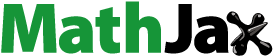
ABSTRACT
Bionanocomposite film is composed of renewable materials such as polymer with the addition of nanofiller in the form of nanocrystal cellulose (NCC). Biopolymer serves as a matrix, while nanofiller is dispersed to improve the functional properties. NCC is a nanoparticle commonly used in the production of bioplastics, and the addition to nanocomposite film can enhance the mechanical and barrier properties. Therefore, this study aimed to determine the physio-chemical, mechanical, and permeability characteristics of elephant foot-yam starch-based nanocomposite films by adding different concentrations of NCC at 3, 5, and 7 wt.%. The experiment used elephant foot-yam starch, NCC, sorbitol, and carboxymethyl cellulose (CMC) as biopolymer, nanofiller, plasticizer, and stabilizer, respectively. The results showed that the optimum concentration was 7 wt.% NCC, showing tensile strength, elongation, WVTR, and thickness consistent with the Japanese Industrial Standard. NCC dispersion showed favorable water solubility and excellent biodegradability, yielding optimal SEM and FTI-R results. SEM analysis showed a well-dispersed structure, while FTI-R showed sharper spectra in specific functional groups. Moreover, XRD showed several diffraction peaks with strong intensity at 2θ = 17.12°(101̅), 22.75° (002), and 35.0° (040), confirming the presence of NCC in the film matrix. Based on the results, this nanocomposite had great potential to be developed into environmentally friendly packaging.
Introduction
Biodegradable plastics are known to naturally decompose in a relatively short time when exposed to certain environmental conditions, such as microorganisms, water, and sunlight.[Citation1] These plastics offer a more environmentally friendly alternative to conventional non-biodegradable ones. One bioplastic material widely used is starch, a carbohydrate glucose polymer composed of amylose and amylopectin. Typically, starch is obtained by extracting plant materials containing carbohydrates, such as cereals and various tubers.[Citation2,Citation3] The unique functional properties allow it to be used in various applications, both as a food and non-food ingredient.
One source that contains a significant amount of starch content is elephant foot-yam tuber. Elephant foot-yam usually grows in yards or fields without special care and is well known among farmers in Java, Sumatra, and Eastern Indonesia. The tuber reportedly contains a starch content of 88.5%[Citation4] and has the potential to be developed as bioplastics.[Citation5,Citation6] According to a previous study, starch is composed of 37.02% amylose and 39.58% amylopectin,[Citation4] both of which play an essential role in making bioplastics matrices. Amylose can increase the mechanical strength and stiffness of bioplastics, while amylopectin has the potential to enhance elasticity and flexibility. In addition, the mixture of both influences the thermal properties and degradation speed.[Citation5]
Studies on the development of bioplastics, particularly in the context of food packaging, have resulted in numerous innovations that have become increasingly beneficial, such as intelligent and active packaging. One common component often added to the innovative packaging is nanoparticles such as zinc oxide (ZnO),[Citation6] NCC,[Citation7] silver nanoparticles,[Citation8] etc. In bio-packaging, nanocomposite usually refers to materials containing 1–7% by weight of a modified nanofiller. The interaction between the matrix and the filler significantly improves when the size of the reinforcing agent is reduced, provided that both phases are compatible and the filler is well dispersed. Therefore, nano-sized particles incorporated into a polymer matrix aim to create a better biopolymer matrix.[Citation9]
Nanocomposite has enhanced properties, including improved strength, lightweight, heat resistance, and antimicrobial characteristics, as well as other physical and mechanical attributes. These properties do not naturally exist in the composite film itself and are achieved through the addition of a nanofiller.[Citation10] The attributes of polymer nanocomposite strongly rely on the uniform dispersion of nanoparticles in polymer matrix and the large interface area generated. A greater interface area between the matrix and filler in the composite leads to enhanced interaction between its components. This impacts the molecular mobility as well as the thermal and mechanical properties of the material. Moreover, nanoparticle amplifies the surface area-to-volume ratio, improving physicochemical characteristics. These factors influence the interaction between the biomaterial and the biological environment, such as hydrophilicity/hydrophobicity, surface energy, etc.[Citation11]
NCC is an example of nanoparticles commonly used in the production of bioplastics. Due to the specific properties, NCC has the potential to be used in security papers, packaging, and composites.[Citation12] The addition of NCC to nanocomposite film can enhance the mechanical properties and heat resistance due to its very strong crystal structure capable of improving the strength and stiffness of bioplastics. NCC has strong mechanical properties; is lightweight, biodegradable, characterized by a large surface area for chemical interactions; and can be integrated with the polymer matrix of bioplastics. The large surface area potentially enhances filler or reinforcement properties, increases density, as well as reduces gas and water vapor permeability.[Citation13]
The inclusion of NCC in nanocomposite film was observed to improve the mechanical, barrier, and thermal characteristics. These improvements mainly arise from the three-dimensional hydrogen bonding interactions between NCC and polymer matrix. Moreover, the crystalline features of NCC and the high aspect ratio enhanced the crystallinity of film, exerting a substantial influence on the physical properties. Mechanical testing showed a significant improvement in the ultimate tensile strength (UTS) of nanocomposite film combined with biopolymer and NCC.[Citation14] The incorporation decreased water absorption, water vapor permeability, and starch solubility by engaging the hydrophilic regions of biopolymer, making it less susceptible to absorbing water. The significant increase in UTS was attributed to the strong bonding adhesion between the filler and the matrix.[Citation15]
Carboxymethyl cellulose (CMC) can also serve as an additive in the production of nanocomposite film to improve the mechanical properties and biodegradability. It functions as a binder or an adhesive between materials in a nanocomposite film mixture, strengthening polymer structure and increasing the viscosity of the blend. This ultimately aids in the formation of a stronger and more durable film. The major reasons for the general use include viscosity, flocculation properties, transparency, nontoxicity, and low price.[Citation16] According to Azwar et al.,[Citation17] CMC has gelation properties, functioning as a thickener, stabilizer, binder, and fine texturizer to improve the mechanical properties of film. The application also increases water vapor resistance and reduces biodegradation rate. Therefore, the addition as a filler has the potential to improve the quality of bioplastics. CMC is a cellulose derivative used as an excellent stabilizer in the food industry and a favorable raw material for packaging.[Citation18] It consists of a sodium carboxymethyl group (CH2COONa), which provides water solubility when added to a cellulose molecule.
Previous studies documented the use of NCC in various film matrices, including those based on elephant foot-yam by Saputra et al.,[Citation19] corn starch by Arifin et al.,[Citation20] avocado seed starch by Fath et al.,[Citation21] and Sago starch by Nasution et al.[Citation22] These studies collectively showed that NCC significantly increased the tensile strength and the resistance of film to water vapor. Meanwhile, as reported by Rahmawati et al.,[Citation23] the use of sorbitol in the matrix increased the elongation and flexibility properties. Azwar et al.[Citation17] also found that CMC demonstrated gelation properties, functioning as a thickener, stabilizer, and binder to refine the mechanical properties of film.
The incorporation of NCC in starch polymer has been extensively explored but the specific use in elephant foot-yam starch from Indonesia is yet to be investigated. Therefore, this study aimed to evaluate the physical, physio-chemical, and mechanical characteristics of elephant foot-yam starch-based nanocomposite film by adding different concentrations of NCC. The results are expected to provide scientific contributions for future studies regarding the use of elephant foot-yam starch which has the potential to become a nanocomposite raw material. Moreover, this study offers insights into the optimal concentration of NCC for its application as a nanofiller in nanocomposite manufacturing. This will help reduce the amount of plastic waste and promote the development of environmentally friendly bioplastics.
Materials and methods
Materials
Elephant foot-yam tuber (Amorphophallus paeoniifolius) was obtained from Brebes, Central Java, Indonesia. Meanwhile, NCC with a size of 471.3 nm (milling with ball mill from Microcrystalline Cellulose Vivapur® MCG 811 F at PRINT-G, Unpad, Indonesia), distilled water, carboxymethyl cellulose, filter paper, PP plastic, silica gel, and sorbitol (182.17 g/mol) were purchased from Bratachem, Bandung, Indonesia.
Starch extraction
Elephant foot-yam starch extraction process referred to Adriansyah[Citation4] with slight modifications. The tuber was sorted, peeled, washed, and cut into pieces manually, then reduced in size using a blender with 1:4 water. Subsequently, filtration was carried out using a filter cloth until all the starch could be extracted. The filtered suspension was left for 24 h and the precipitate was washed with water approximately 2–3× until a clean product was obtained, indicated by clear water conditions. The precipitate was dried in an oven at 50°C for 24 h to obtain water content <13%. The dried starch was ground using a grinder and filtered with an 80 mesh sieve to achieve uniform texture and size. Finally, the starch was placed into a zip lock, and silica gel was added to keep the water content stable.
NCC preparation
NCC with a size of 471.3 nm (Particle Size Analyzer Test) was produced through ball milling designed by PRINT-G laboratory at Universitas Padjadjaran, Bandung, Indonesia. The source material used was Microcrystalline Cellulose Vivapur® MCG 811 F from JRS Pharma, Germany. After the ball milling process, sieving was carried out using a 500 mesh at the Functional Nano Powder University Center of Excellence, Padjadjaran University.
Nanocomposite film preparation
Nanocomposite film preparation referred to a previous study by Arifin et al.,[Citation24] where 7 g elephant foot-yam starch was mixed with 200 ml distilled water to produce a 3.5% (w/v) solution. This starch percentage was determined based on a preliminary study, showing that 3.5% produced a stronger and less brittle film than 3% starch. Referring to Arifin et al., about 5% w/v CMC was added into elephant foot-yam solution gradually while stirring continuously. After CMC dissolved, NCC in different concentrations (3, 5, 7 wt.%) were added. These variations were determined based on previous studies by Saputra et al.,[Citation19] Arifin et al.,[Citation24] Fath et al.,[Citation25] and Nasution et al.,[Citation26] stating the best film was obtained with a concentration between 2 and 7 wt.%. According to preliminary investigation, NCC concentration of 2 wt% produced elephant foot-yam starch film with quite elastic properties but broke easily compared to 3 wt%. The mixture of CMC and NCC was stirred and heated in a beaker glass with a magnetic bar inserted while stirring on a hot plate stirrer. After the gelatinization paste temperature (62°C) was reached, 2% (v/v) sorbitol according to Nasution et al.[Citation26] and Rahmawati et al.[Citation23] was added. Film solution was stirred until 79.5°C, degassed on an ultrasound bath for 15 min, and cooled to an ambient temperature of 40°C. The solution was poured into 20 × 20 cm film plate of 160 ml and finally placed in an oven at 50°C for 20 h.
Nanocomposite film thickness
Measurement of nanocomposite film thickness referred to Arifin et al.[Citation27] and was achieved using a digital screw micrometer with thickness of 0.1 mm. In this study, the Carbon Fiber Composite Digital Thickness Gauge was used with a range: 12.7 mm/0.5,” resolution 0.01 mm/0.0005,” and accuracy: ±0.1 mm/±0.004.” The samples were measured at five distinct points using the screw micrometer. Subsequently, the mean value was computed to ascertain thickness, presented in millimeters (mm).
Nanocomposite film water vapor transmission rate (WVTR)
Water vapor transmission rate (WVTR) of nanocomposite film was measured according to Fath et al.[Citation25] The cup was filled with 10 g silica gel, then the sample was cut based on the diameter of the surface and covered. Subsequently, the cup was placed inside a jar containing a 40% sodium chloride (NaCl) solution (by volume). As water vapor diffused through bioplastic film, it was absorbed by the silica gel, causing an increase in the weight. The test reached equilibrium after approximately 7–8 h, and the weight of film was measured periodically every 1 h. The data obtained was used to create a linear regression equation, which determined WVTR value:
Nanocomposite film water solubility
The solubility of bioplastics was determined according to a previous study by Oluwasina et al.[Citation28] with slight modifications. The water solubility was calculated to ascertain the amount of nanocomposite film components that water could dissolve. The initial weight (wi) with a size of 2 cm × 2 cm was obtained through oven drying at 105°C for 24 h. Film sample was cooled, soaked in 40 mL distilled water for 24 h, stirred, then dried for 24 h at 105°C, and the final weight (wf) was recorded. The solubility of film was calculated using the formula:
Nanocomposite film color
Color testing of nanocomposite film referred to Arifin et al.[Citation27] using a Spectrophotometer (CM-5, Konica Minolta Co., Osaka, Japan) with Software SpectraMagic NX Pro. Ver 2.6 and the L*a*b* scale as a testing tool. The test was carried out by attaching a sensor to film and shooting a beam of light at two different parts with eight distinct points.
Nanocomposite film biodegradability
Biodegradability testing with the soil burial method referred to Hasri et al.[Citation29] with slight modifications. Bioplastics produced were cut into 1 × 1 cm and buried in compost soil at a depth of 6 cm for 12 days. Film degradation was monitored periodically every 3 days. The weight of the sample before (wi) and after burial (wf) was recorded, and the weight loss was calculated using the equation:
Nanocomposite film morphology surface (SEM)
Morphology test was conducted in accordance with Sinaga[Citation30] using a Scanning Electron Microscope with Energy Dispersive X-Ray System of the JEOL brand type JSM-6360LA and serial number MP 18700055. Film sample was cut to 2 × 2 cm, affixed to the set holder, and then gold-plated. Following this, the sample was examined using topographical images at various magnifications.
Nanocomposite film function group (FTI-R)
The function group test was carried out according to Illing[Citation31] and Shafqat et al.[Citation32] using Fourier Transform Infrared Spectroscopy (FTI-R)-Thermo Scientific Nicolet iS5, Thermo Fisher Scientific Inc., Waltham, MA, USA with ASTM E1421 method. The test was conducted by cutting film sample and placing into the set holder, followed by adjustment to the existing spectrum in wavenumber 500–4000 cm−1. FTI-R spectrum was recorded at room temperature using a spectrophotometer, and the data obtained was in the form of a spectrum image between wave number and transmittance.
Mechanical properties
The mechanical properties were evaluated according to the ASTM D882–2002. The Universal Testing Machine, specifically the UCT-5T model manufactured by Orientec Co. Ltd in Japan, was used for this measurement. The machine had a load cell that measured up to 100 kg and the crosshead speed at 50 mm/min. Before testing, film was conditioned in a room maintained at a temperature of 23°C and relative humidity of 50% for a duration exceeding 40 h. Subsequently, tensile strength, elongation, and Young’s modulus were determined in the equation.
where σ = tensile strength (MPa), F = Force applied to the material (N, lb), A = Area (cm2), ɛ = Stretch/Elongation (%), Δl = Change in elongation (cm), L = Initial length (cm), and E = modulus of elasticity (MPa)
Statistical analysis
The experiments were conducted in three repetitions, each with varying concentrations of NCC on nanocomposite film. The results were reported as the mean values and the respective standard deviations (S.D). To analyze the data, a one-way analysis of variance (ANOVA) was used through SPSS 29.0 software (SPSS, Chicago, IL). Additionally, Post hoc analysis was performed using Duncan’s multiple-range test to identify the least significant difference between means at a significance level of 5% (p < 0.05).
Results and discussion
Thickness
shows that the average thickness of nanocomposite film was 0.16–0.22 mm. The lowest value (0.16 mm) was recorded in the treatment without the addition of NCC, while the highest (0.22 mm) was found in the treatment with 7% NCC. As shown in the graph, there was an increasing trend with each increment in NCC addition. The results were consistent with JIS (Japanese Industrial Standard) Z 1707, which recommended 0.25 mm maximum plastic thickness for food packaging.
Figure 1. (a) Nanocomposite film thickness; (b) Nanocomposite film WVTR; (c) Nanocomposite film water solubility; (d) Nanocomposite film biodegradability.

The effect of adding NCC on thickness was reported by Haghighi[Citation33] and Fitriani et al.[Citation34] These studies stated that the use of NCC as a filler material increased the solid content of the resulting film, leading to improved thickness. However, the increase in NCC concentration only had a significant effect at 7%, as the 3% and 5% concentrations showed no significant difference compared to 0%. Similar results were obtained by Coelho et al.[Citation35] which formulated nanocomposite film by adding NCC, with significant changes in thickness observed at concentrations of 5%, 10%, and 15%.
This increase in thickness was due to the higher concentrations of NCC used, which raised the total solid levels. The higher the solution concentration, the thicker the bioplastics formed.[Citation36] The thickness value increased due to the amount of soluble solids present, showing that NCC acted as a suitable matrix filler. NCC, as nanoparticles, has a large surface area and energy to build strong interactions with the matrix and can be well dispersed in the matrix to increase the solution viscosity and make nanocomposite film thicker.[Citation20,Citation34]
Water vapor transmission rate (WVTR)
shows that WVTR value had a decreasing trend with each increment in NCC addition. The highest value of 10.32 was found in the control treatment, while the lowest was obtained at 7% NCC concentration. This showed that the increase in NCC value was directly proportional to WVTR value. The higher the NCC concentration, the better the WVTR value produced. The results met the JIS (Japanese Industrial Standard) Z 1707, which stipulated a maximum WVTR of 10 g/m2.h for food packaging. This was in line with Fath et al.,[Citation25] stating that NCC in the matrix improved water vapor retention and acted as a barrier in film, slowing down water vapor diffusion. At concentrations between 2% and 4% NCC, the most optimum WVTR was recorded, particularly at 4%. Based on the results, the higher the plasticizer concentration, the more optimal the WVTR value.
As shown in , the results showed that the effect of NCC addition to WVTR value was in the order 0%>3%>5%>7%, respectively. Arifin et al.[Citation20] mentioned that the increase in NCC concentration decreased WVTR value. This phenomenon occurred because the nanometer scale restricted water movement in film matrix, creating elongated entanglement paths. In the case of WVTR with sorbitol plasticizer, it was observed that increasing NCC concentration resulted in lower values. This could be attributed to the fact that the higher the concentration, the more tortuosity structures were formed in film. In addition, hydrogen bonds were formed between NCC and the starch matrix of film, preventing the formation of pores as a pathway for water vapor.
According to Idris et al.,[Citation37] the tortuosity structure in bioplastic film refers to the degree of entanglement, twisting, or turning of the pathway through which water vapor or other gases must pass through. The more complicated and tortuous the pathways, the greater the difficulty for water vapor to move freely and cross the material quickly. Therefore, the formation of higher tortuosity structures in bioplastic film can inhibit water vapor transmission and result in lower WVTR values. Several factors influence this structure in formulating bioplastics.[Citation37,Citation38]
NCC can form a tortuous structure in film matrix due to its high aspect ratio. According to George and Sabapathi, this material has a length significantly greater than the width, facilitating the formation of a fiber network which enhances the tortuous structure. NCC also has a large surface area, enabling effective interaction with the matrix and the formation of strong intermolecular bonds. This property enables NCC to create a tortuous structure, improving water vapor transmission resistance. The high aspect ratio and surface area lengthen the path that water vapor or other gases must transverse in the matrix, effectively reducing the water vapor transmission rate.[Citation39,Citation40]
Water solubility
As shown in , the results obtained from the solubility test ranged from 33% to 47% with a decreasing trend along with the addition of NCC concentration. The highest percentage value was obtained in the control, while the lowest value was recorded in the 7% concentration. This showed that the addition of NCC affected the solubility of nanocomposite film in water.
According to the Duncan’s Multiple Range Test by Nasution et al.,[Citation22] the addition of NCC caused a decrease in water uptake. This was associated with the formation of cellulose nanoparticles that prevented starch swelling and water uptake. As stated by Santana,[Citation41] the high solubility value of nanocomposite film without NCC was because starch-based bioplastics were more soluble. Starch readily absorbs water due to the hydroxyl molecules, making it easier for bioplastics to dissolve.
Saiful et al.[Citation42] stated that the high amount of water absorbed might be due to the highly polar nature of water and plasticizers. Water absorption could also be related to the chemical structure of materials with functional groups (OH). Additionally, sorbitol, considered a hydrophilic material was found to bind water, resulting in softer bioplastic surfaces and reduced tensile strength. Bioplastics with sorbitol plasticizers tend to be more porous and degrade easily.[Citation43]
The decrease in water solubility was mainly due to the formation of strong hydrogen bonds between NCC and film matrix. According to Abdollahi et al., hydroxyl groups on NCC form strong interactions through hydrogen bonds with hydroxyl and carboxyl groups, thereby increasing the density of biopolymer matrix and reducing the sensitivity to water.[Citation44] This study found that at concentrations between 0% and 10% NCC, the solubility in water decreased with each increment.
The water solubility value was used to test water resistance and as an indicator of biodegradability in nanocomposite film. Specifically, higher water solubility showed greater biodegradability and an increased capacity for film to decompose easily. This relationship arises from the fact that water provides an ideal environment for the growth of bacteria and microbes. Consequently, a higher water content makes bioplastics more susceptible to degradation. The hydrophilic surface of the plastic further facilitates the attachment of degrading bacteria, enabling the formation of biofilms. These biofilms play a crucial role in breaking down complex polymer into simpler components, thereby accelerating the degradation process.[Citation45]
Biodegradability
Biodegradability was assessed with soil burial method, using andisol soil type. Given that Indonesia has high rainfall, soil has a significantly high water content.[Citation46] The test showed that biodegradability value of elephant foot-yam starch-based nanocomposite film had a percentage between 22.57% and 39.03% with a decreasing trend (). The higher the concentration of NCC added, the lower the percentage value of biodegradability. This trend was consistent with nanocomposite solubility. The relationship between the percentage value of nanocomposite solubility in water and biodegradability was directly proportional. This was because, aside from microbes, the moisture content of soil played a crucial role in degrading nanocomposite film.
The results showed that increasing NCC concentration reduced biodegradability value, consistent with reports from previous studies. This was because only nanocomposite film added with NCC degraded in natural environmental conditions within more long timeframe from the control. This phenomenon can be attributed to the highly crystalline nature of pure NCC, which tightly binds its macromolecular chains. Consequently, water molecules and bacterial cells encounter difficulty in accessing the internal polymer network for hydrolysis or degradation, leading to a delay in the initiation of biodegradation.[Citation47]
Starch-based bioplastics degrade in soil and composting, while anaerobic and aquatic environmental conditions are unsuitable. Therefore, the different biodegradation rates could be due to various factors, including the nature of biopolymer (chemical and physical structure), as well as the environmental conditions to which it was exposed (such as temperature, humidity, pH, sunlight, nutrient and oxygen availability, and the microbial community involved), and the duration of the test.[Citation48]
According to Arief,[Citation45] the length of time for biodegradation of material was influenced by polymer structure. Cellulose bioplastics are easily degraded compared to conventional plastics due to the hydroxyl (OH) and carbonyl (CO) groups. The biodegradation process occurs when the β-1,4-glycosidic bonds in cellulose undergo bond-breaking reactions, gradually breaking the molecules into glucose. Due to the chemical degradation reactions in linear polymer, the molecular weight decreases, and the chain length shortens. Consequently, the weight of the buried nanocomposite film reduced as the burial period extended.
The addition of plasticizers also played a role in the biodegradation of the sample primarily due to enhanced water absorption capacity resulting from the affinity of plasticizers to water.[Citation32] Furthermore, the inclusion of CMC influenced biodegradability. CMC is an intrinsically biodegradable polysaccharide anticipated to degrade completely in environmental systems such as soil.[Citation49]
Color
The L* color value in bioplastics served as a measure for the brightness of the sample and ranged from 0 to 100. The chromaticity coordinates L*, a*, and b* values were part of the CIELab* colorimetric systems and could be used to collect the total color difference.[Citation50] shows that the highest L* value (96.89) was recorded in the control, and the lowest (96.66) was in the treatment with 3% NCC. This difference was influenced by the value of the composition in the manufacturing process. The formula used to manufacture bioplastics in the control treatment was white elephant foot-yam starch, clear sorbitol plasticizer, and slightly yellowish-white CMC. Photos of the nanocomposite films are presented in .
Figure 2. (a) Nanocomposite film color L*; (b) Nanocomposite film color a*; (c) Nanocomposite film color b*.

Figure 3. (a) 0% NCC nanocomposite film; (b) 3% NCC nanocomposite film; (c) 5% NCC nanocomposite film; (d) 7% NCC nanocomposite film.

According to Li et al.,[Citation50] the color value a* in bioplastics measures the redness or greenness of the sample. In this particular nanocomposite film, the a* value remained below 1, except the control. The highest value was in the control, meaning that all nanocomposite film samples had almost no redness and greenness.
The b* value measures the yellowish and bluish color of the sample. In this nanocomposite film, the b* value remained below 2. The highest was obtained in the 3% NCC treatment, possibly due to the slightly yellowish tint color in CMC used. The color of film could affect its visual appeal and suitability for various applications. Several factors, including the type of bioplastic,[Citation51] starch type and concentration,[Citation41] process conditions,[Citation52] and chroma coordinates[Citation53] could influence the color of film.
SEM
Scanning electron microscope (SEM) is a commonly used method to scan the microstructure and morphology of materials. During the procedure, a low-energy electron beam is emitted into the material and scans the surface of the sample. Several different interactions occur when the beam reaches and enters the material, leading to the emission of photons and electrons from or near the sample surface.[Citation54]
The surface morphology of bioplastics is influenced by various factors, including the production method, which can be affected by the type of processing, temperature, and pressure. Moreover, the use of starch as biopolymer[Citation55] and the addition of NCC filler affect the surface morphology of bioplastics.[Citation56]
SEM results showed that the starch granules in the control appeared hollow, while in the 7% NCC treatment, the filler in nanocomposite film matrix was dispersed on the surface (). The result was in line with Arifin et al.,[Citation24] suggesting that NCC contributed to the formation of a strong network through hydrogen bonds, both within and between molecules, as well as mutual bonding with the starch matrix and plasticizer. NCC filler appeared to fill empty spaces in the matrix, causing film surface to become denser and filled. The density of film was due to good adhesion between the filler and polymer matrix, as well as hydrogen bonding interactions between the hydroxyl groups on each component. According to Agustin et al.,[Citation56] the presence of white spots was caused by agglomeration between NCC and non-plasticized starch. The homogeneous dispersion of NCC in the starch matrix could be ascribed to the relatively small crystal size and strong H-bonding interaction between cellulose and starch.
FTIR
FTIR is an analytical technique to identify organic material and polymer with longer wavelengths and lower frequencies than visible light. The analysis uses infrared light to scan the test sample and observe its chemical properties. shows FTIR results of 0% (A) and 7% NCC treatment samples (D). During the processing and drying of the starch matrix, plasticizer, and NCC, hydrogen bonds were formed due to interactions between the hydroxyl groups in the components. The bonds among these three components created a strong and rigid network in film derived from the matrix. These interactions contributed to the stability and durability of the resulting starch-plasticizer-NCC film.[Citation20] NCC is a cellulose derivative containing hydroxyl functional groups (−OH), contributing to its reactivity and ability to form hydrogen bonds with other molecules. This characteristic allows for various functionalization through chemical reactions, leading to the development of different materials.[Citation57]
The results showed that the addition of NCC fillers affected FTIR spectrum of bioplastics due to the interaction with polymer matrix. The type of polymer used to produce bioplastics could affect FTIR spectrum. The slightly sharper absorption peak at OH bond was due to the hydrogen interaction when the starch and NCC components were mixed in nanocomposite manufacturing process. The hydrogen bonds consisted of interactions between the amylose chain with amylose, amylose with amylopectin, as well as amylose with NCC and amylopectin.[Citation26]
Muhammad et al.[Citation58] obtained similar results reporting that all spectra of nanocomposite film had several main peaks, including OH, CH, and OH (water bending). Several significant shifts in the wavenumber of functional groups were observed when NCC was added to nanocomposite film. These shifts could be attributed to the reduction in molecular mass, as vibration frequency was inversely proportional to the molecular mass undergoing vibration. According to Arifin et al.,[Citation20] the shift in absorption bands in FTI-R infrared spectrum indicated the occurrence of new interactions between starch components, plasticizers, and NCC ultimately impacting the surface properties and characteristics of film.
Mechanical properties
The performance of bioplastics was evaluated through mechanical properties such as tensile strength, elongation at break, and modulus of elasticity, or Young’s Modulus. Tensile strength refers to the maximum stress a material can withstand when stretched or pulled before breaking and is measured in megapascals (MPa). Meanwhile, elongation at break is defined as the percentage of deformation a material can undergo before breaking. The modulus of elasticity, also known as Young’s modulus, measures the stiffness or resistance of a material to deformation under stress. These properties are influenced by various factors, such as the starch concentration, additives, and plasticizers used.[Citation7,Citation59]
Based on , the graph showed an increasing trend in tensile strength with each increment in NCC addition. The tensile strength in all treatments was consistent with JIS Z 1707, which specified a maximum value of 0.392 MPa for plastics used in food packaging. As for the elastic modulus, the graph showed an increasing trend in each NCC addition. The graph representing elongation at break demonstrated a characteristic pattern, fluctuating to a concentration of 5%. The elongation value decreased with higher NCC concentration, but at 7%, an increase in the value was observed. However, the value obtained met JIS Z 1707, which stipulated a maximum percentage of 70% for plastics used in food packaging.
Figure 6. (a) Nanocomposite film tensile strength; (b) Nanocomposite film elastic modulus; (c) Nanocomposite film elongation at break.

The graph showed that the tensile strength increased linearly with higher NCC concentration. Theoretically, three main factors could affect the mechanical characteristics of nanocomposite materials: (1) nanofiller dimensions and morphology, (2) processing method, as well as (3) micro/nanostructure of the matrix and matrix/filler interface. Nanofillers with a high aspect ratio have a larger specific surface area, providing a better reinforcement effect. In this context, NCC contributed to forming strong bonds in the material mixture during the curing process for nanocomposite film. The addition of NCC with a larger shape and surface area, along with a reduction in the particle size, enhanced the bond between the nanofiber and the main material, ultimately improving mechanical performance.[Citation60]
Elephant foot-yam starch, containing 24.8% amylose, can be used as a substitute material for producing nanocomposite film. The tensile strength and elastic modulus increased when elephant foot-yam starch was added. However, its impact on the elongation at break decreased. This occurred due to the stiffening effect resulting from starch on nanocomposite film matrix.[Citation19] The modulus and tensile strength increased with each increment in NCC concentration, indicating that NCC was homogeneously dispersed in biopolymer. This uniform distribution was attributed to the formation of hydrogen bonds in nanocomposite film. El Achaby et al.[Citation61] stated that sorbitol as a plasticizer in nanocomposite systems could help improve dispersion and interaction with NCC. Based on the results, each increment in NCC concentration from 1% to 8% caused a linear increase in tensile strength. The results showed that Young’s modulus increased linearly up to a concentration of 5%, but at 8%, a gradual decline was observed. This implied that the highest peak of Young’s Modulus was at NCC concentration of 5%.
The elongation value decreased at 3% and 5% NCC concentration but increased at 7%. The decrease was attributed to the reduced ability of nanocomposite film to stretch. Ilyas et al.[Citation60] also mentioned that the increase in NCC concentration reduced the movement of molecules in bioplastic material, thereby making nanocomposite more rigid. Consequently, nanocomposite film containing NCC became more break-resistant, stiffer, and less stretchable compared to those without NCC. It was concluded that the elongation value was reduced due to a rigid nanofiller structure.
The results also showed that the addition of plasticizer, in this case, sorbitol was directly proportional to the percentage of elongation. Plasticizers can reduce brittleness and increase the flexibility of polymer film by breaking hydrogen bonds between adjacent molecules.[Citation62] The addition of sorbitol in the manufacture of bioplastics also affects cellulose, reduces intermolecular interactions, and increases polymer mobility. The effect was attributed to a reduction in hydrogen bonds, thereby increasing flexibility and causing a decrease in tensile strength.[Citation45]
Despite the general trend of decreasing elongation percentage, an increase was observed when 7% NCC was added. This effect might be attributed to the sorbitol plasticizer, known for its elongation-enhancing properties, which improved interactions with other fillers. Consequently, the elongation value increased due to the ability of the plasticizer to reduce brittleness and enhance the flexibility of polymer film by breaking hydrogen bonds between the molecules. At this concentration, elasticity was enhanced as the plasticizer weakened the bonds between amylose, amylopectin, and amylose-amylopectin molecules within the starch matrix. In this case, hydrogen bonds were formed between the plasticizer and starch molecules. This process was associated with disrupting and reconstructing starch molecular chains, resulting in reduced stiffness and increased film flexibility, allowing more movement.[Citation63]
Similar results were reported by Salari et al.,[Citation64] which found a decrease in elongation up to 4% NCC but observed an increase at 6% concentration. This pattern suggested that the addition of NCC above 5% reduced stiffness but linearly increased tensile strength and elastic modulus, ultimately improving the entire matrix and interface. Furthermore, Chi et al.[Citation65] observed higher elongation and tensile strength values at a concentration of 10%. This could be attributed to the even distribution and interaction between the typically rigid NCC fibers with other nanocomposite film materials, leading to high consistency, uniformity, and intractability.
Elephant foot-yam starch, which acted as a biopolymer in this study, also affected the mechanical characteristics of the resulting nanocomposite film. This was evident in the elongation values, which all met JIS (minimum elongation value of 70%). All four treatments had values above 80%, with the control treatment having the highest value of 138%. The elongation percentage plays a crucial role in bioplastics, particularly to amylose content and its impact on material properties. Lower amylose content produces materials with reduced strength but increased flexibility, primarily due to the optimal hydrogen bonding between the unbranched chains, contributing to relatively high elongation values.
Starch-based bioplastics typically have brittle, discontinuous, stiff, and fragile properties without additives and plasticizers. Common plasticizers such as glycerol and sorbitol, which are compatible with polysaccharides, are used to enhance the flexibility of bioplastics, contributing to a higher elongation value.[Citation66] Sorbitol molecules disrupted starch cohesiveness, reduced intermolecular interactions, and increased polymer mobility. The formation of hydrogen bonds with starch reduced the interaction pressure to intensify the mobility of the molecular chain. The starch bonds disrupted by the sorbitol plasticizer caused film (bioplastic) to become more flexible, reducing its stiffness. As stated by a previous study, sorbitol is relatively easier to place within the intra/intermolecular space of starch due to its smaller molecular weight.[Citation67,Citation68]
The mechanical properties of nanocomposite film were also influenced by the amylose and amylopectin content of starch. The ratio of amylose and amylopectin content in elephant foot-yam starch was 24.8% versus 45.16%. This shows a lower amylose content as tuber-based starch usually has a value ranging from 26% to 35%. Low amylose content tends to form crystals with greater mechanical properties than the amorphous amylopectin. Amylose is a linear polymer of anhydrous glucose units, while amylopectin is a branched polymer. The crystalline nature of low amylose content causes starch molecules to be brittle when used as raw material for bioplastics.[Citation62,Citation69]
X-ray diffraction (XRD)
XRD pattern of film presented in shows that the control (without NCC) lacked diffraction peaks with high intensity. XRD curve showed an intermediate intensity peak at 2θ = 17.92°, a strong peak at 2θ = 23.05°, and a weak peak at 2θ = 14.7°.[Citation70] During film preparation, starch granules were destroyed through gelatinization by heat, resulting in the loss of the original crystal peaks. Consequently, the control starch film (without the addition of NCC) had an amorphous structure characterized by a broad peak centered around 2θ = 20°.[Citation71]
In the 7% NCC group, several diffraction peaks appeared with strong intensity at 2θ = 17.12°(101̅), 22.75° (002), and 35.0° (040), corresponding to the crystal diffraction peaks of NCC (cellulose I) at 2θ = 14.7° (101), 16.5° (101̅), 22.6° (002), and 34.5° (040).[Citation72] The presence of these peaks indicated that NCC added during film manufacturing process using the casting method remained in the matrix, thereby increasing the crystallinity. Additionally, the typical diffraction peak of cellulose I, which was lost in film matrix at 2θ = 14.7°, and a slight shift in the diffraction angle suggested coverage by other film-forming materials.
Conclusion
In conclusion, the use of NCC nanoparticles improved the physico-chemical, mechanical, and permeability properties of elephant foot-yam starch and sorbitol-based nanocomposite film. In this study, indicators that produced significant differences with increasing NCC concentration included WVTR, tensile strength, elongation at break, modulus of elasticity, thickness, water solubility, color a*, and biodegradability. On the other hand, L* color and b* color were not significantly impacted. The 7% NCC concentration was identified as the most optimum treatment with a tensile strength value of (3.756 MPa), WVTR (4.256 g/m2.h), elongation (118.06%), elastic modulus (23.81 MPa), thickness (0.22 mm), solubility (33.13%), biodegradability (22.57%), L* value (96.83), a* value (0.106), and b* value (1,496). Furthermore, this film had several diffraction peaks that appeared with strong intensity at 2θ = 17.12°(101̅), 22.75° (002), and 35.0° (040), and influencing both SEM and FTI-R tests. Based on the result, this nanocomposite had the potential to be developed into environmentally friendly packaging in the future.
Disclosure statement
No potential conflict of interest was reported by the author(s).
Additional information
Funding
References
- Atiwesh, G.; Mikhael, A.; Parrish, C. C.; Banoub, J.; Le, T.-A. T. Environmental Impact of Bioplastic Use: A Review. Heliyon. Sep 2021, 7(9), e07918. DOI: 10.1016/j.heliyon.2021.e07918.
- Herawati, H. Potential for Development of Digestible Starch Products as Functional Food. J. Lit. Pertanian May 2010, 30(1), 31–39.
- Clarinsa, R. M.; Sutoyo, S. Manufacturing and Characterizing Biodegradable Plastic from Hdpe (High Density Polyethylene) Composite and ELephant Foot-Yam Tuber Starch. Unesa J. Chem. Jan 2021, 10(1), 85–95. DOI: 10.26740/ujc.v10n1.p85-95.
- Adriansyah, R. C. E. Characterization of Physicochemical and Functional Properties of Elephant Foot-Yam Starch (Amorphophallus Campanulatus Var. Hortensis) Using the Heat Moisture Treatment Method; Bogor Institute of Agriculture: Bogor, 2014.
- Hasan, M.; Gopakumar, D. A.; Olaiya, N. G.; Zarlaida, F.; Alfian, A.; Aprinasari, C.; Alfatah, T.; Rizal, S.; Khalil, H. P. S. A. Evaluation of the Thermomechanical Properties and Biodegradation of Brown Rice Starch-Based Chitosan Biodegradable Composite Film. Int. J. Biol. Macromol. Aug, 2020, 156, 896–905. DOI: 10.1016/j.ijbiomac.2020.04.039.
- Harunsyah, M. Y.; Fauzan, R.; Fauzan, R. Mechanical Properties of Bioplastics Cassava Starch Film with Zinc Oxide Nanofiller as Reinforcement. IOP Conf. Ser Mater. Sci. Eng. Jun, 2017, 210, 012015. DOI: 10.1088/1757-899X/210/1/012015.
- Abdullah, A. H. D.; Putri, O. D.; Fikriyyah, A. K.; Nissa, R. C.; Intadiana, S. Effect of Microcrystalline Cellulose on Characteristics of Cassava Starch-Based Bioplastic. Polym. Plast. Technol. Eng. Aug 2020, 59(12), 1250–1258. doi:10.1080/25740881.2020.1738465.
- Ounkaew, A.; Janaum, N.; Kasemsiri, P.; Okhawilai, M.; Hiziroglu, S.; Chindaprasirt, P. Synergistic Effect of Starch/Polyvinyl Alcohol/Citric Acid Films Decorated with in-Situ Green-Synthesized Nano Silver on Bioactive Packaging Films. J. Environ. Chem. Eng. Dec 2021, 9(6), 106793. doi:10.1016/j.jece.2021.106793.
- Lagaron, J. M.; Lopez-Rubio, A. Nanotechnology for Bioplastics: Opportunities, Challenges and Strategies. Trends Food Sci. Technol. Nov 2011, 22(11), 611–617. doi:10.1016/j.tifs.2011.01.007.
- Jamróz, E.; Kulawik, P.; Kopel, P. The Effect of Nanofillers on the Functional Properties of Biopolymer-Based Films: A Review. Pol. (Basel). Apr 2019, 11(4), 675. DOI: 10.3390/polym11040675.
- Arrieta, M. P.; Peponi, L.; López, D.; López, J.; Kenny, J. M. An Overview of Nanoparticles Role in the Improvement of Barrier Properties of Bioplastics for Food Packaging Applications. In Food Packaging; Elsevier: 2017; pp. 391–424. doi:10.1016/b978-0-12-804302-8.00012-1
- Landry, V.; Alemdar, A.; Blanchet, P. Nanocrystalline Cellulose: Morphological, Physical, and Mechanical Properties. For. Products J. 2011, 61(2), 104–112. DOI: 10.13073/0015-7473-61.2.104.
- Ilyas, R. A.; Sapuan, S. M.; Lamin Sanyang, M.; Ridzwan Ishak, M., “Nanocrystalline Cellulose Reinforced Starch-Based Nanocomposites: A Review,” 5th Postgraduate Seminar On Natural Fiber Composites, Dec. 2016, [Online]. Available: https://www.researchgate.net/publication/315675302
- Noshirvani, N.; Hong, W.; Ghanbarzadeh, B.; Fasihi, H.; Montazami, R. Study of cellulose nanocrystal doped starch-polyvinyl alcohol bionanocomposite films. Int. J. Biol. Macromol. Feb 2018, 107, 2065–2074. DOI: 10.1016/j.ijbiomac.2017.10.083.
- Noshirvani, N.; Ghanbarzadeh, B.; Fasihi, H.; Almasi, H. Starch–PVA Nanocomposite Film Incorporated with Cellulose Nanocrystals and MMT: A Comparative Study. Int. J. Food Eng. Feb 2016, 12(1), 37–48. doi:10.1515/ijfe-2015-0145.
- Yildirim-Yalcin, M.; Tornuk, F.; Toker, O. S. Recent Advances in the Improvement of Carboxymethyl Cellulose-Based Edible Films. Trends Food Sci. Technol. Nov 2022, 129, 179–193. DOI: 10.1016/j.tifs.2022.09.022.
- Azwar, E.; Asmara, P.; Darni, D. Y. Characterization of Edible Film from Corn Starch with Glycerol Plasticizer and CMC Filler as Food Packaging Material. Journal Teknologi dan Inovasi Industri. 2022, 03(1), 23–31.
- Yaradoddi, J. S.; Banapurmath, N. R.; Ganachari, S. V.; Soudagar, M. E. M.; Mubarak, N. M.; Hallad, S.; Hugar, S.; Fayaz, H. Biodegradable Carboxymethyl Cellulose Based Material for Sustainable Packaging Application. Sci. Rep. 2020 Dec, 10(1), 21960. DOI: 10.1038/s41598-020-78912-z.
- Saputra, A.; Lutfi, M.; Masruroh, E. Study on the Production and Mechanical Characteristics of Biodegradable Plastics Made from Elephant Foot-Yam (Amorphophallus Campanulatus). Jurnal Keteknikan Pertanian Tropis dan Biosistem Feb 2015, 3(1), 1–6.
- Arifin, H. R.; Djali, M.; Nurhadi, B.; Hasim, S. A.; Hilmi, A.; Puspitasari, A. V. Improved Properties of Corn Starch-Based Bio-Nanocomposite Film with Different Types of Plasticizers Reinforced by Nanocrystalline Cellulose. Int. J. Food. Prop. Dec 2022, 25(1), 509–521. doi:10.1080/10942912.2022.2052085.
- Al Fath, M. T.; Lubis, M.; Ayu, G. E.; Dalimunthe, N. F. Effect of Nanocrystalline Cellulose from Palm Oil Fiber as a Filler and Potassium Chloride as a Dispersing Agent on the Physical Properties of Avocado Seed Starch (Persea Americana) Based Bioplastics. J. Chem. Eng USU. Sep 2022, 11(2), 89–94. DOI: 10.32734/jtk.v11i2.9239.
- Nasution, H.; Harahap, H.; Al Fath, M. T.; Afandy, Y. Physical Properties of Sago Starch Biocomposite Filled with Nanocrystalline Cellulose (NCC) from Rattan Biomass: The Effect of Filler Loading and Co-Plasticizer Addition. IOP Conf. Ser Mater. Sci. Eng. Feb, 2018, 309, 012033. DOI: 10.1088/1757-899X/309/1/012033.
- Rahmawati, M.; Arief, M.; Satyantini, W. H. The Effect of Sorbitol Addition on the Characteristic of Carrageenan Edible Film. IOP Conf. Ser. Earth Environ. Sci. Mar, 2019, 236, 012129. DOI: 10.1088/1755-1315/236/1/012129.
- Arifin, H. R.; Djali, M.; Nurhadi, B.; Hasim, S. A.; Hilmi, A.; Puspitasari, A. V. Improved Properties of Corn Starch-Based Bio-Nanocomposite Film with Different Types of Plasticizers Reinforced by Nanocrystalline Cellulose. Int. J. Food. Prop. 2022, 25(1), 509–521. DOI: 10.1080/10942912.2022.2052085.
- Al Fath, M. T.; Nasution, H.; Harahap, H.; Ayu, G. E., “Biocomposite of Pectin and Starch Filled with Nanocrystalline Cellulose (NCC): The Effect of Filler Loading and Glycerol Addition,” in AIP Conference Proceedings, American Institute of Physics Inc, Nov. 2019. doi: 10.1063/1.5134576.
- Nasution, H.; Afandy, Y.; Al-Fath, M. T. Effect of Cellulose Nanocrystals (CNC) Addition and Citric Acid as Co-Plasticizer on Physical Properties of Sago Starch Biocomposite; 2018; p. 020039. DOI: 10.1063/1.5030261.
- Arifin, H. R.; Djali, M.; Nurhadi, B.; Azlin-Hasim, S.; Masruchin, N.; Vania, P. A.; Hilmi, A. Corn Starch-Based Bionanocomposite Film Reinforced with ZnO Nanoparticles and Different Types of Plasticizers. Front. Sustain. Food Syst. 6, Jul, 2022. 10.3389/fsufs.2022.886219.
- Oluwasina, O. O.; Akinyele, B. P.; Olusegun, S. J.; Oluwasina, O. O.; Mohallem, N. D. S. Evaluation of the Effects of Additives on the Properties of Starch-Based Bioplastic Film. Sn. Appl. Sci. Apr 2021, 3(4), 421. DOI: 10.1007/s42452-021-04433-7.
- Hasri, M. S.; Pratiwi, D. E. Synthesis and characterization of bioplastics made from chitosan combined using glycerol plasticizer. Ind. J. Fund. Sci. Oct2021, 7(2): 110–119.
- Febrianto Sinaga, R.; Gita Minawarisa Ginting, M. H. S. G.; Hasibuan, R.; Hasibuan, R. The Effect of Adding Glycerol on the Properties of Tensile Strength and Elongation at Break of Bioplastic from Taro Tumber Starch. J. Chem. Eng USU. Jul 2014, 3(2), 19–24. DOI: 10.32734/jtk.v3i2.1608.
- Illing, I.; MB, S. Bioplastic FTIR Test from Sago Drugs Waste with the Addition of Varied Gelatin Concentration. J. Dinamika Sep 2017, 8(2), 1–13.
- Shafqat, A.; Al-Zaqri, N.; Tahir, A.; Alsalme, A. Synthesis and Characterization of Starch Based Bioplatics Using Varying Plant-Based Ingredients, Plasticizers and Natural Fillers. Saudi J. Biol. Sci. Mar 2021, 28(3), 1739–1749. doi:10.1016/j.sjbs.2020.12.015.
- Haghighi, H.; Maria G.; Salvatore L. C.; Frank P.; Heinz W. S.; Fabio L.; Andrea P. Characterization of Bio-Nanocomposite Films Based on Gelatin/Polyvinyl Alcohol Blend Reinforced with Bacterial Cellulose Nanowhiskers for Food Packaging Applications. Food. Hydro. Apr 2021, 113, 106454. DOI: 10.1016/j.foodhyd.2020.106454.
- Fitriani, F.; Aprilia, S.; Arahman, N.; Bilad, M. R.; Suhaimi, H.; Huda, N. Properties of Biocomposite Film Based on Whey Protein Isolate Filled with Nanocrystalline Cellulose from Pineapple Crown Leaf. Polymers (Basel). Dec 2021, 13(24), 4278. DOI: 10.3390/polym13244278.
- de S Coelho, C. C.; Silva, R. B. S.; Carvalho, C. W. P.; Rossi, A. L.; Teixeira, J. A.; Freitas-Silva, O.; Cabral, L. M. C. Cellulose Nanocrystals from Grape Pomace and Their Use for the Development of Starch-Based Nanocomposite Films. Int. J. Biol. Macromol. Sep, 2020, 159, 1048–1061. DOI: 10.1016/j.ijbiomac.2020.05.046.
- Rostini, I.; Intan Pratama, R.; Rochima, E. Characterization of Bioplastic Packaging from Tapioca Flour Modified with the Addition of Chitosan and Fish Bone Gelatin. World Sci. News. 2019, 135, 85–98. [Online]. Available. www.worldscientificnews.com
- Idris, A.; Muntean, A.; Mesic, B. A Review on Predictive Tortuosity Models for Composite Films in Gas Barrier Applications. J. Coat. Technol. Res. May 2022, 19(3), 699–716. DOI: 10.1007/s11998-021-00579-6.
- Sikora, J. W.; Majewski, Ł.; Puszka, A. Modern Biodegradable Plastics—Processing and Properties Part II. Materials. May 2021, 14(10), 2523. doi:10.3390/ma14102523.
- Shojaeiarani, J.; Bajwa, D. S.; Chanda, S. Cellulose nanocrystal based composites: A review. Compos. Part C: Open Access. Jul 2021, 5, 100164. DOI: 10.1016/j.jcomc.2021.100164.
- George, J.; Sabapathi, S. N. Cellulose Nanocrystals: Synthesis, Functional Properties, and Applications. Nanotechnol. Sci. Appl. Nov 2015, 45. 10.2147/NSA.S64386.
- Santana, R. F.; Bonomo, R. C. F.; Gandolfi, O. R. R.; Rodrigues, L. B.; Santos, L. S.; dos Santos Pires, A. C.; de Oliveira, C. P.; da Costa Ilhéu Fontan, R.; Veloso, C. M. Characterization of Starch-Based Bioplastics from Jackfruit Seed Plasticized with Glycerol. J. Food Sci. Technol. 2018 Jan, 55(1), 278–286. DOI: 10.1007/s13197-017-2936-6.
- Saiful, H. H.; Saleha, S.; Iqbalsyah, T. M.; Iqbalsyah, T. M. Development of Bioplastic from Wheat Janeng Starch for Food Packaging. IOP Conf. Ser Mater. Sci. Eng. May 2019, 523(1), 012015. DOI: 10.1088/1757-899X/523/1/012015.
- Lusiana, S.; Putri, D.; Nurazizah, I. Z.; Bahruddin. Bioplastic Properties of Sago-PVA Starch with Glycerol and Sorbitol Plasticizers. J. Phys. Conf. Ser. Nov 2019, 1351(1), 012102. doi:10.1088/1742-6596/1351/1/012102.
- Abdollahi, M.; Alboofetileh, M.; Behrooz, R.; Rezaei, M.; Miraki, R. Reducing Water Sensitivity of Alginate Bio-Nanocomposite Film Using Cellulose Nanoparticles. Int. J. Biol. Macromol. Mar 2013, 54, 166–173. DOI: 10.1016/j.ijbiomac.2012.12.016.
- Arief, M. D.; Mubarak, A. S.; Pujiastuti, D. Y., “The Concentration of Sorbitol on Bioplastic Cellulose Based Carrageenan Waste on Biodegradability and Mechanical Properties Bioplastic,” IOP Conference Series: Earth and Environmental Science, vol. 679, p. 012013, Feb. 2021, doi: 10.1088/1755-1315/679/1/012013.
- Widiriani, R. Ecofarming Model to Achieve Sustainable Farming Systems in High-Altitude Lands Utilized by Communities (Case Study in Lembang Subdistrict, West Bandung Regency, and Dongko Subdistrict, Trenggalek Regency); Bogor Agricultural Institute: Bogor, 2009.
- Ghasemlou, M.; Daver, F.; Murdoch, B. J.; Ball, A. S.; Ivanova, E. P.; Adhikari, B. Biodegradation of Novel Bioplastics Made of Starch, Polyhydroxyurethanes and Cellulose Nanocrystals in Soil Environment. Sci. Total Environ. Apr 2022, 815, 152684. DOI: 10.1016/j.scitotenv.2021.152684.
- Folino, A.; Karageorgiou, A.; Calabrò, P. S.; Komilis, D. Biodegradation of Wasted Bioplastics in Natural and Industrial Environments: A Review. Sustainability. Jul 2020, 12(15), 6030. DOI: 10.3390/su12156030.
- Karimi, M.; Naimi-Jamal, M. R. Carboxymethyl Cellulose as a Green and Biodegradable Catalyst for the Solvent-Free Synthesis of Benzimidazoloquinazolinone Derivatives. J. Saudi Chem. Soc. Feb 2019, 23(2), 182–187. doi:10.1016/j.jscs.2018.06.007.
- Li, R.; Zhu, X.; Peng, F.; Lu, F. Biodegradable, Colorless, and Odorless PLA/PBAT Bioplastics Incorporated with Corn Stover. ACS Sustain. Chem. Eng. Jun 2023, 11(24), 8870–8883. doi:10.1021/acssuschemeng.3c00691.
- Neves, A. C.; Ming, T.; Mroczkowska, M.; Culliton, D. The Effect of Different Starches in the Environmental and Mechanical Properties of Starch Blended Bioplastics. Adv Sci, Tech & Engi. Sys. J. Nov 2020, 5(6), 550–554. DOI: 10.25046/aj050666.
- Agusman; Fransiska, D.; Murdinah; T.; Irianto, H. E.; Priambudi, P.; Fateha; Abdullah, A. H. D.; Nissa, R. D.; Firdiana, B. Nurhayati Physical Properties of Bioplastic Agar/Chitosan Blend. IOP Conf. Ser. Earth Environ. Sci. Feb 2022, 978(1), 012046. DOI: 10.1088/1755-1315/978/1/012046.
- Montava-Jorda, S.; Lascano, D.; Quiles-Carrillo, L.; Montanes, N.; Boronat, T.; Martinez-Sanz, A.V.; Ferrandiz-Bou, S; Torres-Giner, S. Mechanical Recycling of Partially Bio-Based and Recycled Polyethylene Terephthalate Blends by Reactive Extrusion with Poly(styrene-Co-Glycidyl Methacrylate). Polymers (Basel). Jan 2020, 12(1), 174. DOI: 10.3390/polym12010174.
- Omidi, M.; Fatehinya, A.; Farahani, M;, Akbari, Z.; Shahmoradi, S.; Yazdian, F.; Tahriri, M.; Moharamzadeh, K.; Tayebi, L.; Vashaee, D. Characterization of biomaterials,” in Biomaterials for Oral and Dental Tissue Engineering, Elsevier; 2017pp. 97–115. 10.1016/B978-0-08-100961-1.00007-4.
- Amin, M. R.; Chowdhury, M. A.; Kowser, M. A. Characterization and Performance Analysis of Composite Bioplastics Synthesized Using Titanium Dioxide Nanoparticles with Corn Starch. Heliyon. Aug 2019, 5(8), e02009. doi:10.1016/j.heliyon.2019.e02009.
- Agustin, M. B.; Ahmmad, B.; Alonzo, S. M. M.; Patriana, F. M. Bioplastic Based on Starch and Cellulose Nanocrystals from Rice Straw. J. Reinf. Plast. Compos. Dec 2014, 33(24), 2205–2213. doi:10.1177/0731684414558325.
- Trache, D., Tarchoun, A. F.; Derradji, M.; Hamidon, T. S.; Masruchin, N.; Brosse, N.; Hussin, M. H. Nanocellulose: From Fundamentals to Advanced Applications. Front. Chem. 8, May, 2020. 10.3389/fchem.2020.00392.
- Muhammad, A.; Roslan, A.; Sanusi, S. N. A.; Shahimi, M. Q.; Nazari, N. Z. Mechanical Properties of Bioplastic Form Cellulose Nanocrystal (CNC) Mangosteen Peel Using Glycerol as Plasticizer. J. Phys.: Conf. Ser. Nov 2019, 1349(1), 012099. DOI: 10.1088/1742-6596/1349/1/012099.
- Boey, J. Y.; Lee, C. K.; Tay, G. S. Factors Affecting Mechanical Properties of Reinforced Bioplastics: A Review. Polymers (Basel). Sep 2022, 14(18), 3737. DOI: 10.3390/polym14183737.
- Ilyas, R. A.; Sapuan, S. M.; Ishak, M. R.; Zainudin, E. S. Development and Characterization of Sugar Palm Nanocrystalline Cellulose Reinforced Sugar Palm Starch Bionanocomposites. Carbohydr. Polym. Dec 2018, 202, 186–202. DOI: 10.1016/j.carbpol.2018.09.002.
- El Achaby, M.; Kassab, Z.; Barakat, A.; Aboulkas, A. Alfa Fibers as Viable Sustainable Source for Cellulose Nanocrystals Extraction: Application for Improving the Tensile Properties of Biopolymer Nanocomposite Films. Ind. Crops Prod. Feb 2018, 112, 499–510. DOI: 10.1016/j.indcrop.2017.12.049.
- Gabriel, A. A.; Solikhah, A. F.; Rahmawati, A. Y. Tensile Strength and Elongation Testing for Starch-Based Bioplastics Using Melt Intercalation Method: A Review. J. Phys.: Conf. Ser. Apr 2021, 1858(1), 012028. DOI: 10.1088/1742-6596/1858/1/012028.
- Lubis, M.; Harahap, M. B.; Ginting, M. H. S.; Sartika, M.; Hidayatul, A., “Effect of Microcrystalline Cellulose (MCC) from Sugar Palm Fibres and Glycerol Addition on Mechanical Properties of Bioplastic from Avocado Seed Starch (Persea Americana Mill),” in International Conference on “Engineering & Technology, Computer, Basics and Applied Sciences” ECBA, Academic Fora, Istanbul, Turkey, 2016.
- Salari, M.; Sowti Khiabani, M.; Rezaei Mokarram, R.; Ghanbarzadeh, B.; Samadi Kafil, H. Development and Evaluation of Chitosan Based Active Nanocomposite Films Containing Bacterial Cellulose Nanocrystals and Silver Nanoparticles. Food Hydrocoll. Nov 2018, 84, 414–423. DOI: 10.1016/j.foodhyd.2018.05.037.
- Chi, K.; Catchmark, J. M. Improved Eco-Friendly Barrier Materials Based on Crystalline Nanocellulose/Chitosan/Carboxymethyl Cellulose Polyelectrolyte Complexes. Food Hydrocoll. Jul 2018, 80, 195–205. DOI: 10.1016/j.foodhyd.2018.02.003.
- Abe, M. M.; Martins, J. R.; Sanvezzo, P. B.; Macedo, J. V.; Branciforti, M. C.; Halley, P.; Botaro, V. R.; Brienzo, M. Advantages and Disadvantages of Bioplastics Production from Starch and Lignocellulosic Components. Polymers (Basel). Jul 2021, 13(15), 2484. DOI: 10.3390/polym13152484.
- Ginting, M. H. S.; Lubis, M.; Sidabutar, T.; Sirait, T. P. The Effect of Increasing Chitosan on the Characteristics of Bioplastic from Starch Talas (Colocasia Esculenta) Using Plasticizer Sorbitol. IOP Conf. Ser. Earth Environ. Sci. Mar 2018, 126, 012147. DOI: 10.1088/1755-1315/126/1/012147.
- Tamara, T.; Sumari, N.; Arni, S. Properties of Cassava Starch-Based Bioplastics and CMC with Sorbitol as a Plasticizer. IOP Conf. Ser. Earth Environ. Sci. Feb 2020, 456(1), 012077. DOI: 10.1088/1755-1315/456/1/012077.
- Ratnawati, R.; Aisa, A.; Aryanti, N.; Kumoro, A. C. Glycerol and Temperature Effects on Mechanical Properties of Biodegradable Plastic from Rice Four with Rice Husk Filler. Chem. Eng. Trans. 2022, 95, 223–228. DOI: 10.3303/CET2295038.
- Reddy, C. K.; Haripriya, S.; Noor Mohamed, A.; Suriya, M. Preparation and Characterization of Resistant Starch III from Elephant Foot Yam (Amorphophallus Paeonifolius) Starch. Food. Chem. Jul 2014, 155, 38–44. DOI: 10.1016/j.foodchem.2014.01.023.
- Xu, Y.; Scales, A.; Jordan, K.; Kim, C.; Sismour, E. Starch Nanocomposite Films Incorporating Grape Pomace Extract and Cellulose Nanocrystal. J. Appl. Polym. Sci. Feb 2017, 134(6). DOI:10.1002/app.44438.
- Bondancia, T. J.; De Aguiar, J.; Batista, G.; Cruz, A. J. G.; Marconcini, J. M.; Mattoso, L. H. C.; Farinas, C. S. Production of Nanocellulose Using Citric Acid in a Biorefinery Concept: Effect of the Hydrolysis Reaction Time and Techno-Economic Analysis. Ind. Eng. Chem. Res. 2020 Jun, 59(25), 11505–11516. DOI: 10.1021/acs.iecr.0c01359.