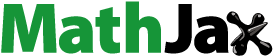
ABSTRACT
Thai indigenous rice varieties provide valuable genetic resources due to their environmental resilience, but it is yet to be elucidated whether their endosperm starches are suitable for whole grain consumption or industrial applications. Here, 19 Thai indigenous rice starches were explored for compositional, structural, and physicochemical characteristics. Grain reducing sugar, protein, and amylose contents significantly differed among genotypes. Their starches were categorized as waxy, very low-, and low-amylose starches. The varieties with low-amylose starches displayed higher amylose content but lower crystallinity than the varieties with waxy and very low-amylose starches. The largest median starch granule size (d(0.5)) was also observed in most varieties with low-amylose starches. The starch compositional and structural variations contributed to substantial differences in starch physicochemical properties among these genotypes. The partial least squares discriminant analysis revealed that amylose content, setback, swelling power, reducing sugar, C- and A-type granules, d(0.5), and final viscosity could discriminate the low-amylose starches from the waxy and very low-amylose starches. Notably, correlation analyses revealed that amylose content was the most crucial factor determining starch physicochemical properties of these varieties. Starch compositional, structural, and physicochemical variations among the indigenous rice varieties can be adopted as alternative industrial raw materials and as genetic resources for future breeding programs.
Introduction
Rice (Oryza sativa L.) is a staple food for the world’s population, and it is also one of the most important agricultural commodities in Thailand.[Citation1] Rice is an important source of income for Thai farmers and as a main export product, it also provides large income for the country.[Citation2] Thailand has approximately 10 million hectares of rice cultivation area and more than 30 million tons of paddy from both the rainy and dry seasons can be produced annually.[Citation3] The largest areas for high-quality rice cultivation are in the Northeast Region of Thailand.[Citation2] This cultivation location has a large rain-fed lowland ecosystem with both soil series and climate suitable for planting local and commercial rice cultivars.[Citation2] In addition, Thailand’s rice fields are among the vital centers of origin and genetic diversity of rice.[Citation4] A great variety of Thai rice genotypes provide differences in grain functionality, serving the diverse demands of rice consumers and rice processing industries.[Citation4,Citation5]
Thai rice is generally cooked and consumed as intact grains, while Thai rice starches also provide primary materials for manufacturing starch-rich food products such as noodles, bakeries, beverages, pharmaceuticals and supplements, cosmetics, animal feed, extruded products, and novel products (e.g., gluten free based foods, infant’s foods, and snacks).[Citation5–7] The quality of these products is intrinsically attributed to the physicochemical properties (i.e., swelling power, solubility, and thermal and pasting properties) of the raw starch materials. These starch physicochemical features are, in turn, controlled by the molecular composition (i.e., protein, amylose, and amylopectin contents) and structure (i.e., granular size, relative crystallinity, and amylopectin fine structure).[Citation5,Citation6] Rice starch is stored as discrete semi-crystalline granules that range in size of 3–9 μm and are made up of alternate arrangements of crystalline lamellar structure (consisting of double-helices of amylopectin branch chains) and amorphous lamellar structure (consisting of amylopectin branching points and linear amylose molecules).[Citation8] Rice starch containing large amounts of amylose and non-starch components, such as protein and soluble fiber, can be readily dissolved in water but hardly swelled upon heating.[Citation1,Citation9] In addition, rice starch consisting of higher proportions of amylose and amylopectin long branch chains and a small median granule size showed higher gelatinization temperatures compared to starch with lower amylose and amylopectin long branch chains and a larger median granule size.[Citation5,Citation9,Citation10] Moreover, the presence of large amounts of amylose and long amylopectin branch chains within gelatinized starch promoted the recrystallization process of dispersed polymer chains, which reassembled into the new double helices, gel networks, and eventually strong starch gels.[Citation5,Citation9,Citation10] The reassembled structure would absorb the high melting temperature and enthalpy to completely dissociate its retrograded structures.[Citation5,Citation9,Citation10] Therefore, rice starch displaying high gelatinization and retrogradation temperatures would require high energy and long duration upon cooking. It could also produce starch-rich food products with high degrees of hardness, low adhesiveness, and deteriorated eating and cooking quality.[Citation5,Citation11]
In Thailand, rice farmers preferably cultivate popular commercial rice cultivars to serve the demands from the rice markets. This contributes to the cultivation of local rice varieties being replaced by the limited number of commercial cultivars.[Citation12] However, most commercial cultivars are sensitive to both biotic and abiotic stresses, which can result in significant losses in yield and grain quality, and an eventual reduction in farmers’ incomes.[Citation13] Thai indigenous rice varieties, which are particularly tolerant to environmental stresses, provide important rice genetic resources for breeding new rice varieties with stress tolerant traits. They also receive increasing attention as alternative rice genotypes that may yield grains and endosperm starches with physicochemical and functional properties suitable and desirable for extensive food and non-food industrial applications.[Citation6,Citation13] Similar to commercial rice kernels, the starch, protein, and amylose contents in the kernels of Thai indigenous rice varieties were in the ranges of 74.54% to 93.32%,[Citation6,Citation14] 5.01% to 8.14%,[Citation6] and 1.20% to 28.90%,[Citation6,Citation14,Citation15] respectively. Variations in the chemical composition directly affected the physicochemical and functional properties of Thai indigenous rice flours.[Citation6,Citation14,Citation15] The Southern Thai rice flours containing a lower amylose content and a higher protein content showed lower peak viscosity (PV), holding strength (HS), breakdown (BD), final viscosity (FV), and setback (SB) values than those of Southern Thai rice flours containing a higher amylose content and a lower protein content.[Citation6] Additionally, the positive correlations between amylose content and pasting parameters (e.g., Ptime and HS) were previously observed in Thai indigenous non-pigmented and pigmented rice flours.[Citation15] There have been reports on the compositional, physicochemical, and functional characteristics of flours obtained from Southern and Northern Thai indigenous rice. However, in-depth characterization of starches obtained from Thai indigenous rice varieties in the Northeastern Thailand, where several indigenous varieties were locally planted and descended through generations of local farmers has been lacking. In this study, the 19 different indigenous rice varieties, which have been locally and culturally cultivated in the Northeast Region of Thailand, were explored for their grain storage product compositions, starch molecular composition and structure, and starch physicochemical and functional properties. These starch features are crucial in determining their unique eating and cooking qualities, serving the desirable rice quality requirements of both the consumers and the industrial sectors.
Materials and methods
Plant materials
Rice caryopses of varieties I Daeng Pla Luk Khrok (EDPLK), Jao I Lom or Khao Lom (EL), Niaw I Tia (ET), Jao Daeng (JD), Jao Loy (JL), Khao Kon (KK), Kham Phai (KP), Kam Pun (KPN), I Lueang Noi (LN), Lao Taek (LT), Man Pet (MP), Niaw Daeng (ND), Niaw Dum (NDD), Nahng Nuan (NN), Plong Aeo (PA), San Pla Tong (SPT), Ta Dee (TD), I Tom Khao (TW), and Unknown (UN) were supplied by the Department of Agronomy, Faculty of Agriculture, Khon Kaen University. The paddies of 19 rice varieties were shown in Figure S1. The caryopses were dried and kept at room temperature in plastic bags with silica gel desiccant until analysis.
Grain storage biomolecule and starch chemical composition analyses
The analyses of storage biomolecule composition in the grain were performed on rice flour samples (i.e., reducing sugar, starch, nitrogen, and protein contents) and rice starch samples (i.e., amylose and moisture contents).
Reducing sugar and total starch content: The flour samples prepared from the dehulled indigenous rice seeds using a manual coffee grinder (CG-01, Zhejiang, China) were filtered through a 35 mesh (0.50 mm) sieve (Humboldt, Raleigh, NC, USA).[Citation16] The filtered flour powder was then used to determine for sugar and starch contents according to previously described methods.[Citation16] A total of 2 mL of 80% (v/v) ethanol were added to 200 mg flour powder, and the suspension was boiled thrice to extract free reducing sugar. The 300 μL ethanol-soluble supernatants were dried using Speed Vac (LaboGene, Kolkata, India). Then, 100 μL of double distilled water was added to reconstitute the sugar residues, and the solution was kept at 4°C for sugar assay. Total starch content was determined with the digestion of the ethanol-insoluble pellet to glucose. Finally, the 3,5-dinitrosalicylic acid assay was applied to assess the amount of reducing sugars present in the reconstituted sugar fraction and the glucoses digested from the starch fraction using glucose as standards.
Total nitrogen (N) and protein content: The N content present in the 1.0 g of rice flour powder was estimated using the Kjeldahl method according to the AOAC standard method 920.87.[Citation17] N values were then converted to protein content by N × 5.95.[Citation17]
Amylose content: Amylose was assayed on 20 mg of isolated rice starch by a Concanavalin A method following the procedures of the Megazyme Amylose/Amylopectin Assay Kit (Megazyme, Wicklow, Ireland).
Moisture content: The standard AOAC method was used to determine the moisture content (%) of the starch powder.[Citation16]
Starch granule isolation and analysis
Starch granule isolation. Starch was isolated by the NaOH extraction method as previously described.[Citation5] Briefly, 10 g of sieved flour powder was soaked in 35 mL of 0.2% (w/v) sodium hydroxide, filtered through four layers of cheesecloth, and centrifuged at 3946 × g for 20 min. The top yellowish layer containing protein and other residues was removed. Thereafter, the starch pellet was redissolved in double distilled water, adjusted to neutral pH, suspended in toluene to remove grain lipids, and washed in double distilled water with centrifugation at 4679 × g for 20 min to completely remove the toluene residues. Finally, the starch pellet was dried at 40°C for 48 h.
Starch granule size and size distribution. The purified starch of 30 mg was weighed and dispersed in 80 mL of double distilled water.[Citation5,Citation9] The starch suspension was then used for granule size distribution analysis on a laser diffraction particle size analyzer (Mastersizer 2000, Malvern Instruments, Malvern, Worcestershire, England).
Relative crystallinity: The starch granule crystallinity was analyzed using an X-ray diffractometer (SmartLab 9 kW, Rigaku, Tokyo, Japan) at 40 kV and 30 mA with a diffraction angle (2θ) of 5–40° and a scanning rate of 2.40°/min. The X-ray diffractogram was generated and used to calculate the degree of crystallinity as previously described.[Citation1]
Swelling power and solubility: One mL of 0.2% (w/v) starch slurry was boiled at 90°C for 30 min and centrifuged at 3118 × g for 30 min at room temperature. The supernatant was dried at 105°C until the weight became constant and recorded. The swelling and solubility behaviors of the indigenous rice starches were determined using the weights of wet pellet and dried supernatant as previously described.[Citation5]
Thermal properties: The starch gelatinization and retrogradation properties were measured by a differential scanning calorimeter (DSC) (STARe System DSC 1, METTLER-TOLEDO, Switzerland) based on the described method.[Citation16] Briefly, starch slurries with a starch-to-water ratio of 1:3 (∼12 mg) were hermetically sealed in an aluminum pan and incubated at room temperature overnight prior to analysis. An empty pan was used as a reference pan. The sample and reference pans were heated at a rate of 10°C/min from 30°C to 90°C for gelatinization analysis. Thereafter, the gelatinized starches were stored at 4 ± 1°C for 14 days, and the sample pans were reheated at a rate of 10°C/min from 30°C to 90°C for retrogradation analysis. The percentage of retrogradation (R%) was calculated using the following formula.[Citation5]
R% = ∆H(r)/∆H(g) × 100
where ∆H(r) is the enthalpy of melting the retrograded starch and ∆H(g) is the gelatinization enthalpy.
Pasting properties: The viscosity of 8% (w/v) starch slurry was analyzed by a rheometer (Physica MCR 51, Anton Paar, Austria). The Rapid Visco-Analyzer (RVA) temperature profile was simulated by the rheometer in the following temperature regime: hold at 50°C for 1 min, heat to 95°C over 4.5 min, hold at 95°C for 3 min, cool to 50°C over 4.5 min, and a final hold at 50°C for 1 min, while maintaining a rotation speed of 160 rpm.[Citation18] The starch viscosity was expressed in millipascal-seconds (mPa.s) and measured in triplicate.
Statistical analysis
Data from three to four replicates were subjected to one-way analysis of variance (ANOVA). Tukey’s post hoc test at p ≤.05 was calculated to compare the means using SPSS® statistics v.23 software (IBM, Armonk, NY, USA).[Citation19] The MetaboAnalyst online software (TMIC, Canada) was used to normalize data via logarithmic transformation and to analyze all variables communally via partial least squares discriminant analysis (PLS-DA).[Citation18,Citation20] The correlation analysis among starch compositional, structural, and physicochemical parameters of the 19 Thai indigenous rice varieties was determined with Pearson’s correlation test.[Citation16]
Results and discussion
Grain storage product and starch chemical composition
Reducing sugar and starch contents: The grain reducing sugar and starch contents of 19 Thai indigenous rice varieties ranged from 0.34 to 1.30 mg/gFW and 695.30 to 756.66 mg/gFW, respectively (). This is consistent with the reported values of 0.54 to 1.64 mg/gFW and 719.73 to 853.15 mg/gFW from Thai certified rice grains, respectively.[Citation5,Citation19] The highest caryopsis reducing sugar content was observed from EL, KP, LN, MP, SPT, TD, and UN rice varieties, whereas EDPLK displayed the lowest grain reducing sugar content (). However, all rice genotypes contained similar amount of grain starch (). Starch is the primary storage biomolecule in rice grains and its properties determine the rice eating and cooking qualities.[Citation21] Grain starch content of Thai certified rice varieties typically varies between 70% and 80% of the total grain weight,[Citation5,Citation9] which is similar to the values found in the kernels of the indigenous rice varieties in this study (). Therefore, these indigenous rice varieties could be used for whole grain consumption and could provide adequate starch for industrial applications.
Table 1. Grain storage biomolecules and starch chemical compositions of 19 Thai indigenous rice varieties.
Protein content: The presence of grain proteins may decrease the amount of available water in the rice grains during the cooking process.[Citation22] Approximately 8% of protein in the rice flour can absorb up to 40% of the available water upon cooking, leaving less water for granular swelling and amylose leakage.[Citation22] It also increased the concentration of the dispersed and viscous phases of gelatinized starch.[Citation22] Accordingly, rice grains with a high protein content required long cooking time and the cooked rice showed increased hardness and less stickiness.[Citation22,Citation23] Here, we found that JL, KPN, LN, and NDD rice possessed the highest grain protein content, while ET and MP rice contained the lowest grain protein content among all rice varieties (). Nonetheless, the grain protein content of all indigenous rice varieties ranged from 5.18% to 6.90% (), which was less than the typical protein content (i.e., 8%) of Thai certified commercial rice grains.[Citation9,Citation22] This suggested that the studied Thai indigenous rice grains could give soft and sticky textures upon cooking.
Amylose content: The amylose content of the 19 rice starches was in a wide range of 1.14% to 15.83% (). This was comparable to the amylose content in pigmented and non-pigmented Thai indigenous rice observed by previous studies.[Citation6,Citation14,Citation15] The waxy Khao Kum (KK) rice variety displayed the lowest amylose content, whereas the non-waxy Jao Loy (JL) rice variety accumulated the highest amylose content in the caryopsis.[Citation15] Similarly, our results show that the non-waxy JD and JL rice starches had the highest amylose content, whereas the waxy SPT rice starch contained the lowest amylose content (). Additionally, rice varieties can be categorized based on the amylose content as waxy rice (0–2%), very low amylose rice (2–10%), low amylose rice (10–20%), intermediate amylose rice (20–25%), and high amylose rice (25–33%).[Citation24,Citation25] Thus, based on the amylose content, we classified the 19 indigenous rice varieties into three groups: (i) the waxy rice varieties consisted of ET (1.58%), KK (1.76%), KP (1.26%), KPN (1.34%), ND (1.87%), NDD (1.58%), NN (1.32%), PA (1.98%), and SPT (1.14%). (ii.) The very low amylose rice varieties comprised LT (3.23%), MP (2.24%), TW (3.66%), and UN (2.96%). Lastly, (iii) The low amylose rice varieties consisted of EDPLK (11.67%), EL (13.67%), JD (15.45%), JL (15.83%), LN (13.28%), and TD (12.53%) ().
Starch structural feature
Starch particle size distribution: Starch granules from the 19 different indigenous rice varieties showed significant variation in size, which was reported as the granule size distribution and the median particle diameter (d(0.5)) ( and ). The starch granules isolated from cereal caryopses were classified based on granular diameter with small, medium, and large granules as follows: C- (<5 µm), B- (5–15 µm), and A- (>15 µm) granules, respectively.[Citation5,Citation26,Citation27] Among the 19 varieties, the C-, B-, and A-type granules contributed 18.66% to 39.63%, 51.29% to 67.52%, and 6.40% to 14.84%, respectively, to the total volume (). The volume distributions of starch granules (%) also revealed that ND, NDD, and SPT starches displayed the highest proportion of C-granules, while EL, JD, JL, and KP starches possessed the lowest proportion of C-granules (). The largest number of B-granules was found in EDPLK, EL, JD, JL, KP, and KPN starches, while the smallest B-type proportion was found in ND starch (). Additionally, the largest proportion of A-granules was observed for EDPLK, EL, ET, JD, JL, KK, KP, KPN, LN, LT, NN, PA, and TD starches, whereas SPT starch had the lowest number of A-granules (). Accordingly, the largest d(0.5) was observed in the low-amylose EDPLK, EL, JD, JL, and LN rice starches and the waxy KP starch, whereas the smallest d(0.5) was observed in the waxy ND starch (). In addition, the d(0.5) values of rice starch isolated from the grains of indigenous rice varieties were found to be in the range of 5.20–8.24 μm (). Interestingly, the indigenous rice varieties, including EDPLK, EL, ET, JD, JL, KK, KP, KPN, LN, and LT, possessed larger median granule sizes (7.64 μm, 7.71 μm, 6.80 μm, 8.19 μm, 8.00 μm, 6.75 μm, 8.24 μm, 7.06 μm, 6.54 μm, and 6.45 μm, respectively ()) than rice starch obtained from Thai commercial rice varieties with the d(0.5) values of 4.33–6.08 μm.[Citation5,Citation9,Citation16] The variation in granular size could be due to the differences in agronomic practices, climatic conditions, rice genotypes, and starch biosynthesis process.[Citation10,Citation25]
undefined 2. Size distribution (volume %), median particle size [d(0.5)], relative crystallinity, swelling power, and solubility of grain starch granules of 19 Thai indigenous rice varieties.
Relative crystallinity: The X-ray diffractograms of all 19 rice starches displayed a comparable pattern (). Their XRD patterns showed the typical A-type diffraction pattern, consisting of strong X-ray resolved peaks at diffraction angles (2θ) of around 15° and 23° and an unresolved doublet peak at 17° and 18° (), which was consistent with XRD patterns of rice and other cereal starches.[Citation8,Citation10,Citation16] Also, the X-ray diffractogram obtained from this study () reveals a weak X-ray diffraction peak at about 20°, which has been associated with amylose-lipid complexes.[Citation5,Citation26] The relative crystallinity computed from X-ray diffractograms exhibited considerable variation among all 19 different rice starches. The relative crystallinity ranged from 33.85% to 40.62% (), which was comparable to the results previously reported for Thai commercial rice starches.[Citation1,Citation16] The diverse arrays of amylose content could be responsible for differences in starch relative crystallinity.[Citation28] The presence of linear amylose in the amorphous lamellae promoted an increase in the amorphous area, while the formation of amylose-lipid complexes intercalating inside the crystalline lamellae could introduce defects in the ordered structures of amylopectin double helices.[Citation16] These phenomena directly influence the stability of the crystalline lamellae,[Citation16] and could eventually cause the granule relative crystallinity to decrease. Accordingly, the waxy rice ET, KP, NDD, NN, and PA starches and the very low-amylose LT and MP rice starches had the highest granule crystallinity, whereas the lowest granule crystallinity was observed for the low-amylose JD and JL rice starches (, and ).
Figure 2. X-ray diffraction patterns of starch granules from 19 Thai indigenous rice varieties. The X-ray diffractogram of all rice starches revealed the typical A-type diffraction pattern, which consists of the strong and weak reflections at these corresponding diffraction angles (2θ): two strong X-ray resolved peaks at 15° and 23°, a strong X-ray unresolved peak at 17° and 18°, and a weak X-ray reflection peak of amylose-lipid complexes at 20°.5, 28

Starch physicochemical properties
Starch swelling power and solubility: Starch chemical composition and molecular structure directly affected swelling power and water solubility of starch granules.[Citation1,Citation9,Citation29] When heating under excess water and minimal mechanical shear conditions, the hydrogen bond derived intra- and intermolecular interactions between starch chains were disrupted.[Citation16] Consequently, the surrounding water molecules form hydrogen bonds in the free hydroxyl groups of amylose long- and amylopectin branch-chains, which are accompanied by amylose leaching from the granules, leading to the increase in water solubility of starch granules.[Citation10] Besides, the exposed hydroxyl groups of amylose molecules could compete with those of amylopectin chains for water binding, which constrained the swelling of starch granules.[Citation16] Accordingly, we observed that starches from the waxy rice KK, NDD, NN, and PA and the very low-amylose MP and UN displayed the highest granular swelling (). Conversely, starches from the low-amylose EDPLK, EL, JD, JL, LN, and TD showed the lowest granular swelling (). The 19 indigenous rice starches possessed the granular swelling values ranging between 11.76 g/g and 36.13 g/g (). These swelling power values were within the range of 9.30 g/g to 55.60 g/g obtained from other Thai rice starch samples.[Citation1,Citation9,Citation16,Citation30] The highest solubility values were also found for the low-amylose JL rice starch (12.49%), while the lowest solubility values were observed for the waxy SPT (5.30%) and low-amylose EL (5.27%) rice starches (). Interestingly, the waxy KP, NDD, NN, and PA and the very low-amylose ET, LT, TW, and UN rice starches with a lower amylose content exhibited higher solubility values than that of the low-amylose EL rice starch containing a higher amylose content (). It is suggested that the discrepancy in water solubility and amylose content might be due to the presence of other water-soluble molecules (e.g., proteins and fibers)[Citation9] in the very low-amylose LT, TW, and UN rice starches. However, the solubility values of all 19 rice starches observed in this study were among the values of Thai certified rice starches (1.14–35.00%) reported by previous research.[Citation1,Citation9,Citation16,Citation30]
Starch thermal properties: The thermal characteristics of starch, which include the transition [i.e., onset (To), peak (Tp), and conclusion (Tc)] temperatures, temperature range (ΔT), and enthalpy (ΔH) of starch gelatinization and retrogradation processes, were assessed and compared among the 19 indigenous rice starches. These starch gelatinization and retrogradation characteristics reflect the temperature and energy required upon cooking, the textural sensory attributes, and the qualities of cooked rice grain upon cooling and storage.[Citation19] Overall, the gelatinization To(g), Tp(g), Tc(g), and ΔT(g) of the indigenous rice starches were between 59.83–71.13°C, 66.56–76.25°C, 71.66–81.12°C, and 8.86–16.81°C, respectively (). The retrogradation To(r), Tp(r), Tc(r), and ΔT(r) of the retrograded starch gels were between 39.09–42.56°C, 50.96–54.49°C, 58.39–64.54°C, and 16.68–24.49°C, respectively (). In addition, the gelatinization and retrogradation enthalpy (ΔH(g) and ΔH(r)) of all indigenous rice starches were between 8.39–13.64 J/g and 1.45–5.43 J/g, respectively (), which is in agreement with the ΔH(g) and ΔH(r) values previously reported for Thai commercial rice starches.[Citation1,Citation9,Citation16] Hence, these indigenous rice grains may require comparable thermal energy upon cooking as the Thai commercial rice grains.[Citation16,Citation19] Since current Thai commercial rice grains are limited to a small number of cultivars, these 19 Thai indigenous rice varieties could offer alternative raw materials for rice starch products. Moreover, LN and JL starches showed the highest gelatinization transition temperatures and the highest Tc(g) value, respectively, while the lowest To(g) value and the lowest Tp(g) and Tc(g) values were found for KP and UN starches and TW starch, respectively (). The low amylose rice LN and JL starches contained higher amylose content than the waxy rice KP and very low amylose rice TW and UN starches (), hence resulting in greater gelatinization temperatures.[Citation16,Citation28] In addition, previous studies demonstrated that rice starches with a larger median granule size[Citation9] and a higher degree of crystallinity[Citation16,Citation28] showed a higher ΔH(g) value than rice starches with a smaller median granule size and a lower degree of crystallinity. Nonetheless, the lowest ΔH(g) value was observed for the low amylose rice LN starch and the very low amylose rice TW starch (), even though LN starch had a lower relative crystallinity than TW starch (). This could be because the LN starch possessed a nonsignificant larger median granule size (p > .05) than that of TW starch (). Rice starches with a large median granule size had a small specific surface area to absorb water molecules, resulting in inefficient hydration and swelling of the granules.[Citation16] Therefore, large starch granules require high thermal energy for phase transition to gel.[Citation16,Citation31] Despite its lower relative crystallinity, the ΔH(g) value () of the low amylose rice LN starch () did not significantly vary from the very low amylose rice TW starch (). The current findings concur with previous research reporting that non-waxy rice starches with a higher relative crystallinity and a smaller starch granule displayed an equal ΔH(g) value to that of non-waxy rice starches with a lower relative crystallinity but had a larger starch granule.[Citation32] In addition, the presence of high amylose could enhance the degree of starch retrogradation due to the dispersal of structural attributes of amylose (i.e., long, linear chains with a few long-chain branch points) in starch gel.[Citation9,Citation29] Starch gel containing high amylose can easily reaggregate and immediately form a network structure of retrograded starch gel upon cooling.[Citation9,Citation29] Accordingly, both the retrograded starch gel of low-amylose EDPLK, EL, JL, LN, and TD rice and the retrograded starch gel of very low-amylose TW rice showed the highest percentage of retrogradation (R%), while the lower %R was observed in waxy rice starches (). The retrograded starch gel requires a high temperature and absorbs high thermal energy to completely melt its newly formed crystal structures.[Citation9,Citation29] This is consistent with our results showing that the low-amylose EDPLK rice starch displays the highest Tp(r), Tc(r), and ∆T(r). Also, the low-amylose LN and JL rice starches showed the highest Tp(r) and Tc(r), respectively, while the waxy ND rice starch showed the lowest Tc(r) (). The low-amylose rice EDPLK, EL, JL, LN, and TD starches and the very low-amylose rice TW starch also displayed the highest ΔH(r) value, but the lowest ΔH(r) value was observed in the waxy rice KK and KP starches (). Interestingly, the low-amylose JD rice starch showed the lowest ΔH(r) and R% values, similar to the waxy rice KK and KP starches (), even though the JD starch possessed a substantially higher amylose content than KK and KP starches (). This observation might be explained by the proportions of amylopectin long and short branch chains, which could affect the reassemble and recrystallization of dispersed starch chains within the gel matrix during the cooling process.[Citation9,Citation16] Accordingly, our recent study showed that low amylose starches obtained from Thai commercial rice cultivars had an equal ΔH(r) value to that of intermediate amylose rice starches due to the presence of a higher proportion of amylopectin long B3+ chains (DP ≥ 37).[Citation16]
Table 3. Starch gelatinization properties of 19 Thai indigenous rice varieties.
Table 4. Starch retrogradation properties of 19 Thai indigenous rice varieties.
Starch pasting properties: The pasting profile of starch slurry displays structural changes of the granules upon heating and cooling under the excess of water and shearing force. This process includes hydrogen bond breakage when heated, granular swelling upon water absorption, amylose molecule leaching, granule breakdown, and dispersed molecular retrogradation.[Citation29] Here, these phenomena were monitored via an RVA-simulated rheometer.[Citation18] Overall, the indigenous rice starches can be separated into two major groups based on their pasting profiles: Group 1, consisting of ET, KK, KP, KPN, LT, MP, ND, NDD, NN, PA, SPT, TW, and UN; and Group 2, consisting of EDPLK, EL, JD, JL, LN, and TD (). The rice starches from the first group had lower pasting temperature (PTemp), peak time (Ptime), final viscosity (FV), and setback (SB) than rice starches from the second group ( and ). Interestingly, the Group 1 starches are all waxy rice starches, which have a lower amylose content than the non-waxy rice starches from the Group 2 (). Amylose and amylose-lipid complexes prevent swelling and preserve the structural integrity of the swollen granules.[Citation28] Meanwhile, the leached amylose molecules rapidly reassemble to build an amylose junction zone when the starch paste begins to cool, raising the FV and SB values of the starch gel.[Citation28] Accordingly, the waxy rice starches showed a rapid swelling (lower Ptemp and Ptime) and a slow reaggregation of dispersed starch chains (lower FV and SB) than the non-waxy rice starches, which contain higher amylose content and the presence of amylose-lipid complexes (). Interestingly, the waxy rice NDD starch demonstrated the highest peak viscosity (PV) and breakdown (BD) values (). It is suggested that the NDD starch granules with the highest relative crystallinity can withstand heat and mechanical shear, swell to a great extent (high PV), and subsequently undergo a substantial loss in viscosity when the swollen granules disintegrate (high BD) ().[Citation28]
Table 5. Starch pasting properties of 19 Thai indigenous rice varieties.
Multivariate analysis
PLS-DA is a multivariate dimensionality-reduction algorithm, which reduces variance within groups to maximize the difference between groups.[Citation19,Citation20] The originally measured variables are dimensionally condensed into a smaller set of new variables termed “scores,” which are projected onto the two-dimensional (2D) plots displaying the first two principal components (PCs).[Citation19] Here, the PLS-DA approach is employed to maximize the separation between the varieties and to identify the starch compositional, structural, or physicochemical variables discriminating between these varieties. The results revealed that six non-waxy rice genotypes, including EDPLK, EL, JD, JL, LN, and TD, are clearly isolated from the 13 waxy rice genotypes, including ET, KK, KP, KPN, LT, MP, ND, NDD, NN, PA, SPT, TW, and UN. The placement of the non-waxy rice group along the PC 2 was attributed to their larger amount of amylose together with higher SB and FV values (). In addition, the Variable Importance in Projection (VIP) plot, which summarizes the importance of the top 15 variables on the PLS-DA model[Citation20] is displayed in . The variables with VIP scores higher than 1.0 are pertinent for modeling explanation.[Citation20] Therefore, the grain compositional variables (i.e., amylose and reducing sugar contents), the starch structural characteristics (i.e., proportions of C-type and A-type granules and d(0.5)), and the starch physicochemical features (i.e., SB, swelling power, and FV values) were the key parameters discriminating these 19 Thai indigenous rice starches (). Notably, the amylose content displayed the highest VIP score (), suggesting its most important role in starch phenotypic discrimination among these indigenous rice varieties.
Figure 4. Partial-Least Squares Discriminant Analysis (PLS-DA) of measured variables. PLS-DA (a) score plot (b) loading plot and (c) VIP plot of all variables measured in this study were shown. Each of the biological replicates was individually projected onto the plane of principal components (PC) 1 and 2. The first two highest variances were observed in the PC2 and PC1, with 85.4% and 10.8% of total variance, respectively. The confidence ellipses occupy the 95% confidence intervals of the data. The colored boxes shown on Figure 4C indicate the relative values of the corresponding parameters in each rice variety.

Correlation analysis between starch compositional and structural features and starch physicochemical properties
Besides the variations in the starch properties, understanding the relationships between the starch compositional and structural characteristics and physicochemical properties of all 19 rice varieties are essential for their uses as cooked rice and as a biopolymer in food and non-food industries.[Citation16] The Pearson correlation coefficients were calculated between the starch parameters of all indigenous rice varieties (). A significant negative correlation (r = −0.816, p ≤.01) between amylose content and swelling power (SP) was observed (). The presence of amylose could impede the absorption of water into the granule amorphous and crystalline regions upon heating, resulting in a decline in the swelling of starch granules.[Citation9,Citation16,Citation28] In addition, a strong positive correlation between the relative crystallinity (RC) and SP (r = 0.786, p ≤.01) was observed (). Previous reports noted that swelling is a property of amylopectin branch chains, which are the major components of the granule crystalline regions.[Citation9,Citation16] Starch granules with a high degree of crystallinity had the ability to absorb and associate with water via hydrogen bonding and swell during heating under excess water conditions.[Citation10,Citation16]
Table 6. Pearson correlation coefficients between starch chemical composition and molecular structure and starch physicochemical properties of 19 Thai indigenous rice varieties.
Moreover, the amylose content was positively correlated with To(g) and Tp(g) (r = 0.790, p ≤.01 and r = 0.742, p ≤.01, respectively), but negatively correlated with ΔT(g) (r = −0.707, p ≤.01), which is consistent with previous reports.[Citation16,Citation32] Amylose hinders the swelling of starch granules and the crystalline collapsing of swollen granules by forming helical complexes with lipids.[Citation16,Citation32] Consequently, starch granules containing a high amylose content require high temperatures (e.g., To(g) and Tp(g)) to swell and completely melt.[Citation16,Citation32] Additionally, the presence of amylose and amylose-lipid complexes could inhibit granular swelling, amylose leakage, amylopectin solubilization, and the formation of starch paste when starch is continuously heated under the excess of water and shearing force.[Citation10,Citation16,Citation28] Hence, starch granules consisting of a high amylose content and the presence of amylose lipid complexes require a high pasting temperature (Ptemp) and long peak time (PTime).[Citation10] This is consistent with the strong positive correlations between the amylose content and Ptemp (r = 0.873, p ≤.01) and PTime (r = 0.957, p ≤.01) observed in this study (). On the other hand, RC was negatively correlated with Ptemp (r = −0.758, p ≤.01) and PTime (r = −0.920, p ≤.01) (). It was suggested that waxy rice starch granules with an absence of amylose-lipid complexes typically display a high relative crystallinity,[Citation10,Citation16,Citation28,Citation32] and would therefore swell rapidly (low Ptemp and PTime) under heat and shearing force.[Citation10] As reported in several previous works, the amylose content is one of the most important factors influencing the retrogradation and pasting properties of rice starches.[Citation9,Citation16,Citation28] Long linear amylose chains dispersing within the starch paste facilitate the formation of intra- and intermolecular interactions between starch chains via hydrogen bonds, resulting in the formation of a strongly crystallized gel network.[Citation9,Citation16] This firm gel requires a high temperature for complete dissociation (i.e., high retrogradation temperatures).[Citation10,Citation16] The gel network formation involving amylose upon cooling is mainly responsible for the final viscosity (FV) and setback (SB) values of starch paste.[Citation10,Citation16] Consistently, the amylose content was positively correlated with Tc(r), ∆T(r), FV, and SB (r = 0.858, p ≤.01, r = 0.749, p ≤.01, r = 0.856, p ≤.01, and r = 0.927, p ≤.01, respectively) (). In contrast, the RC was negatively correlated with Tc(r), FV, and SB (r = −0.771, p ≤.01, r = −0.840, p ≤.01, and r = −0.882, p ≤.01, respectively) (). The high-crystallinity rice starches, which typically contain a low amylose content,[Citation10,Citation16,Citation28,Citation32] would retrograde and recrystallize less readily during cooling and display low FV and SB.[Citation10,Citation28] Accordingly, the starch gel obtained from high-crystallinity rice starches would melt easily during heating (low Tc(r)).[Citation10,Citation16]
Conclusion
The 19 indigenous Thai rice starches had a great variation in compositional, structural, and physicochemical characteristics. Despite the similar starch content, there were significant differences in grain reducing sugar, protein, and amylose contents among these varieties. Starch granules from all varieties displayed A-type crystalline polymorph with wide variations in the proportions of C-, B-, and A-granules and relative crystallinity. The variability in amylose content contributed to their diverse physicochemical and functional properties including swelling power, solubility, gelatinization and retrogradation transition temperatures, and pasting profiles. Multivariate analysis revealed that amylose content, SB, swelling power, reducing sugar content, C- and A-type granules, d(0.5), and FV were significant in differentiating between the low-amylose rice varieties and the rest. It also suggested that amylose content was the most important variable in the starch phenotypic discrimination among these indigenous varieties. Accordingly, the amylose content was the major factor showing significant correlations with the physicochemical properties of rice starch. The swelling power and ∆T(g) decreased, while To(g), Tp(g), Tc(r), ∆T(r), Ptemp, Ptime, FV, and SB values increased with increasing amylose content. Taken together, the starch features of these 19 Thai indigenous rice varieties were characterized for the first time. With a wide variation of the starch properties, they could promote local food sovereignty as alternative sources of biopolymers, functional food ingredients, or raw materials for rice starch applications in both food and non-food industries.
Abbreviation
Breakdown | = | BD |
Conclusion temperature | = | Tc |
Differential scanning calorimetry | = | DSC |
Final viscosity | = | FV |
Gelatinization enthalpy | = | ∆H(g) |
Gelatinization temperature ranges | = | ΔT |
Holding strength | = | HS |
Nitrogen | = | N |
Onset temperature | = | To |
Partial-least squares discriminant analysis | = | PLS-DA |
Peak temperature | = | Tp |
Pasting temperature | = | PTemp |
Peak temperature | = | Tp |
Peak time | = | PTime |
Peak viscosity | = | PV |
Rapid Visco Analysis | = | RVA |
Retrogradation enthalpy | = | ∆H(r) |
Setback | = | SB |
Temperature range of gelatinization | = | ∆T(g) |
Temperature range of retrogradation | = | ∆T(r) |
X-ray Diffractometry | = | XRD |
Supplemental Material
Download MS Word (3.4 MB)Acknowledgments
The authors are grateful to Mr. Theparit Pitirit and Mr. Supon Bokum for their kind assistance in protein content and granule size distribution analyses, respectively. We also thank Mr. Methani Kongracha, Mr. Yodsapat Prasert, and Miss Ladapa Wirakham for their technical assistance in grain composition and starch molecular structure analyses.
Disclosure statement
No potential conflict of interest was reported by the author(s).
Data availability statement
The data that support the findings of this study are available from the corresponding author, MT, upon reasonable request.
Supplementary material
Supplemental data for this article can be accessed online at https://doi.org/10.1080/10942912.2023.2293465.
Additional information
Funding
References
- Thitisaksakul, M.; Sangwongchai, W.; Mungmonsin, U.; Promrit, P.; Krusong, K.; Wanichthanarak, K.; Tananuwong, K. Granule Morphological and Structural Variability of Thai Certified Glutinous Rice Starches in Relation to Thermal, Pasting, and Digestible Properties. Cereal Chem. 2021, 98(3), 492–506. DOI: 10.1002/cche.10389.
- Suebpongsang, P.; Ekasingh, B.; Cramb, R. Commercialisation of Rice Farming in Northeast Thailand. In White Gold: The Commercialisation of Rice Farming in the Lower Mekong Basin; Cramb, R., (Ed.); Palgrave Macmillan: Singapore, 2020; pp. 39–68. DOI: 10.1007/978-981-15-0998-8_2.
- TREA. 2022. Rice Production Forecast 2017-2022. (Online) TREA Rice Production. http://www.thairiceexporters.or.th/production.htm (Accessed 8 8 2022).
- Rerkasem, B. The Agroecosystem of Thai Rice: A Review. CMU. J. Nat. Sci. 2015, 14(1), 1–21. DOI: 10.12982/cmujns.2015.0068.
- Sangwongchai, W.; Tananuwong, K.; Krusong, K.; Thitisaksakul, M. Y. Yield, Grain Quality, and Starch Physicochemical Properties of 2 Elite Thai Rice Cultivars Grown Under Varying Production Systems and Soil Characteristics. Foods. 2021, 10(11), 2601. DOI: 10.3390/foods10112601.
- Oppong, D.; Panpipat, W.; Chaijan, M. C. Physical, and Functional Properties of Thai Indigenous Brown Rice Flours. PLoS. One. 2021, 16(8), e0255694. DOI: 10.1371/journal.pone.0255694.
- Rerkasem, B. The Rice Value Chain: A Case Study of Thai Rice. CMU. J. Soc. Sci. Humanity. 2017, 4(1), 1–26. DOI: 10.12982/CMUJASR.2017.0001.
- Patindol, J. A.; Siebenmorgen, T. J.; Wang, Y. J. Impact of Environmental Factors on Rice Starch Structure: A Review. Starch/Stärke. 2015, 67(1–2), 42–54. DOI: 10.1002/star.201400174.
- Tangsrianugul, N.; Wongsagonsup, R.; Suphantharika, M. Physicochemical and Rheological Properties of Flour and Starch from Thai Pigmented Rice Cultivars. Int J Biol Macromol. 2019, 137, 666–675. DOI: 10.1016/j.ijbiomac.2019.06.196.
- Kong, X.; Zhu, P.; Sui, Z.; Bao, J. Physicochemical Properties of Starches from Diverse Rice Cultivars Varying in Apparent Amylose Content and Gelatinisation Temperature Combinations. Food Chem. 2015, 172, 433–440. DOI: 10.1016/j.foodchem.2014.09.085.
- Zhou, T. Y.; Li, Z. K.; Li, E. P.; Wang, W. L.; Yuan, L. M.; Zhang, H.; Liu, L. J.; Wang, Z. Q.; Gu, J. F.; Yang, J. C. Optimization of Nitrogen Fertilization Improves Rice Quality by Affecting the Structure and Physicochemical Properties of Starch at High Yield Levels. J. Integr. Agric. 2022, 21(6), 1576–1592. DOI: 10.1016/S2095-3119(21)63678-X.
- Rerkasem, B.; Rerkasem, K. Agrodiversity for In Situ Conservation of Thailand’s Native Rice Germplasm. Cmu. J. 2002, 1(2), 129–148.
- Khotasena, S.; Sanitchon, J.; Chankaew, S.; Monkham, T. The Basic Vegetative Phase and Photoperiod Sensitivity Index as the Major Criteria for Indigenous Upland Rice Production in Thailand Under Unpredictable Conditions. Agron. 2022, 12(4), 957. DOI: 10.3390/agronomy12040957.
- Wongsa, P.; Landberg, R.; Rattanapanone, N. Chemical Compositions and Metabolite Profiling of Rice Varieties from Chiang Rai Province Thailand. Chiang Mai J. Sci. 2018, 45, 2703–2714.
- Ponjanta, J.; Chomsri, N. O.; Meechoui, S. Correlation of Pasting Behaviors with Total Phenolic Compounds and Starch Digestibility of Indigenous Pigmented Rice Grown in Upper Northern Thailand. Funct. Foods Health Dis. 2016, 6(3), 133–143. DOI: 10.31989/FFHD.V6I3.231.
- Sangwongchai, W.; Tananuwong, K.; Krusong, K.; Natee, S.; Thitisaksakul, M. Starch Chemical Composition and Molecular Structure in Relation to Physicochemical Characteristics and Resistant Starch Content of Four Thai Commercial Rice Cultivars Differing in Pasting Properties. Polym. 2023, 15(3), 574. DOI: 10.3390/polym15030574.
- AOAC. AOAC Official Method 920.87 Protein (Total) in Flour, In Official Methods of Analysis AOAC International, 16th; Cunniff, P., Ed.; AOAC International: 481 North Frederick Avenue, Suite 500, Gaithersburg, MD 20877-2417, USA, 1999; pp. 12–13.
- Thitisaksakul, M.; Tananuwong, K.; Shoemaker, C. F.; Chun, A.; Tanadul, O. U. M.; Labavitch, J. M.; Beckles, D. M. Effects of Timing and Severity of Salinity Stress on Rice (Oryza Sativa L.) Yield, Grain Composition, and Starch Functionality. J. Agric. Food. Chem. 2015, 63(8), 2296–2304. DOI: 10.1021/jf503948p.
- Sangwongchai, W.; Krusong, K.; Thitisaksakul, M. Salt Tolerance at Vegetative Stage is Partially Associated with Changes in Grain Quality and Starch Physicochemical Properties of Rice Exposed to Salinity Stress at Reproductive Stage. J. Sci. Food Agric. 2022, 102(1), 370–382. DOI: 10.1002/jsfa.11367.
- Worley, B.; Powers, R. Multivariate Analysis in Metabolomics. Curr. Metabolomics. 2013, 1(1), 92–107. DOI: 10.2174/2213235X11301010092.
- Chen, X.; Chen, M.; Lin, G.; Yang, Y.; Yu, X.; Wu, Y.; Xiong, F. Structural Development and Physicochemical Properties of Starch in Caryopsis of Super Rice with Different Types of Panicle. BMC. Plant Biol. 2019, 19(1), 1–15. DOI: 10.1186/s12870-019-2101-7.
- Martin, M.; Fitzgerald, M. Proteins in Rice Grains Influence Cooking Properties! J. Cereal Sci. 2002, 36(3), 285–294. DOI: 10.1006/jcrs.2001.0465.
- Furukawa, S.; Tanaka, K.; Masumura, T.; Ogihara, Y.; Kiyokawa, Y.; Wakai, Y. Influence of Rice Proteins on Eating Quality of Cooked Rice and on Aroma and Flavor of Sake. Cereal Chem. 2006, 83(4), 439–446. DOI: 10.1094/CC-83-0439.
- Juliano, B. R. Overview. In Encyclopedia of Food Grains; Wrigley, C., Corke, H., Seetharaman, K. Faubion, J., (Eds.); Academic Press: Oxford, UK, 2016; pp. 125–129. DOI: 10.1016/B978-0-12-394437-5.00015-2.
- Wani, A. A.; Singh, P.; Shah, M. A.; Schweiggert‐Weisz, U.; Gul, K.; Wani, I. A. Rice Starch Diversity: Effects on Structural, Morphological, Thermal, and Physicochemical Properties—A Review. Compr. Rev. Food Sci. Food Saf. 2012, 11(5), 417–436. DOI: 10.1111/j.1541-4337.2012.00193.x.
- Singh, S.; Singh, N.; Isono, N.; Noda, T. Relationship of Granule Size Distribution and Amylopectin Structure with Pasting, Thermal, and Retrogradation Properties in Wheat Starch. J. Agric. Food. Chem. 2010, 58(2), 1180–1188. DOI: 10.1021/jf902753f.
- Tang, H.; Ando, H.; Watanabe, K.; Takeda, Y.; Mitsunaga, T. Physicochemical Properties and Structure of Large, Medium and Small Granule Starches in Fractions of Normal Barley Endosperm. Carbohydr Res. 2001, 330(2), 241–248. DOI: 10.1016/s0008-6215(00)00292-5.
- Chung, H. J.; Liu, Q.; Lee, L.; Wei, D. Relationship Between the Structure, Physicochemical Properties and In Vitro Digestibility of Rice Starches with Different Amylose Contents. Food Hydrocoll. 2011, 25(5), 968–975. DOI: 10.1016/j.foodhyd.2010.09.011.
- Srichuwong, S.; Jane, J. I. Physicochemical Properties of Starch Affected by Molecular Composition and Structures: A Review. Food Sci. Biotechnol. 2007, 16(5), 663–674.
- Wanichthanarak, K.; Thitisaksakul, M. ThRsdb: A Database of Thai Rice Starch Composition, Molecular Structure and Functionality. Database. 2020, 2020. DOI: 10.1093/database/baaa068.
- Koch, K.; Jane, J. L. Morphological Changes of Granules of Different Starches by Surface Gelatinization with Calcium Chloride. Cereal Chem. 2000, 77(2), 115–120. DOI: 10.1094/CCHEM.2000.77.2.115.
- Zhu, D.; Zhang, H.; Guo, B.; Xu, K.; Dai, Q.; Wei, C.; Zhou, G.; Huo, Z. Physicochemical Properties of Indica-Japonica Hybrid Rice Starch from Chinese Varieties. Food Hydrocoll. 2017, 63, 356–363. DOI: 10.1016/j.foodhyd.2016.09.013.