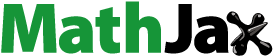
ABSTRACT
Finite Element Model simulations are widely used to validate experimental and field observations. Out of these, modelling and comparing the stability of dams under various loading conditions presents significant challenges for researchers and practitioners, none more than the concrete-faced rockfill dams that are present in Australia (e.g. Cethana dam) and around the globe. A review shows that the construction sequence of such dams has not been incorporated in the initial configuration of concrete-faced rockfill dams. In this research, static analysis has been conducted for 2D and 3D finite element models of the Kotmale concrete-faced rockfill dam (CFRD) with and without the construction stage. Both 2D and 3D analysis of the case study dam using MIDAS FEA NX software and static analysis have been conducted. A linear elastic material model and a nonlinear material model (Duncan and Chang EB Model) were used to model the rockfill material. The resulting deformations were compared with the in-situ measurements to identify the accuracy of the developed cases. Results show that construction stage inclusion and the nonlinear material model agree well with the in-situ measurements, warranting the need for such advancements in Finite Element Analysis. Such an inclusion can model future settlement behaviour reasonably well, which can lead to the development of appropriate management measures.
1. Introduction
Finite element simulations are widely used for the design and construction of concrete-faced rockfill (CFR) dams and to assess the performance of existing CFR dams for extreme loading conditions such as earthquakes using a variety of techniques. Zhang et al. (Citation2004) developed a 2D finite element model incorporating 15 stages in the construction of the Tianshengqiao-I dam in China to assess the deformation under nonlinear static analysis. Özkuzukiran et al. (Citation2006) developed a 2D model consisting of 5 m construction layers of rockfill of the Kurtan dam in Turkey to assess the settlement behaviour using a hardening soil model to depict the nonlinear inelastic and stress dependency of rockfill. Zhang and Zhang (Citation2009) focused on the soil-structure interface using a 3D finite element model of the Zipingpu CFR dam located in China. Bayraktar et al. (Citation2009) developed a 2D finite element model to conduct linear and nonlinear time history analysis of the Torul dam located in Turkey. Dam-soil interaction and dam-reservoir interaction were highlighted in this research; however, the construction sequence has not been incorporated in the FE model. The response surface method together with the welded and friction contacts was included in the developed 2D dam model of the Torul dam in Turkey by Kartal et al. (Citation2010) and the seismic response was analysed. Bayraktar et al. (Citation2011) used a similar finite element model to analyse the effect of the slab-rockfill interaction during an earthquake using friction contacts and welded contacts. A similar type of assessment was conducted by Kartal and Bayraktar (Citation2013) using a 2D finite element model where the linear and nonlinear behaviour of the structure was analysed during an earthquake and the results were compared. Kartal and Bayraktar (Citation2014) continued the assessment with a nonlinear response of rockfill material and the tensile stresses generated were investigated for dynamic analysis of the Torul dam. In these analyses, the stagewise construction has not been incorporated in the finite element simulations, and the selection of a 2D model has not been justified. Seiphoori et al. (Citation2011) developed a typical 100 m high 3D dam model to assess the dam-foundation effect for P, SV and SH waves acting on the structure during an earthquake. The dam body construction was stimulated in five stages; moreover, the scaled boundary finite element method has been followed in the research, and load failures of construction joints and the opening movements are identified through the analysis. Messochora dam, which is located in a narrow canyon in Greece, was selected by Dakoulas (Citation2012) to develop a 3D finite element model to obtain the settlement effect due to dynamic excitation. The stage construction, impounding and creep settlement were considered. Fallah and Wieland (Citation2013) developed a 2D dam model of the Koman dam located in Albania consisting of 13 stages of gravity loads to depict the construction behaviour and the peak settlement was analysed in case of seismic loading under different peak ground accelerations. Zou et al. (Citation2013) developed a 3D finite element model of the Zipingpu CFR dam located in China to numerically analyse the effect during the Wenchuan earthquake. The construction sequence of the face slab has been taken into consideration; however, the construction sequence of the dam body has not been implemented in the FE model. Ghafari et al. (Citation2015) analysed the Chenareh Rockfill Dam in Iran to investigate the arching effect during construction, while Zhang et al. (Citation2017) comprehensively analysed the tensile stress distribution in the face slab during an earthquake using a 3D finite element model including the construction sequence in 20 stages.
In the literature, finite element models of CFR dams have been incorporated in material parameter optimisation as well. Yu et al. (Citation2007) conducted a back analysis using artificial neural networks and optimising algorithms. 2D finite element models compromising the construction sequence of Maopingxi dam and Gongboxia CFR dam both located in China were developed to validate the back analysis results. The time-dependent settlement of the Shuibuya CFR dam was analysed by Zhou et al. (Citation2011) using a 2D finite element model depicting 12 layers of rockfill material. Back analysis was conducted using a genetic hybrid algorithm to predict the settlement for the next 3 years. Jia and Chi (Citation2015) developed a 3D finite element model of the Malutang II dam in China with 20 construction layers of rockfill material to conduct back analysis using the parallel mutation particle swarm optimisation method. Response surface method and the genetic optimisation were adopted by Guo et al. (Citation2016) for the back analysis of material parameters of the creep model and the Duncan and Chang EB model using a developed 3D finite element model of Pubugou dam. The deformations during the dam construction have been taken into account; however, the implementation of the construction sequence in the FE model has not been discussed.
In the literature discussed above, it can be noted that the construction sequence of the rockfill material has not been incorporated by some researchers. However, in the research where stage-wise construction has been adopted in the developed FE simulations, the selection of the number of stages for the analysis and the significance of incorporating the construction sequence have not been explicitly presented. Furthermore, it can be noted that 2D and 3D models have been utilised for analysis however, justification in selecting the models for the analysis has not been discussed.
Therefore, this research aims to identify the significance of adopting the construction sequence through construction stage analysis in the finite element simulations to accurately depict the initial behaviour of concrete-faced rockfill dams by considering the material and the geometric nonlinearity. Furthermore, the variation of the results (deformations) of the concrete-faced rockfill dams obtained through 2D and 3D finite element models is assessed to emphasise the accuracy of adopting either model for the analysis. Similarly, the variation of the results obtained through nonlinear material models and linear elastic material models (for the rockfill material) is assessed to identify the significance. Finally, the research investigates the variation of stresses generated in the concrete slab through the construction sequence. For this purpose, the Kotmale Dam in Sri Lanka was selected as a case study.
Initially, a 2D finite element model was developed which includes the foundation, rockfill layers, transition layers, drainage layers, concrete layer and the toe weights. The construction sequence was embedded into the developed finite element model through the construction stage analysis. Sensitivity analysis was conducted to deduce the optimum mesh size, and a static analysis was conducted considering the construction stages and without considering the construction stages. The optimised mesh was used to develop the 3D dam model. Contact surfaces were introduced between the rockfill and the foundation, the rockfill and the concrete surface to assess the relative movements. The two main types of material at CFR dams are concrete and rockfill. The concrete was assumed to be linear elastic, while both linear and nonlinear models were adopted for rockfill material.
2. Material models
The main components of the CFR dams are the rock fill and the concrete slab. Since the rockfill material is nonlinear and pressure-dependent, different material models have been used to depict the material behaviour. Although the Duncan and Chang EB model is widely used by Zhang et al. (Citation2004), Yu et al. (Citation2007) and Zhou et al. (Citation2011), the Chang-Duncan model (Zhang, Wang, and Shi Citation2004), modified ideal model and generalised plasticity models (Xu, Zou, and Liu Citation2012) have also been used for this purpose as well. For this research, the Duncan and Chang EB model has been used due to its simplicity and accuracy in predicting rockfill behaviour. To model the concrete material, linear elastic material models have been used in the aforementioned literature. In this research, a linear elastic model has been adopted to depict concrete behaviour.
2.1. Duncan and Chang EB model
To predict the nonlinear elasticity behaviour of rockfill material, the Duncan and Chang model is commonly adopted (. Hook’s law and Mohr-Coulomb’s failure criteria ( have been used to develop the stress-strain behaviour of the rockfill material.
The above equation (Equation 1) defines the hyperbolic stress–strain curve where is the major principal stress,
is the minor principal stress and
is the corresponding strain. Curve fitting parameters a and b are based on the rockfill material property, where a is inversely proportional to the initial stiffness (
which is defined through EquationEquation 2
(2)
(2) . The parameter b is inversely proportional to the ultimate deviatoric stress.
K is the modulus number, is the atmospheric pressure, and n is defined as the exponent related to the confining pressure on the initial modulus.
2.2. Mohr-coulomb failure criteria
The failure surface is defined using the Mohr circle. As shown in cohesion (c) and the friction angle define the failure surface while a relationship between the confinement stress (
) and the deviator stress at the failure can be obtained using EquationEquation 3
(3)
(3) .
Ultimate deviatoric stress is defined from the Duncan and Chang EB model and the failure deviatoric stress is defined using the Mohr coulomb model. Therefore, a failure ratio is defined as per EquationEquation 4(4)
(4)
Tangent stiffness is defined using the integration of the Duncan and Chang curve function which can be written as EquationEquation 5(5)
(5) .
Bulk modulus (B) depends on the confining stress as shown in EquationEquation 6(6)
(6) .
The variation of the Bulk modulus with Poisson’s ratio is defined as follows.
The material parameters of the Duncan and Chang EB model are K, , c,
, n, m,
. The values for these parameters can be obtained through laboratory tests and optimisation methods such as displacement-based back analysis. In this research, the material parameters adopted by Zhang et al. (Citation2004) and Yu et al. (Citation2007) have been used for the developed finite element model.
3. Developing finite element model
3.1. Geometric model development
Kotmale Dam is a 95 m high concrete face rockfill dam marking the origin of the cascade dam system in the Mahaweli River Basin of Sri Lanka. The dam is located upstream of major cities in the central highlands in Sri Lanka. Therefore, a breach in the dam could be catastrophic to the region. The CFR Dam spans across the Kotmale Oya with a dam length of 800 m bearing a reservoir capacity of 172.6 MCM. As shown in , out of the entire volume of material, Rockfill is significant in CFRDs. To reduce the seepage, a concrete face is introduced . In between the rockfill and the concrete layer, there exist two transition layers to provide good contact. The drainage layers provide a drainage path to the seepage water thus protecting the rockfill material from getting washed off. Toe weights provide stability to the dam and the concrete grouting reduces the cavities in the foundation rock. Under the Kotmale Dam, there is a Gneiss layer, and below that there is a limestone layer.
As mentioned in the previous section Duncan and Chang EB material model is used in the finite element model to depict the rockfill material behaviour. The material properties selected for this study are given in . The material properties are consistent with the material parameters available in the literature for rockfill material (Zhou, Hua et al. Citation2011) and Yu, Zhang et al. (Citation2007) since the rockfill material has similar features. The material used for the two transition layers (transfer layers) and the drainage layer are different from the core rockfill material therefore different parameter values are obtained for each layer as shown in . Furthermore, the material behaviour of the concrete and the foundation material is assumed to be linear elastic in the developed finite element model. gives the material parameters used for the linear elastic model. The values are derived using the experimental data obtained during the construction of the Kotmale Dam.
Table 1. Material parameters of the Duncan and Chang EB model.
Table 2. Material parameters for linear elastic material.
3.2. Two-dimensional finite element model development
Initially, a 2D finite element model was developed using MIDAS FEA NX finite element software. Foundation layers, rockfill material, two transition layers, concrete layer, drainage layer, toe weights and concrete grouting are included in the finite element model. As shown in , fixed boundary conditions were used at the bottom, at the left and the right ends of the foundation.
In the 2D dam model, plain strain elements have been used. Developing the finite element mesh is crucial since the results can vary when the mesh size is changed. Therefore, to avoid these conflicts, it is required to conduct a proper mesh sensitivity analysis and select the ideal mesh size. Apart from the accuracy, the computational time is also critical since our ultimate objective is to develop an efficient 2D finite element model. Quadrilateral elements were mostly used in the finite element mesh, and the aspect ratio was kept below 2 for 99% of the elements.
3.3. Three-dimensional finite element model development
The 3D finite element model was developed using MIDAS FEA-NX software as shown in . The rock fill layers, the transition layers, the concrete layer and the toe weights were modelled using 8-node hexahedron solid elements. For the foundation and the embankments, 8-node hexahedron solid elements and 6-node pentahedron solid elements were used. The foundation and the embankments were modelled up to 1.5 times the dam height and the same ratio was used when selecting the upstream and the downstream length to be modelled. The embankments and the foundation were fixed, and contact surfaces were introduced between the rockfill material and the foundation, the concrete layer and the transition layers. The total number of nodes generated in the developed finite element model is 1,059,614.
General contact surfaces and welded contact surfaces are used in the finite element model. General contacts allow a relative deformation between the defined surfaces, whereas welded contacts do not allow a relative movement which can be also defined as fixed connections. For the initial analysis, between the foundation and the rockfill layers and between the transition layers and the concrete face, node-to-node connection was maintained (welded contacts). A contact surface was then introduced between the layers (between foundation and rockfill layers, between concrete face and transition layers) with a friction coefficient of 0.7 to analyse the relative movement between these faces.
3.4. Construction sequence of rockfill material
Since the typical practice in the construction of concrete-faced rockfill dams is to construct the rockfill body in layers, the construction sequence should be considered when conducting the analysis. When constructing the layers, the rockfill material is added and then compacted in layers according to the design configuration. The strain will be accumulated in each construction layer when the above layer is added. It is important to note that the rockfill material is a pressure-dependent material; therefore, it is very important to consider the confinement pressure imposed by other layers during construction to numerically predict the deformation more accurately. Therefore, it is crucial to correctly depict the actual construction stages in the finite element model.
Initially, the analysis was conducted using 20 construction layers of rockfill material as per design configuration. When deciding the number of layers to be modelled, the actual construction and the computational time should be considered. The optimum number of layers that should be used is always a doubt in the developed finite element model. To address this issue, the Kotmale Dam model was analysed for 15, 20 and 25 layers of rockfill, and the results were compared with the in-situ measurements.
For the 2D model, initially, the foundation was added followed by the transition layers, and the concrete layers were added after the rockfill layers. Finally, the water pressure was added to the concrete layer and the toe weight. In the 3D model, the foundation and the embankments were added first. The construction stages of the rockfill were followed by the transition layers and the toe weights. As the final step, the water pressure was added similar to the 2D model.
In Midas FEA NX software, the construction stages can be defined, and the analysis can be conducted accordingly for both 2D and 3D models. The typical stagewise construction is defined and the calculations take place accordingly where the deformation of the initial stage is recorded and is added as a force when the next layer is added, similarly the analysis is repeated for all the stages thus the strain accumulation at the lower level of the rockfill is considered.
3.5. Analysis cases
The 2D model is analysed under different cases as shown in . The construction sequence is considered in models 1 and 2 and the rockfill material behaviour is assumed to be nonlinear in the 1st model and the 2nd model, linear elastic behaviour is assumed. For the 3rd and the 4th case, the analysis is conducted without considering the construction stages where the gravity loads of the entire structure are considered at once followed by the water pressure. Similarly, the rockfill material behaviour was considered to be non-linear in the 3rd model, and in the 4th model, the material behaviour of the rockfill is taken as linear elastic.
Table 3. Analysis cases for 2D model.
A similar type of analysis is conducted for the 3D dam model, where the analysis was conducted under four cases as given in .
Table 4. Analysis cases for 3D model.
3.6. Dam monitoring
Dam monitoring instruments are located at the Chainage 400 cross section as shown in . Settlement gauges are installed in the rockfill and the concrete layers, while the horizontal movement of the dam is measured using electromagnetic probes. Dam monitoring is done at three levels as shown in .
The vertical deformation measuring instruments are located at specific levels during the construction. Therefore, the readings obtained just after the construction depict the deformation of the material during the construction period. However, it is important to note that lateral deformation during construction cannot be obtained since the initial recording is obtained after the completion of the construction. The initial reading can be deducted from the observed value after impounding, which gives the lateral deformation due to the water body. Therefore, for comparison purposes, the lateral deformation resulting from the water pressure is used.
4. Results and discussion
4.1. Introduction
To assess the importance of the construction stage analysis of concrete-faced rockfill dams, 2D and 3D finite element models of the Kotmale Dam were analysed under different cases introduced in the previous section. Furthermore, the rockfill material was modelled using non-linear material models and linear elastic material models under different cases as defined in the previous section.
The in-situ measurements of the dam deformations were used to validate the finite element model results. The dam settlements measured at the chainage 400 m cross-section of the dam in three different levels located at 35 m, 55 m and 75 m from the dam base are used for the comparison. When the deformations at certain levels (along the horizontal axis) of the dam are considered, 35 m and 55 m levels are considered for the comparison. However, for the deformation profiles along the dam centre axis (vertical axis), measurements at 35 m, 55 m and 75 m levels are considered.
Finite element analysis gives the accumulated horizontal deformation. Therefore, the difference between lateral deformation obtained after the completion of the structure and after impounding (adding the water pressure) is compared with the in-situ measurements.
4.2. Sensitivity analysis results
For the 2D finite element model, a sensitivity analysis was conducted for different mesh sizes. shows the variation of the maximum settlement of the dam with different mesh sizes. Furthermore, the total number of elements generated, the number of elements having more than 1.5 and 2 aspect ratio and the computational time are presented in .
Table 5. Comparison of mesh quality and computation time for different element sizes.
According to , minor variations to the resulting maximum settlement can be observed when the mesh size is varied from 1 m to 5 m. However, significant variations are observed when the mesh size is further increased. The optimum mesh size can be identified using the data presented in . When the element size is 3 m, the computational time is reasonable and the elements less than an aspect ratio of 1.5 is less than 2% of the total elements. Therefore, the optimum mesh size was considered to be 3 m. The developed 2D model with a mesh size of 3 m is used to derive the 3D model such that the dimensions of the elements of the 3D model are kept below 3 m as well.
4.3. Construction stage analysis results
Kotmale Dam was constructed by adding the rockfill material layer-wise and compacting it according to the design configuration. For the mesh sensitivity analysis, 20 construction layers of rock fill were selected according to the design configuration. In order to identify the ideal number of layers, 2D finite element models were developed with 15, 20 and 25 rockfill layers and then the static analysis was conducted to compare the deformations. The vertical settlement at selected points and the horizontal settlements of the upstream and downstream faces were compared for the different cases. It is important to note that the mesh size was maintained at 3 m for the developed models.
illustrates the vertical deformation at level 35 m from the dam base. The values obtained without construction stage analysis show a large variation to the in-situ measurements, whereas when more layers are introduced the results agree well with the actual readings. At the point −52.1 m from the dam axis, the results obtained through different finite element analysis cases show deviations of more than 40% from the in-situ values as shown in . Towards the downstream, the results agree when the layer-wise construction is considered. At 0 axis, the deviations are less than 10% when 15 and 25 layers were used; however, when 20 layers are considered, the variation is almost 0%. Overall, the deviations when using different numbers of layers are similar, but without construction stages, the deviation is significantly high.
illustrates the vertical deformation at the level 55 m from the dam base. Similar to the previous discussion, when the construction stages are defined using one layer (without construction stages) the percentage deviations to the in-situ values are more than 100% at the three points as shown in . At the three locations, when the number of layers is varied, the results do not vary significantly compared to the analysis without the construction stages. illustrate the horizontal deformation of the rockfill material in the upstream and downstream slope, respectively, obtained from the finite element analysis for different cases. The values depict the horizontal deformation of the rockfill material from the beginning of the construction until after the impounding of the dam is completed. The negative deformations at the downstream slope indicate the movement of the material during the construction towards the dam body. It can be noted that when the number of stages is varied, the variation of the horizontal deformation is not significant.
Therefore, the selection of the number of layers would be based on the vertical deformation results. Based on the results obtained for the level 35 m from the dam base, for 20 layers, the error is minimum in multiple locations; however, at level 55 m from the dam base, errors from the 15-layer analysis are lower than the results obtained from 20 to 25 layers. However, compared to the analysis without the construction sequence, the errors are significantly lower for the three cases (15, 20 and 25-layer analysis). Therefore, the use of 20 layers for the analysis could be justified.
4.4. Vertical settlement
Static analysis was conducted on the developed 2D and 3D models which consist of the optimum mesh size, containing 20 construction layers of rockfill. Analysis was conducted considering the construction stage and without the construction stage where the results obtained were compared with the in-situ measurements. In all cases, the stimulations of the rockfill material were assumed to be nonlinear, and the concrete material was considered to be linear elastic. The resulting vertical settlement contours are shown in for 2D and 3D models, respectively. The maximum deformation values obtained are presented in .
Table 6. Maximum deformations obtained through 2D and 3D models from .
illustrate the deformed configuration of the dam body at 400 m chainage when the 2D model simulates the gravity load, considering stage construction and without considering stage construction, respectively. At first glance, it can be noted that peak deformations resulted at two completely distinct locations, highlighting the importance of incorporating the stage construction simulation into the analysis. When the construction sequence is not considered, the vertical settlement is maximum at the top of the dam with a value of 2007.5 mm () and when it is considered, the maximum vertical settlement is maximum near the middle of the dam body with a value of 1045.7 mm () which is a 50% reduction compared to the first case. This phenomenon is due to the accumulation of the strain at the middle sections due to the addition and compaction of the layers above. No significant deformation can be observed in the foundation and the embankments for both cases.
illustrate the deformed configuration of the 3D finite element model under gravity loads without construction stage analysis and with construction stage analysis, respectively. Since the critical section is the chainage 400 m, the 3D model results of that section are considered for comparison. The 3D model depicts the same phenomenon observed in the 2D model where the maximum deformation location is completely different for the two scenarios. In the analysis conducted without the construction stages where the entire dam was modelled as one unit, the vertical settlement is maximum at the top of the dam with a value of 1823.3 mm (), whereas with construction stage analysis the vertical deformation maximum is closer to the centre of the dam with a value of 988.8 mm (). No deformation can be observed in the foundation and the embankments for both cases.
As mentioned in section 3.5, eight different analysis options were considered in this study to investigate the influence of material nonlinearity and the construction sequence in the dam body deformation. illustrates the variation of the vertical settlement along the dam centre axis obtained from eight different finite element models (cases mentioned in section 3.5). Furthermore, these deformed shapes are compared with the in-situ measurements.
Figure 12. Vertical settlement profile at the dam centre for different cases defined in Section 3.5.

When the construction sequence is not considered in the analysis, the vertical deformation increases towards the top of the dam from its base, but when the construction sequence is considered, the vertical deformation increases from the bottom up to the centre of the dam and then decreases towards the top of the dam. This phenomenon can be observed in both 2D and 3D models, while the in-situ measurements also have a deformation shape similar to that of the results of the analysis with construction stages. Furthermore, similar deformation patterns can be observed for rockfill dams in the literature. It must be noted that when the rockfill material behaves as linear elastic, the deformations are relatively lower than results obtained through nonlinear material models. At the base of the dam, the deformations are minimal; thus, no significant variation can be observed for different scenarios. At the top of the dam, the vertical deformation resulting in nonlinear material behaviour under the self-weight is twice that is obtained through a linear elastic model without construction stage analysis. As observed in the previous comparisons, the variation between the deformations obtained through construction stage analysis has large deviations to the results obtained without construction stage analysis. Ninety per cent variation can be observed with and without construction stage analysis when the nonlinear material models were used and a deviation of 85% can be seen for the two cases (with construction stage analysis and without construction stage analysis) when the linear elastic model was adopted in the 3D model, whereas in the 2D model the variation is 88% and 91%, respectively. At the centre of the dam, around 50% variation in the vertical deformation can be observed when the material model was varied from nonlinear to linear. The deformation variation when the construction sequence is considered and not considered is relatively lesser than the deviation at the top of the dam. The values show 25% and 15%, respectively, for the analysis with linear elastic material behaviour and nonlinear material behaviour.
As mentioned in the previous section, the in-situ settlement readings at two levels (35 m and 55 m from the dam base) of the dam are used for the comparison of 2D and 3D model simulation results obtained by implementing a nonlinear model for rockfill material behaviour in the FE simulation. In-situ readings were taken at five stations located at 35 m height above the dam base and three stations at level 55 m above the dam base. The in-situ deformation taken after the construction was compared with the numerically obtained deformations at these locations as shown in and the percentage deviation to the in-situ values is depicted in bar charts in .
illustrates the symmetric deformed shapes of the dam body resulting in 2D and 3D model simulations at 35 m level. When considering the effects of construction sequence in the analysis, the results agree well with the in-situ measurements with a maximum percentage of deviation of 10% (. However, unsymmetric deformed shapes have resulted from the finite element simulation conducted without incorporating the effects of construction sequence, and they are very much deviated from the in-situ measurements with a maximum deviation of 50%. At the upstream slope, a 24% deviation from the in-situ values is obtained from the 3D model and the deviation reduces to 16% for the 2D analysis. At the centre of the dam axis, the 3D model results (without CS) agree well with the in-situ measurements, but for the 2D analysis without construction stages, the deviation is close to 17%. Towards the downstream slope, the results show a variation of 43% for both 2D and 3D without construction stage analysis. When the construction stages are taken into account, at the upstream slope, for the 2D analysis there is a deviation of 16% from the in-situ measurements. However, with the 3D model, the results obtained show a deviation of less than 7%. Towards the centre of the dam for both cases (2D and 3D) under construction stage analysis, the deformation variation is less than 5% to the in-situ measurements. And moving towards the downstream slope, the deviation reduction to less than 10% can be observed.
illustrates the symmetric deformed shapes of the dam body resulting in 2D and 3D model simulations at the 35 m level where it can be noted that the deformation obtained without the construction stage deviates significantly from the in-situ measurements. In the 2D analysis (without Construction Stage) throughout the dam cross section the results obtained are almost two times the in-situ measurements. It can be noted in that at the upstream slope in the 3D analysis (without the Construction stage), the deviation is 20%, this increases to 50% at the centre of the dam, and towards the downstream slope, the deviation increases to almost 70%. The two cases (2D and 3D) under construction stage analysis result in deformation shapes quite similar to the in-situ measurements.
4.5. Lateral deformations
The lateral deformations are obtained through 2D and 3D model analysis where the rockfill material behaviour was considered to be nonlinear. The construction stage analysis has been incorporated, and the resulting contours are presented in , respectively. show the lateral deformation contours for the 2D model after the construction of the rockfill layers and after impounding, respectively. For the 3D model, the resulting lateral deformation contours after the construction of the rockfill layers and after impounding are shown in respectively. Maximum deformations obtained in the above-mentioned four cases are presented in .
Table 7. Lateral deformation values obtained through 2D and 3D models from .
When the lateral displacement of the 2D model is considered, a symmetric displacement shape can be observed until the completion of the rockfill layers where the maximum deformation is 173 mm at the left and right slopes. But when the transition layers, concrete layer and the water pressure are added, the deformations change as shown in . The maximum deformation of 291 mm at the left slope has moved further lower towards the dam base. At the right slope, the horizontal deformation has reduced up to −160 mm (negative value indicates deformation in the opposite direction). This phenomenon is the result of the water pressure pushing the dam in the lateral direction.
Similar to the 2D dam model, until the completion of the rockfill layers, a symmetric displacement of 167 mm can be observed in the transverse direction at level 60 m from the dam base in the 3D dam model as shown in . Once the transition layers, concrete layer and water pressure were added, the deformations varied as shown in . The maximum deformation at the left slope has increased by 108 mm, and at the right slope the lateral deformation has reduced by 35 mm. The maximum deformation is now visible at 55 m level from the dam base.
The instrument stations are located at the dam cross section at chainage 400 m. Four monitoring points are located at the level 35 m from the dam base. However, readings at three stations are obtained for the comparison. Since the lateral movement is measured using electromagnetic probes, the deformation during the construction cannot be obtained (unlike the vertical settlement gauges). As mentioned before, in-situ measurements indicate the lateral movement after the water pressure is added. Therefore, in-situ measurements of lateral deformations are compared with the lateral deformation difference obtained from the stage after the completion of the dam body and after the water pressure was added. illustrate the comparison of the lateral deformation obtained through the 3D model at 35 m and 55 m, respectively.
Figure 17. Comparison of the horizontal deformation obtained from the 3D model with the in-situ recordings.

At both levels, the deformation shapes agree well with the in-situ measurements but there are significant deviations at the upstream. At level 55 m, the variation between the analysis results and the in-situ measurements is 25% throughout the level. Towards the upstream slope, the deformation increases up to 70%. At level 35 m, at the centre line, the variation is around 35%, and towards the upstream end, the variation moves up to 50%.
Furthermore, the lateral deformation profile obtained through the 3D model at the upstream face, centreline and downstream face of the dam at the chainage of 400 m is mapped as shown in .
The maximum lateral deformation can be observed at the upstream slope and the deformation reduces significantly towards the downstream slope. This shows the material compaction towards the centre of the dam along the lateral direction. Furthermore, the lateral deformations observed with construction stage analysis and without the construction stages do not have large variations; thus, the construction sequence does not significantly affect the lateral deformations. During the construction, loads due to the self-weight are significant; thus, vertical deformation is highly affected by the construction stage analysis. Since the lateral loads are not significant during the construction, the lateral deformation has less sensitivity to the construction sequence.
In the upstream slope and at the centre, the lateral deformation variation along the dam height is parabolic, where the maximum deformation increases towards the centre and reduces up to the top of the dam. This behaviour represents the water pressure effect on the lateral deformation of the dam. Since the forces generated through the water body increase from the top to the bottom, the increase in the deformation can be seen from the top to the centre along the dam height. Since the material volume increases towards the dam base, the lateral deformations reduce from the centre to the bottom of the dam along the dam height. Due to the toe weight at the downstream slope, the deformation shape has a variation from the parabolic shape observed at the centre and the upstream slope.
To further investigate the lateral deformation along the dam cross section (Chainage 400 m), the deformations at three levels (35 m, 50.5 m and 70.5 m from the dam base) were mapped as shown in .
The same phenomena can be observed at the three levels, where the deformations are significant at the upstream slope and towards the centre and the downstream, the deformations reduce. And towards the centre and the downstream slope of the dam, the deformations at the three levels are similar. At the upstream slope, at 50.5 m level, the deformations are larger than at the level of 70.5 m from the dam base.
4.6. Stress variation in concrete slab
The stress variation is identified using the 2D model analysis implementing a nonlinear model for rockfill material. shows the stress generated in the entire dam structure, and it can observe that the maximum stresses are generated in the concrete slab. In , the stresses generated in the face slab can be observed, where the tensile stresses are present around point D and compressive stresses occur in the E region. The variation of the stresses generated at the three stages is presented in . It is important to note the reduction of the tensile stresses at point D when the toe weights are added thus giving stability to the concrete face. Moreover, through the construction stage analysis, the stress variation at each construction stage can be identified. This would be extremely helpful in the design and construction process to identify and mitigate critical stresses generated.
Table 8. Stresses generated in the concrete slab.
5. Conclusion
Finite element models are developed to accurately depict the behaviour of the actual dam to assess the stability under extreme loading conditions. Therefore, the accuracy of the results depends on the accuracy of the developed finite element model. Initially, the material behaviour should be predicted accurately using material models. In this research, the Duncan and Chang EB model and linear elastic model were used to depict the rockfill material behaviour. A significant variation was observed in the in-situ readings when linear elastic models were used, but with the Duncan and Chang EB model, the results agreed well with the in-situ measurements.
When defining the initial configuration of the finite element model, the construction sequence is crucial. The vertical deformation shape obtained without construction stage analysis was completely different from the actual situation. Moreover, the values showed significant variations to the in-situ measurements when the construction sequence was not considered. With the construction stage analysis, the correct deformation shape was obtained, and the values agreed well with the in-situ readings. When the number of layers is varied, the deviation of results is not significant in most cases; therefore, the optimum number of layers has been utilised in the finite element analysis. Furthermore, no deviation of results was observed when contact surfaces were introduced; thus, it could be concluded that no relative displacement is observed between the rockfill layers and the foundation and between the concrete face and the transition layers.
It can be observed that to obtain accurate lateral deformations the construction stage analysis is not crucial. The material behaviour through the dam can be easily identified by mapping the horizontal deformations where the upstream material had significant movement compared to the centre and the downstream material.
The results obtained through the 2D model show minor variations to the results obtained through the 3D model. Since the analysis conducted was static, it can be concluded that for static analysis a 2D model would be sufficient. This would reduce a significant amount of time when conducting optimisation methods for material parameters.
The deformations and the stresses generated in the foundation and the embankments are not significant. Most importantly, through construction stage analysis, we could identify the progression of stresses during the construction. These data would help reduce structural damage during construction.
Acknowledgments
The research was funded by the Royal Melbourne Institute of Technology Melbourne, Australia, and the University of Peradeniya, Sri Lanka. The necessary data to develop the geometric model of the Kotmale Dam and the monitored data was provided by the Mahaweli Authority, Sri Lanka.
Disclosure statement
The authors declare that they have no known competing financial interests or personal relationships that could have appeared to influence the work reported in this paper.
Data availability statement
The developed finite element models are available from the corresponding author upon request. The geometric data and the monitored data were obtained from the Mahaweli Authority, Sri Lanka. These data can be provided only through consent from the mentioned authority.
References
- Bayraktar, A., M. E. Kartal, and S. Adanur. 2011. “The Effect of Concrete Slab–Rockfill Interface Behavior on the Earthquake Performance of a CFR Dam.” International Journal of Non-Linear Mechanics 46 (1): 35–46. https://doi.org/10.1016/j.ijnonlinmec.2010.07.001.
- Bayraktar, A., M. E. Kartal, and H. B. Basaga. 2009. “Reservoir Water Effects on Earthquake Performance Evaluation of Torul Concrete-Faced Rockfill Dam.” Water Science & Engineering 2 (1): 43–57. https://doi.org/10.3882/j.issn.1674-2370.2009.01.005.
- Dakoulas, P. 2012. “Nonlinear Seismic Response of Tall Concrete-Faced Rockfill Dams in Narrow Canyons.” Soil Dynamics and Earthquake Engineering 34 (1): 11–24. https://doi.org/10.1016/j.soildyn.2011.09.004.
- Fallah, H., and M. Wieland 2013. Koman Concrete Face Rockfill Dam Updating the Static and Seismic Evaluations.
- Ghafari, A., H. R. Nikraz, and A. Sanaeirad. 2015. “Finite Element Analysis of Deformation and Arching Inside the Core of Embankment Dams During Construction.” Australian Journal of Civil Engineering 14 (1): 13–22. https://doi.org/10.1080/14488353.2015.1092639.
- Guo, Q., L. Pei, Z. Zhou, J. Chen, and F. Yao. 2016. “Response Surface and Genetic Method of Deformation Back Analysis for High Core Rockfill Dams.” Computers and Geotechnics 74:132–140. https://doi.org/10.1016/j.compgeo.2016.01.001.
- Jia, Y., and S. Chi. 2015. “Back-Analysis of Soil Parameters of the Malutang II Concrete Face Rockfill Dam Using Parallel Mutation Particle Swarm Optimization.” Computers and Geotechnics 65:87–96. https://doi.org/10.1016/j.compgeo.2014.11.013.
- Kartal, M. E., and A. Bayraktar. 2013. “Non-Linear Earthquake Response of CFR DAM–Reservoir–Foundation Systems.” Mathematical and Computer Modelling of Dynamical Systems 19 (4): 353–374. https://doi.org/10.1080/13873954.2012.759595.
- Kartal, M. E., and A. Bayraktar. 2014. “Non-Linear Response of the Rockfill in a Concrete-Faced Rockfill Dam Under Seismic Excitation.” Mathematical and Computer Modelling of Dynamical Systems 21 (1): 77–101. https://doi.org/10.1080/13873954.2014.898157.
- Kartal, M. E., A. Bayraktar, and H. B. Başağa. 2010. “Seismic Failure Probability of Concrete Slab on CFR Dams with Welded and Friction Contacts by Response Surface Method.” Soil Dynamics and Earthquake Engineering 30 (11): 1383–1399. https://doi.org/10.1016/j.soildyn.2010.06.013.
- Özkuzukiran, S., M. Y. Özkan, M. Özyazicioğlu, and G. S. Yildiz. 2006. “Settlement Behaviour of a Concrete Faced Rock-Fill Dam.” Geotechnical and Geological Engineering 24 (6): 1665–1678. https://doi.org/10.1007/s10706-005-5180-1.
- Seiphoori, A., S. Mohsen Haeri, and M. Karimi. 2011. “Three-Dimensional Nonlinear Seismic Analysis of Concrete Faced Rockfill Dams Subjected to Scattered P, SV, and SH Waves Considering the Dam–Foundation Interaction Effects.” Soil Dynamics and Earthquake Engineering 31 (5–6): 792–804. https://doi.org/10.1016/j.soildyn.2011.01.003.
- Xu, B., D. Zou, and H. Liu. 2012. “Three-Dimensional Simulation of the Construction Process of the Zipingpu Concrete Face Rockfill Dam Based on a Generalized Plasticity Model.” Computers and Geotechnics 43:143–154. https://doi.org/10.1016/j.compgeo.2012.03.002.
- Yu, Y., B. Zhang, and H. Yuan. 2007. “An Intelligent Displacement Back-Analysis Method for Earth-Rockfill Dams.” Computers and Geotechnics 34 (6): 423–434. https://doi.org/10.1016/j.compgeo.2007.03.002.
- Zhang, Y., X. Kong, D. Zou, and B. Xu. 2017. “Tensile Stress Responses of CFRD Face Slabs During Earthquake Excitation and Mitigation Measures.” International Journal of Geomechanics 17 (12). https://doi.org/10.1061/(ASCE)GM.1943-5622.0000997.
- Zhang, B., J. G. Wang, and R. Shi. 2004. “Time-Dependent Deformation in High Concrete-Faced Rockfill Dam and Separation Between Concrete Face Slab and Cushion Layer.” Computers and Geotechnics 31 (7): 559–573. https://doi.org/10.1016/j.compgeo.2004.07.004.
- Zhang, G., and J.-M. Zhang. 2009. “Numerical Modeling of Soil–Structure Interface of a Concrete-Faced Rockfill Dam.” Computers and Geotechnics 36 (5): 762–772. https://doi.org/10.1016/j.compgeo.2009.01.002.
- Zhou, W., J. Hua, X. Chang, and C. Zhou. 2011. “Settlement Analysis of the Shuibuya Concrete-Face Rockfill Dam.” Computers and Geotechnics 38 (2): 269–280. https://doi.org/10.1016/j.compgeo.2010.10.004.
- Zou, D., B. Xu, X. Kong, H. Liu, and Y. Zhou. 2013. “Numerical Simulation of the Seismic Response of the Zipingpu Concrete Face Rockfill Dam During the Wenchuan Earthquake Based on a Generalized Plasticity Model.” Computers and Geotechnics 49:111–122. https://doi.org/10.1016/j.compgeo.2012.10.010.