisah A, Thakore P, Argunhan F, et al. (2017). A novel α-calcitonin gene-related peptide analogue protects against end-organ damage in experimental hypertension, cardiac hypertrophy, and heart failure. Circulation 136:367–83.
- Danser AHJ, MaassenVanDenBrink A. (2017). Calcitonin gene-related peptide receptor agonism: a double-edged sword? Circulation 136:384–7.
- Tian XH, Wang ZG, Meng H, et al. (2013). Tat peptide-decorated gelatin-siloxane nanoparticles for delivery of CGRP transgene in treatment of cerebral vasospasm. Int J Nanomedicine 8: 865–76.
- Danhier F, Ansorena E, Silva JM, et al. (2012). PLGA-based nanoparticles: An overview of biomedical applications. J Control Release 161:505–22.
- Mir M, Ahmed N, Rehman A. u. (2017). Recent applications of PLGA based nanostructures in drug delivery. Colloids Surf B Biointerfaces 159: 217–31.
- Swider E, Koshkina O, Tel J, et al. (2018). Customizing poly(lactic-co-glycolic acid) particles for biomedical applications. Acta Biomater 73: 38–51.
- Gao X, Li L, Cai X, et al. (2021). Targeting nanoparticles for diagnosis and therapy of bone tumors: Opportunities and challenges. Biomaterials 265: 120404.
- Pasut G, Veronese FM. (2012). State of the art in PEGylation: the great versatility achieved after forty years of research. J Control Release 161:461–72.
- Suk JS, Xu Q, Kim N, et al. (2016). PEGylation as a strategy for improving nanoparticle-based drug and gene delivery. Adv Drug Deliv Rev 99: 28–51.
- Huckaby JT, Lai SK. (2018). PEGylation for enhancing nanoparticle diffusion in mucus. Adv Drug Deliv Rev 124: 125–39.
- Zhang L, Hoff AO, Wimalawansa SJ, et al. (2001). Arthritic calcitonin/α calcitonin gene-related peptide knockout mice have reduced nociceptive hypersensitivity. Pain 89:265–73.
- Mora-Huertas CE, Fessi H, Elaissari A. (2010). Polymer-based nanocapsules for drug delivery. Int J Pharm 385:113–42.
- Bilati U, Allémann E, Doelker E. (2005). Development of a nanoprecipitation method intended for the entrapment of hydrophilic drugs into nanoparticles. Eur J Pharm Sci 24:67–75.
- Derman S, Mustafaeva ZA, Abamor ES, et al. (2015). Preparation, characterization and immunological evaluation: canine parvovirus synthetic peptide loaded PLGA nanoparticles. J Biomed Sci 22:89.
- Rietscher R, Czaplewska JA, Majdanski TC, et al. (2016). Impact of PEG and PEG-b-PAGE modified PLGA on nanoparticle formation, protein loading and release. Int J Pharm 500:187–95.
- Park MH, Jun HS, Jeon JW, et al. (2018). Preparation and characterization of bee venom-loaded PLGA particles for sustained release. Pharm Dev Technol 23:857–64.
- Pridgen EM, Alexis F, Kuo TT, et al. (2013). Transepithelial Transport of Fc-Targeted Nanoparticles by the Neonatal Fc Receptor for Oral Delivery. Sci Transl Med 5:213ra167.
- Joshi AS, Thakur AK. (2014). Biodegradable delivery system containing a peptide inhibitor of polyglutamine aggregation: a step toward therapeutic development in Huntington's disease. J Pept Sci 20:630–9.
- Kaur S, Bhararia A, Sharma K, et al. (2016). Thyrotropin-releasing hormone loaded and chitosan engineered polymeric nanoparticles: towards effective delivery of neuropeptides. J Nanosci Nanotechnol 16:5324–32.
- Ma W, Chen M, Kaushal S, et al. (2012). PLGA nanoparticle-mediated delivery of tumor antigenic peptides elicits effective immune responses. Int J Nanomedicine 7: 1475–87.
- Keum CG, Noh YW, Baek JS, et al. (2011). Practical preparation procedures for docetaxel-loaded nanoparticles using polylactic acid-co-glycolic acid. Int J Nanomedicine 6: 2225–34.
- Scholes PD, Coombes AGA, Illum L, et al. (1999). Detection and determination of surface levels of poloxamer and PVA surfactant on biodegradable nanospheres using SSIMS and XPS. J Control Release 59:261–278.
- Zhang H, Cui W, Bei J, et al. (2006). Preparation of poly(lactide-co-glycolide-co-caprolactone) nanoparticles and their degradation behaviour in aqueous solution. Polym Degrad Stab 91:1929–1936.
- Astete CE, Sabliov CM. (2006). Synthesis and characterization of PLGA nanoparticles. Journal of Biomaterials Science, Polymer Edition 17:247–289.
- Marshall IAN. (1992). Mechanism of vascular relaxation by the calcitonin gene-related peptidea. Ann NY Acad Sci 657:204–215.
- McCormack DG, Mak JC, Coupe MO, et al. (1989). Calcitonin gene-related peptide vasodilation of human pulmonary vessels. J Appl Physiol (1985) 67:1265–1270.
- McCormack DG, Salonen RO, Barnes PJ. (1989). Effect of sensory neuropeptides on canine bronchial and pulmonary vessels in vitro. Life Sci 45:2405–2412.
- Sandor M, Harris J, Mathiowitz E. (2002). A novel polyethylene depot device for the study of PLGA and P(FASA) microspheres in vitro and in vivo. Biomaterials 23:4413–4423.
- Zolnik BS, Burgess DJ. (2008). Evaluation of in vivo–in vitro release of dexamethasone from PLGA microspheres. J Control Release 127:137–145.
- Ferrara N, Davis-Smyth T. (1997). The biology of vascular endothelial growth factor. Endocr Rev 18:4–25.
- Leung DW, Cachianes G, Kuang WJ, et al. (1989). Vascular endothelial growth factor is a secreted angiogenic mitogen. Science 246:1306–1309.
- Lahm T, Crisostomo PR, Markel TA, et al. (2007). The critical role of vascular endothelial growth factor in pulmonary vascular remodeling after lung injury. Shock 28:4–14.
- Voelkel NF, Vandivier RW, Tuder RM. (2006). Vascular endothelial growth factor in the lung. Am J Physiol Lung Cell Mol Physiol 290:L209–L221.
- Brain SD, Grant AD. (2004). Vascular actions of calcitonin gene-related peptide and adrenomedullin. Physiol Rev 84:903–934.
- Guo Y, Zhang Q, Chen H, et al. (2019). The protective role of calcitonin gene-related peptide (CGRP) in high-glucose-induced oxidative injury in rat aorta endothelial cells. Peptides 121:170121.