Abstract
Context
Diabetic kidney disease (DKD) affects nearly 40% of diabetic patients, often leading to end-stage renal disease that requires renal replacement therapies, such as dialysis and transplantation. The gut microbiota, an integral aspect of human evolution, plays a crucial role in this condition. Traditional Chinese medicine (TCM) has shown promising outcomes in ameliorating DKD by addressing the gut microbiota.
Objective
This review elucidates the modifications in gut microbiota observed in DKD and explores the impact of TCM interventions on correcting microbial dysregulation.
Methods
We searched relevant articles from databases including Web of Science, PubMed, ScienceDirect, Wiley, and Springer Nature. The following keywords were used: diabetic kidney disease, diabetic nephropathy, gut microbiota, natural product, TCM, Chinese herbal medicine, and Chinese medicinal herbs. Rigorous criteria were applied to identify high-quality studies on TCM interventions against DKD.
Results
Dysregulation of the gut microbiota, including Lactobacillus, Streptococcus, and Clostridium, has been observed in individuals with DKD. Key indicators of microbial dysregulation include increased uremic solutes and decreased short-chain fatty acids. Various TCM therapies, such as formulas, tablets, granules, capsules, and decoctions, exhibit unique advantages in regulating the disordered microbiota to treat DKD.
Conclusion
This review highlights the importance of targeting the gut-kidney axis to regulate microbial disorders, their metabolites, and associated signaling pathways in DKD. The Qing-Re-Xiao-Zheng formula, the Shenyan Kangfu tablet, the Huangkui capsule, and the Bekhogainsam decoction are potential candidates to address the gut-kidney axis. TCM interventions offer a significant therapeutic approach by targeting microbial dysregulation in patients with DKD.
Introduction
Diabetic kidney disease (DKD) develops in up to 40% of patients with diabetes and is recognized as a significant microvascular complication of diabetes. Globally, DKD is the primary cause of chronic kidney disease (CKD) and end-stage renal disease (ESRD) (Alicic et al. Citation2017). DKD is distinguished by microalbuminuria and macroalbuminuria, with morphological changes that include nodular expansion of the mesangium, diffuse thickening of the glomerular/tubular basement membranes, and glomerular hypertrophy (Anders et al. Citation2018). The pathological features of DKD are characterized by four hierarchies of glomerular lesions, including thickening of the glomerular basement membrane, mild/severe mesangial expansion, nodular sclerosis (Kimmelstiel-Wilson lesions), and glomerulosclerosis (Qi et al. Citation2017). Hyperglycemia, hypertension, and epigenetics contribute to the promotion of DKD, with its natural course involving glomerular hyperfiltration, progressive albuminuria, a decrease in glomerular filtration rate (GFR), and eventual progression to ESRD (Alicic et al. Citation2017).
Diabetic kidneys commonly experience hypoxia, and enhancing the activity of hypoxia-inducible factors may present a novel approach to treating DKD by improving kidney oxygen homeostasis (Persson and Palm Citation2017). Hypoglycemic drugs, such as SGLT2 inhibitors and GLP-1R agonists, along with antihypertensive drugs, contribute to slowing the progression of DKD. However, ineffective treatment or unsatisfactory outcomes are not uncommon (Patel et al. Citation2020). Addressing this clinical challenge requires the development of innovative strategies to prevent DKD. In the era of big data, Fu et al. (Citation2019) proposed that gaining a new understanding of the changes in kidney structure associated with diabetes, coupled with emerging research technologies, will provide avenues for advancing the clinical treatment of DKD. Timely nephrological evaluations, intensified treatment, and dietary strategies guided by multidisciplinary experts may collectively contribute to the delay in the onset and progression of DKD (Gembillo et al. Citation2021).
Organic intercommunication between the gut and kidney has been documented to affect human health directly or indirectly (Giordano et al. Citation2021; Liu et al. Citation2021). With advances in high-throughput sequencing technologies, the gut microbiota (GM), recognized as a crucial factor in modulating enterocyte metabolism and shaping local/systemic immunity, has gained increasing attention (Krautkramer et al. Citation2021). Substantial evidence indicates that pathological alterations in the intestinal microbiome during diseases compromise the integrity and function of the intestinal defense barrier, leading to the translocation of viable bacteria from the gut to the extraintestinal kidney. This results in metabolic disorders and the accumulation of uremic toxins (Lv et al. Citation2022). In contrast, the uremic state of the kidney induces changes in microbial composition, abnormal activation of immune cells, overproduction of antibodies and immune complexes, and infiltration of inflammatory cells, which collectively exacerbates damage to the renal parenchyma (Chen et al. Citation2019). Modulating abnormal GM is promising as a potential approach to prevent or alleviate kidney diseases related to diabetes and obesity (Zaky et al. Citation2021).
Clinical and experimental studies on DKD have provided evidence suggesting that novel treatment strategies can be developed once the pathophysiological mechanisms underlying the gut-kidney axis are elucidated (Lehto and Groop Citation2018; Zhao Citation2022). Evidence from DKD studies in db/db mice has shed light on the gut-plasma metabolism-kidney axis, and a comprehensive understanding of this axis holds promise for advancing our knowledge of DKD pathogenesis (Wu et al. Citation2022). Mosterd et al. (Citation2021) summarized evidence on the gut-kidney axis and inflammation driven by gut dysbiosis in CKD and DKD, focusing primarily on short-chain fatty acids (SCFAs) and end-products of protein metabolism. Lu et al. (Citation2018) reported that intestinal dysbiosis activates the renal renin-angiotensin system (RAS), contributing to the development of DKD. Wang et al. (Citation2021) reviewed how GM dysbiosis promotes DKD progression by altering metabolite profiles and activating the immune response. Fernandes et al. (Citation2019) proposed that diabetic GM dysbiosis, characterized by inflammation and immunosenescence, promotes the progression of nephropathy. Lin et al. (Citation2022) demonstrated that the dysbiotic microbiota played a crucial role in the pathogenesis of DKD by generating uremic toxins, emphasizing that modifying the diet and controlling environmental factors are essential strategies to prevent DKD progression. Given the limited attention to the microbial signature and metabolites in animal and human DKD and intervention methods, exploring the potential interaction between the gut and kidney from this perspective is considered a new hypothesis for understanding and managing DKD.
This review presents insights into GM alterations, associated metabolites, and their connections to DKD. We summarize the latest findings concerning the intriguing features of the bidirectional relationship between the gut and kidney, focusing on microbes, metabolite profiling, and signaling molecules. Finally, we discuss and emphasize intervention strategies, including traditional Chinese medicine (TCM) and diet approaches. This study aims to stimulate further research into the intricate pathways associated with the microbiome and its metabolites, offering a promising avenue for treating and managing DKD.
Materials and methods
Search strategy
Research questions are as follows: (1) What are the characteristics of gut microbiome in animal and human DKD models? (2) Which metabolites are key indicators of microbial dysregulation in individuals with DKD, and how they change? (3) How does TCM regulate the disordered microbiota in DKD? A comprehensive literature search was conducted in English databases (Web of Science, PubMed, ScienceDirect, Wiley, and Springer Nature), from January 2018 to September 2023, using specific keywords, such as ‘gut microbiota’, ‘diabetic kidney disease’, ‘diabetic nephropathy’, ‘natural product’, ‘traditional Chinese medicine’, ‘Chinese herbal medicine’, and ‘Chinese medicinal herb’. Chinese databases were not searched. The literature retrieval strategy used was: (Topic = ‘gut microbiota’) AND (Topic = ‘diabetic kidney disease’ OR ‘diabetic nephropathy’) AND (Topic = ‘natural product’ OR ‘traditional Chinese medicine’ OR ‘Chinese herbal medicine’ OR ‘Chinese medicinal herb’).
Inclusion and exclusion criteria
The studies providing the details of microbiome alterations in DKD and of the TCM regulating microbiota were included. Literature containing incomplete references and issue number were excluded.
Literature screening and data extraction
The irrelevant and duplicate items of the retrieved studies were removed. Two independent investigators (XYH and LZ) first screened studies by titles and abstracts, and further decided the final inclusion by assessing the full texts based on the eligible criteria. The article types of primary research, secondary sources, such as review articles, and randomized controlled trials were included. The following information was extracted: study characteristics, microbial community composition, DKD models, and TCM intervention. Any disagreements were solved by the third investigator (YLZ).
Results
Features of the gut microbiome linked to DKD
The primary clinical features of DKD are characterized by varying degrees of albuminuria and damage to the glomerular filtration barrier. However, this paradigm may not fully capture the complexities observed in normoalbuminuric diabetic patients with progressive renal insufficiency and non-proteinuric patients experiencing renal function loss, known as normoalbuminuric DKD and non-proteinuric DKD, respectively. Increasing evidence suggests that the disrupted microbiota and its metabolites, including trimethylamine-N-oxide (TMAO), indoxyl sulfate (IS), and p-cresol sulfate (p-CS), play crucial roles in DKD (Zhao Citation2022).
An analysis of fecal samples from 15 nondialysis DKD patients and 15 healthy controls revealed a significant correlation between decreased Roseburia intestinalis and increased Bacteroides stercoris with clinical indices (HbA1C, total cholesterol) in DKD (Zhang et al. Citation2022). A meta-analysis involving 15 studies and 1640 participants, including nondialysis DKD patients and healthy controls, showed depletion of Butyricicoccus, Faecalibacterium, and Lachnospira in DKD. In contrast, the genera Hungatella and Escherichia were significantly enriched, with proportions of statistical differences exceeding 0.8 (Han et al. Citation2022). A systematic review and meta-analysis of 578 nondialysis DKD patients and 444 healthy controls in 16 studies showed that DKD patients exhibited expansions of the Escherichia, Citrobacter, and Klebsiella genera, along with a depletion of Roseburia (Wang et al. Citation2022c). These findings indicate that patients with DKD exhibit specific alterations in the gut microbiome at various taxonomic levels, emphasizing the importance of understanding taxonomic distributions in different DKD subjects, including the phylum, class, order, family, and genus levels.
Four dominant phyla, Firmicutes, Bacteroidetes, Actinobacteria, and Proteobacteria, make up 99% of bacteria in the healthy human gut (Feng et al. Citation2019). Firmicutes, as a typical phylum that produces SCFAs, are generally involved in reducing inflammation in the body. Firmicutes mainly include classes Bacilli and Clostridia, orders Lactobacillales and Clostridiales, families Lactobacillaceae and Clostridiaceae, in which genera Lactobacillus, Streptococcus, Clostridium (an opportunistic pathogen), Ruminococcus, Faecalibacterium, Oscillospira, Roseburia, Anaerostipes, Dorea, Blautia, and Lachnospira are primarily involved (Seong et al. Citation2018). Lactobacillales are predominated by Lactobacillus and Streptococcus. Clostridiales account for 95% of Firmicutes. It mainly consists of three common family Clostridium clusters: IV (Ruminococcaceae, genera Ruminococcus, Faecalibacterium, Oscillospira, Anaerotruncus, Subdoligranulum, Butyricicoccus), XI (Peptostreptococcaceae, Clostridium sordellii and Clostridioides difficile, and genus Anaerococcus), and XIVa (Lachnospiraceae, genera Anaerosporobacter, Anaerostipes, Butyrivibrio, Phascolarctobacterium, Roseburia, Coprococcus, Dorea, and Blautia) (Cuív et al. Citation2015). The genus Ruminococcus, which belongs to Ruminococcaceae and Lachnospiraceae, is considered the core bacterium in most human guts, together with Blautia (a genus derived from the reassignment of many former Ruminococcus spp.), plays a vital role in polysaccharide degradation (La Reau and Suen Citation2018). Butyrogenic bacteria (Faecalibacterium, Anaerotruncus, Subdoligranulum, Anaerostipes, Butyrivibrio) within Clostridium clusters IV and XIVa are used as probiotics to increase butyrate levels in the colon (Fu et al. Citation2019). Based on data from experimental murine models of DKD, Li et al. (Citation2020b) presented that the genera Allobaculum and Anaerosporobacter were more abundant in mice with severe proteinuria. Blautia was more abundant in mice with mild proteinuria compared to healthy mice.
The members of Bacteroidetes are known for their ability to degrade a variety of complex carbohydrates. The genera Bacteroides, Prevotella, Paraprevotella, Porphyromonas, Alistipes, Odoribacter, Parabacteroides, and Flavobacterium are usually predominant (Johnson et al. Citation2017). Bacteroides generally maintain a beneficial relationship with the host. However, they escape the intestinal environment, leading to bacteremia and abscesses (Wexler Citation2007). In streptozotocin (STZ)-induced diabetic mice, Bacteroides, Alistipes, and Odoribacter x were associated with the progress and therapeutic effect of type 1 diabetes mellitus (T1DM) (Cao et al. Citation2021). Prevotella is classically considered beneficial for health due to its positive role in fermenting a plant-based diet rich in fibers and improving glucose metabolism (Kovatcheva-Datchary et al. Citation2015). However, some Prevotella strains (Prevotella nigrescens, Prevotella intermedia) are clinically important pathobionts, as they participate in human disease by promoting chronic inflammation (Larsen Citation2017). The microbial analysis results of 60 nondialysis DKD patients in stages III (urinary albumin to creatinine ratio, UACR 30–300 mg/g), IV (UACR > 300 mg/g) and V (estimated GFR, eGFR < 20 mL/min/1.73 m2) showed that the bacterial diversity decreased with DKD progression, and Bacteroides, Alistipes, Lachnoclostridium, Ruminococcus torques, Subdoligranulum may be detrimental factors of the disease progression (Zhang et al. Citation2022).
Relationship of the gut microbiome and its metabolites with DKD
The dysbiosis of the intestinal microbiota is associated with endotoxemia, disruption of the intestinal barrier, abnormal production of metabolites, and inflammatory status (Chi et al. Citation2021). This dysbiosis has direct effects on renal function. In STZ combined with uninephrectomy-induced DKD rats, positive correlations were observed between Bacteroidetes and Elusimicrobia with UACR and tubular injury index. At the same time, Firmicutes and Actinobacteria were negatively correlated with these indicators (Zhao et al. Citation2020). In Shenyan Kangfu tablet-treated db/db mice, Chen et al. (Citation2021) found that high abundances of Bacteroidetes and Proteobacteria were positively correlated with HbA1c, urine microalbuminuria, and UACR levels. At the same time, Firmicutes exhibited negative correlations with these three parameters. In resveratrol-treated db/db mice, reversing the altered gut microbiota and inflammatory responses was associated with improved kidney function (Cai et al. Citation2020). These findings suggest a positive correlation between intestinal microbiota imbalance and DKD.
Microbial dysregulation-associated metabolites have been observed in DKD. Plasma analysis of early-stage DKD patients revealed that producing uremic solutes and oxidative stress markers (glutamine, phenylacetylglutamine, and IS) could indicate declined kidney function (Tan et al. Citation2021). Barrios et al. (Citation2015) found that IS, phenylacetylglutamine, and p-CS were early markers of kidney function decline and inversely associated with eGFR in fecal samples from 855 individuals with early kidney disease. Gut microbiome-derived phenyl sulfate (PS) led to albuminuria in DKD rats by inducing inflammation and perivascular fibrosis and promoting glomerulosclerosis (Kikuchi et al. Citation2019). In T2DM-CKD patients, elevated serum LPS had significantly positive correlations with inflammatory marker levels (TNF-α, IL-6) (Salguero et al. Citation2019). In individuals with DKD, serum and fecal SCFA levels were lower than healthy individuals and negatively correlated with renal function (Zhong et al. Citation2021). Clinical data from 100 DKD patients showed that acetate, butyrate, and isovalerate were negatively associated with DKD. However, high serum concentrations of propionate and isobutyrate synergistically increased the risk of DKD in T2DM patients (Li et al. Citation2022). These findings suggest that increased uremic solutes and decreased SCFAs are essential indicators of microbial aberrations in DKD.
Together, GM aberrations promote DKD by altering its composition and function, accumulating uremic toxins, decreasing the content of beneficial SCFAs, and mediating low-grade inflammation, nephrotoxicity, and glomerulosclerosis/renal tubulointerstitial fibrosis in the kidney. Pathogen recognition receptors (PRRs), as germline-encoded host sensors, receive pathogen-associated molecular patterns in an evolutionarily conserved way and trigger various antimicrobial immune responses (Kumar et al. Citation2011). Disordered GM promotes DKD through metabolic disarrangement and activation of PRRs. Detailed evidence is shown in .
Figure 1. The gut microbiota aberrations promote diabetic kidney disease through metabolic disarrangement involving pathogen-associated molecular patterns (PAMPs) and pathogen recognition receptors (PRRs). The decreased microbial richness or diversity causes alterations in microbial composition and function, translocating opportunistic pathogens (pathobionts) like LPS, and activating toll-like receptors (TLRs), NOD-like receptors (NODs), reducing SCFAs, and increasing uremic toxins, leading to low-grade inflammation, nephrotoxicity, progressive glomerulosclerosis and fibrosis, consequently a reduced glomerular filtration rate (GRF) and proteinuria.
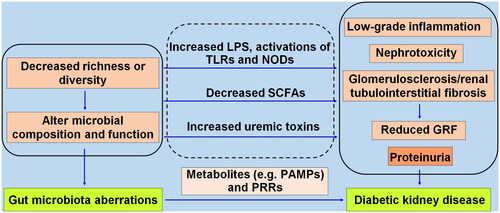
Gut microbiome-associated metabolites in DKD
GM plays a crucial role in metabolizing complex carbohydrates, proteins, and peptides from food that cannot be digested and absorbed by the small intestine, resulting in the production of a diverse array of end-products, such as SCFAs, amines, indoles, ammonia, thiols, and phenols. The daily diet significantly influences the balance of microorganisms and the production of these metabolites. In both human and animal models of kidney diseases, the accumulation of metabolic endotoxins indicates an increase in proteolytic bacteria (Enterobacteria and Enterococci) and a reduction in bacteria (Lactobacillaceae and Prevotellaceae) that possess SCFA-forming enzymes (Lau et al. Citation2021). Researchers have suggested that SCFAs, bile acids (BAs), and uremic toxins (IS, p-CS, TMAO) play distinct roles in DKD. IS and p-CS are entirely microbial products, while TMAO is derived primarily from the microbiota and dietary components (Mishima et al. Citation2017). This discussion highlights the characteristics of the main metabolites, aiming to uncover related signaling pathways and gain insight into the intricate communication between the gut and the kidney in DKD.
SCFAs
SCFAs, organic fatty acids comprising 1–6 carbon atoms, represent the primary products of bacterial fermentation within the colon. The main SCFAs consist of acetic acid, propionic acid, and butyric acid, with concentration proportions in the colonic lumen of ∼60, 25, and 15%, respectively. Acetate can reach peripheral tissues and undergo metabolic processes in the circulation, while the liver predominantly takes up propionate. Butyrate, a significant energy source for colonocytes and enterocytes, plays a crucial role in regulating the generation, trafficking, and function of adaptive and innate immune cells (Gonçalves et al. Citation2018). In individuals with advanced DKD, significantly lower levels of valerate and caproate have been shown to predict renal outcomes in patients with biopsy-confirmed DKD (Zhong et al. Citation2022).
The production of SCFAs constitutes one of the primary mechanisms through which GM modulates the host’s energy metabolism. SCFA content, synthesis, and bioavailability depend highly on dietary components, enzymes, and microbial activity (Koh et al. Citation2016). Genera, such as Ruminococcus, Faecalibacterium, Roseburia, Clostridium, Coprococcus, Blautia, and Eubacterium from the Firmicutes phylum, as well as Bacteroides, Alistipes, and Prevotella within the Bacteroidetes phylum, efficiently release SCFAs by fermenting non-digestible carbohydrates in the upper gastrointestinal tract. Firmicutes primarily produce butyric acids, whereas Bacteroidetes mainly secrete high levels of acetic acid and propionic acid (Fu et al. Citation2019).
Compelling evidence indicates that SCFAs can directly activate G protein-coupled receptors (GPR41, GPR43, Olfr78, GPR109A), inhibit histone deacetylase, and serve as energy substrates for intestinal cells, positively regulating host physiology. GPR41 and GPR43, highly expressed in adipose tissue and immune cells, respectively, are activated primarily by propionic acid and butyric acid. The affinity values (EC50) for propionic acid and butyric acid on GPR41 and GPR43 in humans are 6–127 and 28–371 µM, respectively. Olfr78 is mainly expressed in the renal juxtaglomerular apparatus and is an oxygen sensor for renin secretion and blood pressure regulation. Acetic acid and propionic acid are the primary activators of Olfr78, with EC50 values of 2.35 mM and 920 µM, respectively. GPR109A, predominantly expressed in the lumen-facing apical membrane of colonic and intestinal epithelial cells, generally recognizes butyric acid as a ligand, with an EC50 value of ∼0.7 mM.
A substantial body of recent research, including experimental animal studies and patient investigations, has shed light on the therapeutic potential of supplemental SCFAs in DKD (Li et al. Citation2019). Moon et al. (Citation2021) proposed that circulating SCFAs contribute to metabolic processes that mitigate the development of albuminuria in T1DM. Generally, alternative therapy with SCFAs enhances kidney function by activating GPR43 and GPR109A. GPR43 activation promotes the MAPK cascade and inhibits NF-κB activation by coupling with Gi/o and Gq/11 proteins, as well as β-arrestin2, thereby augmenting the immune response in cells. GPR109A plays a role in limiting inflammation by enhancing the anti-inflammatory properties of macrophages and dendritic cells and inducing the differentiation of regulatory T cells (Lymperopoulos et al. Citation2022).
In the presence of GPR43 or GPR109A, treatment with acetate, butyrate, or propionate protected diabetic mice from nephropathy and inhibited high glucose-induced inflammation in renal tubular cells and podocytes. This protective effect was associated with the suppressed expression of inflammatory cytokines (IL-6, IFN-γ), chemokines (CCL2, CXCL10), and fibrosis-related genes (fibronectin, TGF-β) (Li et al. Citation2020a). Sodium butyrate (or butyrate) alleviates cellular apoptosis, injury, and inflammation in high glucose-induced cells and db/db mice (Du et al. Citation2020; Cheng et al. Citation2022). Exogenous butyrate improved kidney damage in DKD mice and alleviated high glucose-induced injury in glomerular mesangial cells (GMCs) through GPR43-mediated inhibition of oxidative stress and NF-κB signaling (Huang et al. Citation2020). Supplementary butyrate suppressed the expression of TGF-β and P311 in the kidneys of db/db mice and high glucose-induced SV40-MES-13 cells (Du et al. Citation2020). In db/db mice, butyrate supplementation in a normal diet protected DKD-induced muscle atrophy by enhancing gut barrier function and activating the GPR41-mediated PI3K/Akt/mTOR pathway (Tang et al. Citation2022). Cai et al. (Citation2022) found that oral butyrate supplementation improved renal injury in diabetic rats by increasing autophagy-associated activation of the AMPK/mTOR pathway.
Contradictory results regarding the acetate supplementation or the activation of SCFA receptors have emerged. In a DKD model induced by intraperitoneal injection of STZ, acetate exhibited a positive correlation with the expression of the intrarenal angiotensin II protein, and the excessive presence of acetate in plasma-activated intrarenal RAS, which is a known initiator of DKD (Lu et al. Citation2020). Furthermore, in fecal samples of diabetic rats, excessive acetate disrupted cholesterol homeostasis by activating GPR43, leading to tubulointerstitial injury in DKD (Hu et al. Citation2020). A previous study also reported that acetate promoted podocyte injury by stimulating GPR43 activation and inhibiting Akt signaling (Lu et al. Citation2021). In contrast, in GPR109A-/- diabetic mice, GPR109A deletion did not play a critical role in maintaining intestinal homeostasis (Snelson et al. Citation2020). In summary, these data suggest that the potential beneficial effects of supplementing SCFAs or activating their receptors on DKD remain subject to further investigation.
Bile acids
BAs play a crucial role as intermediates in the physiological conversion of lipids, including sterols and phospholipids. Under normal physiological conditions, anaerobic bacteria, such as Clostridium, Bacteroides, Enterobacter, Escherichia, and Bifidobacteria metabolize primary BAs, such as cholic acid and chenodeoxycholic acid, into secondary BAs, including deoxycholic acid, taurodeoxycholic acid, and taurolithocholic acid, through processes, such as deconjugation, dihydroxylation, and epimerization. In a study in which telmisartan vesicles containing bile salts were administered to STZ-induced DKD rats for 4 weeks, improvements were observed in biological indices (creatinine, urea, total urinary protein) and inflammatory parameters (TNF-α, IL-6) (Ahad et al. Citation2018).
Identifying specific agonists for BA receptors is crucial for understanding the biological mechanisms of BAs in the kidney. Activation of BA receptors, including the nuclear hormone receptor farnesoid X receptor (FXR) and the membrane-bound G protein-coupled BA receptor 1 (GPBAR1, also known as TGR5), holds promise as a therapeutic approach to prevent DKD (Wang et al. Citation2018). Naturally occurring primary BAs, such as chenodeoxycholic acid and tauroursodeoxycholic acid (THDCA), followed by secondary BAs, such as cholic acid, deoxycholic acid, and lithocholic acid, are believed to be effective ligands for FXR. Deoxycholic acid and lithocholic acid activate primarily TGR5. THDCA can activate highly expressed FXR in tubular cells and induce the expression of FXR-dependent genes (SOCS3 and DDAH1). THDCA-treated db/db mice exhibited reduced glomerular and tubular injuries (Marquardt et al. Citation2017).
The FXR agonist GW4064 demonstrated beneficial effects on systemic organs (kidney, pancreas, liver, heart) in db/db mice and improved renal lipid metabolism (Han et al. Citation2021). Treatment of db/db mice with a selective TGR5 agonist resulted in decreased proteinuria, podocyte injury, mesangial expansion, and CD68 macrophage infiltration into the kidney (Wang et al. Citation2016). Gentiopicroside, the main active secoiridoid glycoside, could prevent renal inflammation in diabetic mice by regulating the TGR5-β-arrestin2-NF-κB signaling pathway (Xiao et al. Citation2020). These findings suggest that activating FXR/TGR5 may represent an effective therapeutic option to improve renal injury in diabetic animals. However, the causal relationship between receptor activation and efficacy against DKD needs further elucidation.
Amino acid-derived catabolites
Amino acids, the fundamental building blocks of proteins, also function as signaling molecules in regulating metabolism. Modifying the free amino acid profile in plasma, considered a prognostic factor, can potentially mitigate or prevent the occurrence of end-stage DKD (Saleem et al. Citation2019). In diabetes patients at various stages of CKD, a low level of tryptophan (<44.20 μmol/L) has been associated with a rapid decline in eGFR (Chou et al. Citation2018). Plasma analysis involving 3089 T2DM patients revealed associations between impaired kidney function, branched-chain and aromatic amino acids, and glycoprotein acetyls (Tofte et al. Citation2020). Recent experimental studies suggest that the presence of disordered GM-derived tryptophan metabolites serves as an indicator of renal injury (Liu et al. Citation2021). Modifications in amino acids or their metabolism may play a crucial role in predicting the pathophysiology of DKD.
GM plays a crucial role in the initial steps of amino acid catabolism, primarily through catalytic deamination or decarboxylation reactions that yield carboxylic acids and amines. Commonly catabolized amino acids include sulfur-containing amino acids (methionine and cysteine), basic amino acids (arginine, histidine, and lysine), and aromatic amino acids (tryptophan, tyrosine, and phenylalanine). Generally, methionine and cysteine undergo catabolism into hydrogen sulfide (H2S) and methyl mercaptan through degrading enzymes (methionine γ-lyase, cysteine desulfhydrase) present in sulfidogenic bacteria, such as Streptococcus, Clostridium, Pseudomonas putida, Enterobacter, and Klebsiella (Walker and Schmitt-Kopplin Citation2021). The expansion of sulfidogenic bacteria, such as Clostridium, Pseudomonas putida, Enterobacter, and Klebsiella may contribute to DKD by producing excess sulfides (Xu et al. Citation2017; Gradisteanu et al. Citation2019; Rysz et al. Citation2021). The genera Clostridium, Lactobacillus, Streptococcus, Bifidobacterium, and Escherichia convert basic amino acids into biogenic amines (Pugin et al. Citation2017). Tryptophan, tyrosine, and phenylalanine are primarily metabolized by intestinal anaerobes (genera Clostridium, Bacteroides, Bifidobacterium, Lactobacillus, Escherichia) into indolic and phenolic compounds (Debnath et al. Citation2021). Tryptophan, being the only amino acid with an indole structure, generates metabolites (indole, indolic acid, skatole, and tryptamine) mainly through indole pyruvate decarboxylase and tryptophanase, enzymes present in the genera Lactobacillus, Clostridium, and Bacteroides, as well as Clostridium sporogenes and Ruminococcus gnavus species (Gao et al. Citation2018). The Enterococcus and Clostridium genera typically degrade tyrosine into p-cresol, tyramine, phenols, and p-coumarate. Limited studies have explored phenylalanine-derived metabolites (phenylethylamine, transcinnamic acid) produced by Clostridium and Peptostreptococcus (Oliphant and Allen-Vercoe Citation2019). These findings suggest that the Clostridium genus may be crucial in affecting amino acid catabolism.
Indole is generally sulfonated by sulfotransferase 1A1 into IS, while p-cresol undergoes sulfation to form aromatic p-CS through aryl sulfotransferases (Gryp et al. Citation2017). Enzymes responsible for indole and p-cresol formation have been identified in the Clostridiaceae, Enterobacteriaceae, and Verrucomicrobiaceae families (Rysz et al. Citation2021). Elevated levels of IS and p-CS in patients with T2DM progressing to ESRD were associated with enriched microbes, specifically Clostridiaceae, Enterobacteriaceae, and Verrucomicrobiaceae (Fiaccadori et al. Citation2020). In DKD mice exhibiting renal tubular injury, a significant increase in plasma IS levels was observed (Ahmed et al. Citation2022). Meprin-β was found to improve diabetic kidney injury, promoting the production of uremic toxins (IS, N-methyl-pyridone-carboxamide) and altering the metabolite balance in the kidney (Gooding et al. Citation2019). A preliminary study indicated that the accumulation of IS was associated with increased kidney damage (Ellis et al. Citation2016; Leong and Sirich Citation2016). The use of AST-120 (Kremezin®) suppressed the production of indole and p-cresol, thus delaying the progression of renal dysfunction in DKD (Hwang et al. Citation2019; Sato et al. Citation2020).
The formation of p-cresol sulfate involves mainly the synthesis of phenol by GM, specifically by bacteria belonging to the Clostridiaceae, Bacteroidaceae, and Bifidobacteriaceae families, followed by further transformation in the liver (Thomas et al. Citation2021). Kikuchi et al. (Citation2019) proposed that PS could be a predictive marker of the 2-year progression of albuminuria in patients with microalbuminuria. Comprehensive analyses of various experimental models (transgenic rats, diabetic mice) and clinical studies involving diabetes patients indicate that reducing circulating PS levels may mitigate the risk of developing DKD (Fiaccadori et al. Citation2020). Overexpression of the proximal tubular cell transporter SLCO4C is associated with increased PS excretion, and drugs that upregulate SLCO4C may have therapeutic potential for patients with CKD (Toyohara et al. Citation2009). In the db/db DKD model, inhibitors of the enzyme tyrosine phenol-lyase reduced plasma PS levels and prevented the progression of renal failure (Kikuchi et al. Citation2019). In conclusion, an in-depth investigation into identifying amino acids and their catabolites is imperative for diagnosing, preventing, and treating DKD.
Trimethylamine N-oxide
TMAO is an oxygenated product derived from trimethylamine (TMA), primarily through the action of hepatic flavin-containing monooxygenases, particularly FMO3. Certain members of GM, including the genera Sporosarcina, Citrobacter, Providencia, Escherichia-Shigella, and Klebsiella pneumoniae species, play a key role in this process, which depends on dietary nutrients, such as tertiary amines (choline, betaine, l-carnitine) (Janeiro et al. Citation2018). Human and animal studies suggest that the families Deferribacteraceae (phylum Aquificae), Anaeroplasmataceae (phylum Mollicutes), Prevotellaceae (phylum Bacteroidetes), and Enterobacteriaceae are involved in TMA/TMAO production (Velasquez et al. Citation2016). In a study by Li et al. (Citation2020b) involving male SPF C57BL/6 mice that received fecal microbiota transplantation (FMT) from DKD mice with severe proteinuria, increased abundance of the genus Anaerosporobacter could be related to TMAO production. Individuals with T1DM who had higher plasma TMAO concentrations showed associations with increased mortality, cardiovascular disease events, and unfavorable renal outcomes (Winther et al. Citation2019).
The plasma level of TMAO is associated with CKD in patients with T2DM (Kalagi et al. Citation2023). In individuals with T2DM and albuminuria, four metabolites of the TMAO pathway serve as risk markers for the deterioration of renal function (Winther et al. Citation2021). TMAO promotes DKD by facilitating inflammation, oxidative stress, and fibrosis (Yang et al. Citation2021). Rats with diet-induced DKD (high-fat diet/low-dose streptozotocin) exhibited higher TMAO levels than normal rats, and TMAO treatment exacerbated kidney dysfunction and renal fibrosis by activating NLRP3 (Fang et al. Citation2021).
In T2DM-CKD patients, elevated serum TMAO levels showed positive correlations with markers of intestinal permeability (zonulin), endotoxin lipopolysaccharide (LPS), and serum biomarkers of inflammatory and endothelial dysfunction (IL-6, TNF-α, ET-1) (Al-Obaide et al. Citation2017). Potential approaches to managing TMAO include reducing TMA production by controlling intestinal bacteria or inhibiting the hepatic metabolism of TMA into TMAO. A study demonstrated that lowering microbial TMA production with metformin decreased TMAO availability in db/db mice (Kuka et al. Citation2020). Another study indicated that targeting a TMA-generating enzyme inhibitor ameliorated renal dysfunction in a mouse model of diet-induced obesity by inhibiting TMA production (Sun et al. Citation2017). Despite extensive research on the harmful effects of TMAO and its management in DKD, potential mechanisms still warrant further investigation.
The gut-kidney axis as a therapeutic target for DKD
Considering the abnormal communication between the gut and the kidney, disturbances of GM and its metabolites contribute to the exacerbation of kidney dysfunction, mainly manifested by damage to the glomerular filtration membrane (). Mitigating deleterious changes in GM composition and metabolite profiles and restoration of impaired glomeruli could be beneficial in improving DKD (Wang et al. Citation2021; Ni et al. Citation2022). Potential strategies, including pharmacological therapy and dietary intervention, are summarized in .
Figure 2. Interpreting the mechanism underlying diabetic kidney disease (DKD) from the interplay between gut and kidney, and the therapeutic target of DKD. GM dysbiosis and the metabolite abnormalities responsible for the leaky gut are important features of intestinal epithelial barrier disruption. Kidney dysfunction characterized by glomerular filtration membrane damage includes podocyte injury, mesangial cell proliferation, and endothelial cell damage. The common metabolites derived from microbiota include SCFAs, trimethylamine (TMA), indole, p-cresol, LPS, ammonia, and secondary bile acids (SBAs). TMA, indole, p-cresol, and LPS can directly induce gut leakage. In circulation, these compounds are converted into trimethylamine N-oxide (TMAO), indoxyl sulfate (is), and phenyl sulfate (PS) by flavin-containing monooxygenase 3 (FMO3), sulfotransferase 1A1 (SULT1A1) and tyrosine phenol-lyase (TPL). When they reach the kidney, is and PS upregulates plasminogen activator inhibitor-1 (PAI-1) expression and increases ROS levels by activating NF-κB and NOX4 signaling. LPS mainly activates NF-κB and TLR4 signaling.
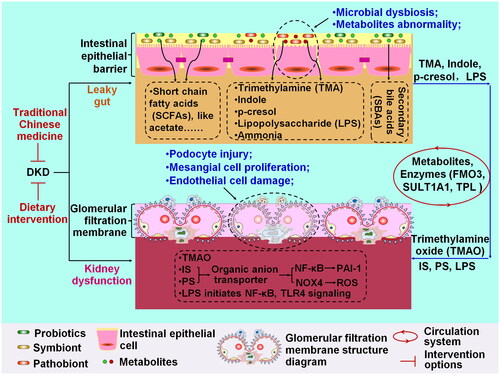
Table 1. Summary of intervention approaches targeting interplay of gut and kidney in diabetic kidney disease (DKD).
Pharmacological therapy
Glucose-controlling agents and RAS inhibitors are commonly used in clinical settings to improve kidney function and reduce albuminuria in DKD despite sometimes causing undesirable outcomes. In particular, the SGLT2 inhibitor empagliflozin has been reported to alleviate T2DM-related DKD by reducing LPS-producing bacteria and increasing SCFA-producing bacteria in DKD mice (Deng et al. Citation2022). Furthermore, emerging studies explore TCM as a potential pharmacological approach for managing DKD (Luo et al. Citation2021; Cao et al. Citation2022; Miao et al. Citation2022; Yu et al. Citation2022; Wang et al. Citation2022a, Citation2022b).
For example, magnesium lithospermate B (MLB), a major component of Danshen water extracts, demonstrated significant decreases in 24 h urinary albumin, bile acids, and the ratio of cholic acid to taurocholic acid when used to treat DKD rats (Zhao et al. Citation2019). Given that MLB has an exceptionally low bioavailability (0.02%) but a significant oral activity (Lee et al. Citation2003), the therapeutic impact of MLB on renal injury may be associated with its modulation of the gut microbiome and bile acid metabolic profiles. The Tangshen formula, a traditional Chinese herbal medicine, significantly attenuated renal injury, reconstructed microbial profiles, and decreased metabolic endotoxemia/LPS in DKD rats (Zhao et al. Citation2020). Similarly, based on the ‘ZhengJia’ theory, the Qing-Re-Xiao-Zheng formula positively affected urinary albumin, colonic mucosa damage, gut dysbiosis, and gut-derived LPS in DKD model mice (Gao et al. Citation2021).
The Shenyan Kangfu tablet, prescribed to treat CKD, demonstrated alterations in gut microbiota composition by increasing Firmicutes and decreasing Bacteroidetes. Furthermore, it exerted anti-inflammatory activity by downregulating the expression of renal inflammatory markers (NF-κB, TNF-α, and IL-1β) (Chen et al. Citation2021). The QiDiTangShen granule, formulated based on the theory of ‘filling the renal essence to treat kidney diseases’, reported alterations in gut microbiota composition, with a reduced Lactobacillus, Bacteroides, and Lachnospiraceae_NK4A136_group and an increase in Alloprevotella. Additionally, it decreased serum bile acid profiles, and the gut-microbiota-bile acid (GM-BA) axis was presumed to be a crucial target for renoprotection of the QiDiTangShen granule (Wei et al. Citation2021). The San-Huang-Yi-Shen capsule, used in DKD treatment, ameliorated detrimental effects in DKD rats by improving hyperglycemia, gut microbiota imbalance, renal dysfunction, oxidative stress, and inflammatory response (Su et al. Citation2021). Similarly, the Huangkui capsule, an Abelmoschus manihot (L.) extract, exhibited modulatory effects on the gut microbiota and improved plasma metabolite levels in non-obese diabetic mice with DKD (Shi et al. Citation2022). These improvements in gut microbiota imbalance and metabolic status, along with the inhibition of inflammatory reactions, are possibly associated with the efficacy of these prescriptions.
Jowiseungki decoction, commonly prescribed for diabetic complications, induced significant changes in the abundance of bacteria, including the Alphaproteobacteria class, the Atopobiaceae family, the genera Acetatifactor, Butyricicoccus, Kerstersia, Peptococcus, and Coriobacteriaceae_UCG-002, when administered orally to STZ-induced DKD mice (Meng et al. Citation2020b). The Bekhogainsam decoction, created by Chang ChungChing in the Treatise on Febrile Disease, demonstrated the ability to suppress inflammatory reactions and reshape gut homeostasis in DKD mice. It achieved this by enhancing the growth of probiotic bacteria (Clostridiales, Peptococcus) and reducing the abundance of Actinobacteria (Meng et al. Citation2020a). Zicuiyin decoction, originating from the Qing dynasty and created by Xichun Zhang, showed the ability to improve kidney function and modulate gut microbiota dysbiosis, particularly in patients with decreased eGFR (Liu et al. Citation2022). When subjected to solid-state fermentation, the medicinal and edible fungus Paecilomyces cicadae significantly reduced urine protein in DKD mice and improved their physiological condition. This effect was attributed to regulating abundances of Ruminococcaceae_UCG-014, Allobaculum, Unclassified_f_Lachnospiraceae Alloprevotella, and Bacteroides (Zhou et al. Citation2022).
Furthermore, a C5a/C5a receptor antagonist alleviated renal macrophage infiltration and proinflammatory factor expression while increasing gut microbiota diversity and SCFA levels in db/db mice (Phull et al. Citation2021). Long-acting pirfenidone reversed gut microbiota dysbiosis in leptin receptor-deficient db/db mice, showing an increase in Lactobacillus and Bacteroides and a decrease in Akkermansia. These findings provide insight into the potential beneficial effects of long-acting pirfenidone therapy (Singh et al. Citation2020). These observations suggest that a comprehensive understanding of the efficacy and mechanisms of traditional medicines will serve as the foundation for basic research on preventing and treating DKD.
Dietary intervention
Dietary intervention by supplementing microecological preparations, polysaccharides, and polyphenolic compounds is beneficial for DKD. Introducing sufficient amounts of biotics into dietary supplements is critical to rescuing microbial populations, improving renal health in diabetes, and potentially restoring a proper intestinal environment (Paul et al. Citation2022). Nagase et al. (Citation2022) proposed that prebiotics and/or probiotics could be individually tailored to prevent and/or treat DKD. Dietary probiotics function by selectively stimulating the growth and/or activity of specific microbial genera/species, with common supplements including Bifidobacterium (such as Bifidobacterium bifidum, Bifidobacterium longum NCC 2705), Lactobacillus, Saccharomyces, and Streptococcus. Numerous studies have shown that probiotic consumption reduces blood urea and p-cresol concentrations and systemic inflammation and increases Bifidobacteria and Lactobacillus counts (Lopes et al. Citation2018).
In clinical practice, probiotics improve glycemia, renal function, and lipid profiles and reduce serum insulin levels (Moravejolahkami et al. Citation2021). Probiotic-rich foods, such as honey, milk, and yogurt, have been reported to decrease inflammatory and oxidative stress biomarker levels in the serum and plasma of patients with these conditions (Navid et al. Citation2019; Jb et al. Citation2021). In a randomized clinical study, consuming Bifidobacterium bifidum, Bifidobacterium bifidum strain ZT-B1, Lactobacillus acidophilus, and Lactobacillus acidophilus ZT-L1 improved glycemic control in patients with DKD (Mafi et al. Citation2018; Jiang et al. Citation2021). Furthermore, supplementing soy milk with the Lactobacillus plantarum A7 probiotic positively affected albuminuria, eGFR, serum creatinine, IL-18, and sialic acid levels in T2DM patients with nephropathy (Miraghajani et al. Citation2017). Similar benefits were observed in DKD patients who consumed probiotic-containing soy milk (Miraghajani et al. Citation2019). A meta-analysis highlighted that the beneficial effects of probiotics depended on intervention duration, probiotic dose, and consumption patterns in patients with DKD (Dai et al. Citation2022). These findings suggest that probiotic supplements benefit DKD patients by reducing uremic toxins, modifying the gut microbiota, and decreasing inflammatory marker levels. However, these favorable effects exhibit heterogeneity, and their reputation is modest. There is still no consensus on the optimal strain or dose of probiotics for individuals with DKD. Future controlled trials with larger sample sizes and longer follow-up periods are necessary to validate the effectiveness of probiotic supplementation (Vlachou et al. Citation2020).
Discussion
Both animal models and humans with DKD indicate that the microbial composition has alterations characterized by a low abundance of Firmicutes and high proportions of Bacteroidetes and Proteobacteria. The proteobacteria-dominated microbiome (family Enterobacteriaceae) is characteristically associated with elevated inflammation. A meta-analysis from hundreds of DKD nondialysis patients and data from the experimental murine models of DKD showed that the beneficial microbes are commonly depleted, specifically Clostridiales order, including families Ruminococcaceae and Lachnospiraceae, genera Roseburia, Faecalibacterium. Blautia, Butyricicoccus, Coprococcus, species Roseburia spp., Faecalibacterium prausnitzii, etc. The genera Odoribacter, Parabacteroides, Alloprevotella, and Akkermansia potentially protect DKD. Some pathogenic bacteria (Escherichia, Allobaculum, Hungatella, Haemophilus, Desulfovibrio) and opportunistic pathogens (Clostridium, Enterococcus, Bacteroides, Klebsiella, Parasutterella, Citrobacter, Veillonella) as detrimental factors promote DKD. Due to the limited reported research, the roles of Alistipes, Rikenella, Lachnoclostridium, and Subdoligranulum in DKD must be further confirmed. Overall, GM alterations were found in experimental models of DKD, but more studies should be undertaken to characterize general modifications of the gut microbiome in various DKD models.
Data on the gut microbiome-associated metabolites of DKD patients and animal models demonstrate that the increased uremic solutes and decreased SCFAs are key indicators of microbial dysregulation. Their levels are significantly correlated with inflammatory marker levels (TNF-α, IL-6) and kidney function indicators (e.g., eGFR). These suggest that a comprehensive understanding of the metabolites associated with GM may mitigate kidney damage, and provide valuable information on the roles, spatial concentrations, and potential functions of microbial metabolites. Simultaneously, it also could be a cornerstone for improving systemic inflammatory responses and addressing multiple organ dysfunctions in DKD.
TCM emerges as an ideal regulator of intestinal microecology in rats and mice models of DKD, demonstrating beneficial effects on DKD. Formulations, such as Tangshen formula, Qing-Re-Xiao-Zheng formula, Shenyan Kangfu tablet, and Jowiseungki decoction have a good effect in increasing Firmicutes and decreasing abundances of Bacteroidetes and Proteobacteria. Specifically, probiotic Clostridiales order, families Ruminococcaceae and Lachnospiraceae, genera Alloprevotella and Butyricicoccus increase, whereas genera Bacteroides and Allobaculum decrease. The alterations of these microbial compositions protect intestinal barrier function, reduce metabolic endotoxins (e.g., LPS) and some inflammatory markers (e.g., NF-κB, TNF-α, and IL-1β) levels, and balance SCFA and BA metabolism. Despite extensive cellular and animal experiments, clinical trials of TCM for DKD treatment are limited. More research is imperative to comprehensively understand their mechanisms of action, ensure safety, and establish effectiveness, thus promoting TCM as a priority strategy for DKD treatment.
This study has several limitations. First, our data may have omitted publications from other databases, such as International Pharmaceutical Abstracts. Furthermore, our study was limited to publications in the English language. Despite these limitations, the findings of our efforts can offer valuable insight and guidance for future research on the prevention of DKD.
Conclusions
Profound changes in GM structure, the accumulation of uremic toxins, systemic inflammation, and malnutrition are potential contributors to the abnormal interaction between the gut and the kidney, leading to the onset and progression of DKD. Significant associations between the GM and DKD phenotype, along with microbial markers that classify DKD, offer promising avenues for research. Joint efforts in basic and clinical research to identify the richness/diversity, composition, and metabolic features of DKD could provide opportunities to understand the pathogenesis of the disease. Both pharmacological therapy and dietary intervention have shown beneficial effects in improving DKD. Traditional Chinese herbal medicines, including the Tangshen formula, Shenyan Kangfu tablet, QiDiTangShen granule, and Jowiseungki decoction, have shown protective effects on DKD by targeting the gut-kidney axis related to the microbial disorder, its metabolites, and signaling pathways in DKD. Innovative research and preclinical and clinical trials will provide information on the TCM intervention. Additionally, large-sample clinical investigations covering different stages of DKD that integrate multiomics (such as transcriptomics, proteomics, and metabolomics) and molecular biological methods are essential to develop effective approaches to managing DKD. Deciphering the mechanisms underlying DKD through the interrelationship between the gut and kidney and targeting gut microbiota using TCM represents a promising therapeutic strategy for clinically treating DKD.
Authors contributions
XQW and YYZ designed and wrote the review. XQW, LZ, and YLZ collected and analyzed the data. YLZ and XL revised the manuscript. XYH and LZ downloaded references and processed the figures and table in the manuscript. YYZ and XL supervised the draft preparation. All authors accepted the final version of the review.
Disclosure statement
No potential conflict of interest was reported by the author(s).
Additional information
Funding
References
- Ahad A, Raish M, Ahmad A, Al-Jenoobi F, Al-Mohizea A. 2018. Development and biological evaluation of vesicles containing bile salt of telmisartan for the treatment of diabetic nephropathy. Artif Cells Nanomed Biotechnol. 46(sup1):532–539. doi: 10.1080/21691401.2018.1430700.
- Ahmed S, Sparidans R, Lu J, Mihaila S, Gerritsen K, Masereeuw R. 2022. A robust, accurate, sensitive LC-MS/MS method to measure indoxyl sulfate, validated for plasma and kidney cells. Biomed Chromatogr. 36(5):e5307.
- Alicic RZ, Rooney MT, Tuttle KR. 2017. Diabetic kidney disease: challenges, progress, and possibilities. Clin J Am Soc Nephrol. 12(12):2032–2045. doi: 10.2215/CJN.11491116.
- Al-Obaide M, Singh R, Datta P, Rewers-Felkins K, Salguero M, Al-Obaidi I, Kottapalli K, Vasylyeva T. 2017. Gut microbiota-dependent trimethylamine-N-oxide and serum biomarkers in patients with T2DM and advanced CKD. J Clin Med. 6(9):86. doi: 10.3390/jcm6090086.
- Anders H, Huber T, Isermann B, Schiffer M. 2018. CKD in diabetes: diabetic kidney disease versus nondiabetic kidney disease. Nat Rev Nephrol. 14(6):361–377. doi: 10.1038/s41581-018-0001-y.
- Barrios C, Beaumont M, Pallister T, Villar J, Goodrich J, Clark A, Pascual J, Ley R, Spector T, Bell J, et al. 2015. Gut-microbiota-metabolite axis in early renal function decline. PLOS One. 10(8):e0134311. doi: 10.1371/journal.pone.0134311.
- Cai K, Ma Y, Cai F, Huang X, Xiao L, Zhong C, Ren P, Luo Q, Chen J, Han F. 2022. Changes of gut microbiota in diabetic nephropathy and its effect on the progression of kidney injury. Endocrine. 76(2):294–303. doi: 10.1007/s12020-022-03002-1.
- Cai T, Ye X, Li R, Chen H, Wang Y, Yong H, Pan M, Lu W, Tang Y, Miao H, et al. 2020. Resveratrol modulates the gut microbiota and inflammation to protect against diabetic nephropathy in mice. Front Pharmacol. 11:1249. doi: 10.3389/fphar.2020.01249.
- Cao G, Miao H, Wang YN, Chen DQ, Wu XQ, Chen L, Guo Y, Zou L, Vaziri ND, Li P, et al. 2022. Intrarenal 1-methoxypyrene, an aryl hydrocarbon receptor agonist, mediates progressive tubulointerstitial fibrosis in mice. Acta Pharmacol Sin. 43(11):2929–2945. doi: 10.1038/s41401-022-00914-6.
- Cao M, Peng Y, Lu Y, Zou Z, Chen J, Bottino R, Knoll M, Zhang H, Lin S, Pu Z, et al. 2021. Controls of hyperglycemia improves dysregulated microbiota in diabetic mice. Transplantation. 105(9):1980–1988. doi: 10.1097/TP.0000000000003603.
- Chen Q, Ren D, Wu J, Yu H, Chen X, Wang J, Zhang Y, Liu M, Wang T. 2021. Shenyan Kangfu tablet alleviates diabetic kidney disease through attenuating inflammation and modulating the gut microbiota. J Nat Med. 75(1):84–98. doi: 10.1007/s11418-020-01452-3.
- Chen Y, Chen D, Chen L, Liu J, Vaziri N, Guo Y, Zhao Y. 2019. Microbiome-metabolome reveals the contribution of gut-kidney axis on kidney disease. J Transl Med. 17(1):5. doi: 10.1186/s12967-018-1756-4.
- Cheng X, Zhou T, He Y, Xie Y, Xu Y, Huang W. 2022. The role and mechanism of butyrate in the prevention and treatment of diabetic kidney disease. Front Microbiol. 13:961536. doi: 10.3389/fmicb.2022.961536.
- Chi M, Ma K, Wang J, Ding Z, Li Y, Zhu S, Liang X, Zhang Q, Song L, Liu C. 2021. The immunomodulatory effect of the gut microbiota in kidney disease. J Immunol Res. 2021:5516035. doi: 10.1155/2021/5516035.
- Chou C, Lin C, Chiu D, Chen I, Chen S. 2018. Tryptophan as a surrogate prognostic marker for diabetic nephropathy. J Diabetes Investig. 9(2):366–374. doi: 10.1111/jdi.12707.
- Cuív P, Smith WJ, Pottenger S, Burman S, Shanahan ER, Morrison M. 2015. Isolation of genetically tractable most-wanted bacteria by metaparental mating. Sci Rep. 5(1):13282. doi: 10.1038/srep13282.
- Dai Y, Quan J, Xiong L, Luo Y, Yi B. 2022. Probiotics improve renal function, glucose, lipids, inflammation and oxidative stress in diabetic kidney disease: a systematic review and meta-analysis. Ren Fail. 44(1):862–880. doi: 10.1080/0886022X.2022.2079522.
- Debnath N, Kumar R, Kumar A, Mehta P, Yadav A. 2021. Gut-microbiota derived bioactive metabolites and their functions in host physiology. Biotechnol Genet Eng Rev. 37(2):105–153. doi: 10.1080/02648725.2021.1989847.
- Deng L, Yang Y, Xu G. 2022. Empagliflozin ameliorates type 2 diabetes mellitus-related diabetic nephropathy via altering the gut microbiota. Biochim Biophys Acta Mol Cell Biol Lipids. 1867(12):159234. doi: 10.1016/j.bbalip.2022.159234.
- Du Y, Yang Y, Tang G, Jia J, Zhu N, Yuan W. 2020. Butyrate alleviates diabetic kidney disease by mediating the miR-7a-5p/P311/TGF-β1 pathway. FASEB J. 34(8):10462–10475. doi: 10.1096/fj.202000431R.
- Ellis R, Small D, Vesey D, Johnson D, Francis R, Vitetta L, Gobe G, Morais C. 2016. Indoxyl sulphate and kidney disease: causes, consequences and interventions. Nephrology. 21(3):170–177. doi: 10.1111/nep.12580.
- Fang Q, Zheng B, Liu N, Liu J, Liu W, Huang X, Zeng X, Chen L, Li Z, Ouyang D. 2021. Trimethylamine N-oxide exacerbates renal inflammation and fibrosis in rats with diabetic kidney disease. Front Physiol. 12:682482. doi: 10.3389/fphys.2021.682482.
- Feng Y, Cao G, Chen D, Vaziri N, Chen L, Zhang J, Wang M, Guo Y, Zhao Y. 2019. Microbiome-metabolomics reveals gut microbiota associated with glycine-conjugated metabolites and polyamine metabolism in chronic kidney disease. Cell Mol Life Sci. 76(24):4961–4978. doi: 10.1007/s00018-019-03155-9.
- Fernandes R, Viana S, Nunes S, Reis F. 2019. Diabetic gut microbiota dysbiosis as an inflammaging and immunosenescence condition that fosters progression of retinopathy and nephropathy. Biochim Biophys Acta Mol Basis Dis. 1865(7):1876–1897. doi: 10.1016/j.bbadis.2018.09.032.
- Fiaccadori E, Cosola C, Sabatino A. 2020. Targeting the gut for early diagnosis, prevention, and cure of diabetic kidney disease: is the phenyl sulfate story another step forward? Am J Kidney Dis. 75(1):144–147. doi: 10.1053/j.ajkd.2019.07.001.
- Fu X, Liu Z, Zhu C, Mou H, Kong Q. 2019. Nondigestible carbohydrates, butyrate, and butyrate-producing bacteria. Crit Rev Food Sci Nutr. 59(sup1):S130–S152. doi: 10.1080/10408398.2018.1542587.
- Gao J, Xu K, Liu H, Liu G, Bai M, Peng C, Li T, Yin Y. 2018. Impact of the gut microbiota on intestinal immunity mediated by tryptophan metabolism. Front Cell Infect Microbiol. 8:13. doi: 10.3389/fcimb.2018.00013.
- Gao Y, Yang R, Guo L, Wang Y, Liu W, Ai S, Woon T, Wang Z, Zhai Y, Wang Z, et al. 2021. Qing-Re-Xiao-Zheng formula modulates gut microbiota and inhibits inflammation in mice with diabetic kidney disease. Front Med. 8:719950. doi: 10.3389/fmed.2021.719950.
- Gembillo G, Ingrasciotta Y, Crisafulli S, Luxi N, Siligato R, Santoro D, Trifirò G. 2021. Kidney disease in diabetic patients: from pathophysiology to pharmacological aspects with a focus on therapeutic inertia. Int J Mol Sci. 22(9):4824. doi: 10.3390/ijms22094824.
- Giordano L, Mihaila S, Eslami Amirabadi H, Masereeuw R. 2021. Microphysiological systems to recapitulate the gut-kidney axis. Trends Biotechnol. 39(8):811–823. doi: 10.1016/j.tibtech.2020.12.001.
- Gonçalves P, Araújo J, Santo J. 2018. A cross-talk between microbiota-derived short-chain fatty acids and the host mucosal immune system regulates intestinal homeostasis and inflammatory bowel disease. Inflamm Bowel Dis. 24(3):558–572. doi: 10.1093/ibd/izx029.
- Gooding J, Cao L, Ahmed F, Mwiza J, Fernander M, Whitaker C, Acuff Z, McRitchie S, Sumner S, Ongeri E. 2019. LC-MS-based metabolomics analysis to identify meprin-β-associated changes in kidney tissue from mice with STZ-induced type 1 diabetes and diabetic kidney injury. Am J Physiol Renal Physiol. 317(4):F1034–F1046. doi: 10.1152/ajprenal.00166.2019.
- Gradisteanu G, Stoica R, Petcu L, Picu A, Suceveanu A, Salmen T, Stefan D, Serafinceanu C, Chifiriuc M, Stoian A. 2019. Microbiota signatures in type-2 diabetic patients with chronic kidney disease – a pilot study. J Mind Med Sci. 6(1):130–136. doi: 10.22543/7674.61.P130136.
- Gryp T, Vanholder R, Vaneechoutte M, Glorieux G. 2017. p-Cresyl sulfate. Toxins. 9(2):52. doi: 10.3390/toxins9020052.
- Han S, Chen M, Cheng P, Zhang Z, Lu Y, Xu Y, Wang Y. 2022. A systematic review and meta-analysis of gut microbiota in diabetic kidney disease: comparisons with diabetes mellitus, non-diabetic kidney disease, and healthy individuals. Front Endocrinol. 13:1018093. doi: 10.3389/fendo.2022.1018093.
- Han S, Song H, Cha J, Han J, Kang Y, Cha D. 2021. Farnesoid X receptor (FXR) agonist ameliorates systemic insulin resistance, dysregulation of lipid metabolism, and alterations of various organs in a type 2 diabetic kidney animal model. Acta Diabetol. 58(4):495–503. doi: 10.1007/s00592-020-01652-z.
- Hu ZB, Lu J, Chen PP, Lu CC, Zhang JX, Li XQ, Yuan BY, Huang SJ, Ruan XZ, Liu BC, et al. 2020. Dysbiosis of intestinal microbiota mediates tubulointerstitial injury in diabetic nephropathy via the disruption of cholesterol homeostasis. Theranostics. 10(6):2803–2816. doi: 10.7150/thno.40571.
- Huang W, Man Y, Gao C, Zhou L, Gu J, Xu H, Wan Q, Long Y, Chai L, Xu Y, et al. 2020. Short-chain fatty acids ameliorate diabetic nephropathy via GPR43-mediated inhibition of oxidative stress and NF-κB signaling. Oxid Med Cell Longev. 2020:4074832.
- Hwang Y, Kim S, Hur K, Cha B, Kim I, Park T, Baik S, Yoon K, Lee K, Lee I, et al. 2019. Predictive factors for efficacy of AST-120 treatment in diabetic nephropathy: a prospective single-arm, open-label, multi-center study. J Korean Med Sci. 34(15):e117. doi: 10.3346/jkms.2019.34.e117.
- Janeiro M, Ramírez M, Milagro F, Martínez J, Solas M. 2018. Implication of trimethylamine N-oxide (TMAO) in disease: potential biomarker or new therapeutic target. Nutrients. 10(10):1398. doi: 10.3390/nu10101398.
- Jb A, In B, Bi B, Mahk C, Zbz D, Arm E. 2021. Effect of probiotics on oxidative stress and inflammatory status in diabetic nephropathy: a systematic review and meta-analysis of clinical trials. Heliyon. 7(1):e05925.
- Jiang H, Zhang Y, Xu D, Wang Q. 2021. Probiotics ameliorates glycemic control of patients with diabetic nephropathy: a randomized clinical study. J Clin Lab Anal. 35(4):e23650.
- Johnson EL, Heaver SL, Walters WA, Ley RE. 2017. Microbiome and metabolic disease: revisiting the bacterial phylum Bacteroidetes. J Mol Med. 95(1):1–8. doi: 10.1007/s00109-016-1492-2.
- Kalagi NA, Thota RN, Stojanovski E, Alburikan KA, Garg ML. 2023. Plasma trimethylamine N-oxide levels are associated with poor kidney function in people with type 2 diabetes. Nutrients. 15(4):812. doi: 10.3390/nu15040812.
- Kikuchi K, Saigusa D, Kanemitsu Y, Matsumoto Y, Thanai P, Suzuki N, Mise K, Yamaguchi H, Nakamura T, Asaji K, et al. 2019. Gut microbiome-derived phenyl sulfate contributes to albuminuria in diabetic kidney disease. Nat Commun. 10(1):1835. doi: 10.1038/s41467-019-09735-4.
- Koh A, De Vadder F, Kovatcheva-Datchary P, Bäckhed F. 2016. From dietary fiber to host physiology: short-chain fatty acids as key bacterial metabolites. Cell. 165(6):1332–1345. doi: 10.1016/j.cell.2016.05.041.
- Kovatcheva-Datchary P, Nilsson A, Akrami R, Lee YS, De Vadder F, Arora T, Hallen A, Martens E, Björck I, Bäckhed F. 2015. Dietary fiber-induced improvement in glucose metabolism is associated with increased abundance of Prevotella. Cell Metab. 22(6):971–982. doi: 10.1016/j.cmet.2015.10.001.
- Krautkramer K, Fan J, Bäckhed F. 2021. Gut microbial metabolites as multi-kingdom intermediates. Nat Rev Microbiol. 19(2):77–94. doi: 10.1038/s41579-020-0438-4.
- Kuka J, Videja M, Makrecka-Kuka M, Liepins J, Grinberga S, Sevostjanovs E, Vilks K, Liepinsh E, Dambrova M. 2020. Metformin decreases bacterial trimethylamine production and trimethylamine N-oxide levels in db/db mice. Sci Rep. 10(1):14555. doi: 10.1038/s41598-020-71470-4.
- Kumar H, Kawai T, Akira S. 2011. Pathogen recognition by the innate immune system. Int Rev Immunol. 30(1):16–34. doi: 10.3109/08830185.2010.529976.
- La Reau AJ, Suen G. 2018. The Ruminococci: key symbionts of the gut ecosystem. J Microbiol. 56(3):199–208. doi: 10.1007/s12275-018-8024-4.
- Larsen JM. 2017. The immune response to Prevotella bacteria in chronic inflammatory disease. Immunology. 151(4):363–374. doi: 10.1111/imm.12760.
- Lau W, Chang Y, Vaziri N. 2021. The consequences of altered microbiota in immune-related chronic kidney disease. Nephrol Dial Transplant. 36(10):1791–1798. doi: 10.1093/ndt/gfaa087.
- Lee GT, Ha H, Jung M, Li H, Hong SW, Cha BS, Lee HC, Cho YD. 2003. Delayed treatment with lithospermate B attenuates experimental diabetic renal injury. J Am Soc Nephrol. 14(3):709–720. doi: 10.1097/01.asn.0000051660.82593.19.
- Lehto M, Groop P. 2018. The gut-kidney axis: putative interconnections between gastrointestinal and renal disorders. Front Endocrinol. 9:553. doi: 10.3389/fendo.2018.00553.
- Leong S, Sirich T. 2016. Indoxyl sulfate-review of toxicity and therapeutic strategies. Toxins. 8(12):358. doi: 10.3390/toxins8120358.
- Li L, Tao S, Ma L, Fu P. 2019. Roles of short-chain fatty acids in kidney diseases. Chin Med J. 132(10):1228–1232. doi: 10.1097/CM9.0000000000000228.
- Li Y, Qin GQ, Wang WY, Liu X, Gao XQ, Liu JH, Zheng T, Zhang W, Cheng L, Yang K, et al. 2022. Short chain fatty acids for the risk of diabetic nephropathy in type 2 diabetes patients. Acta Diabetol. 59(7):901–909. doi: 10.1007/s00592-022-01870-7.
- Li Y, Su X, Gao Y, Lv C, Gao Z, Liu Y, Wang Y, Li S, Wang Z. 2020b. The potential role of the gut microbiota in modulating renal function in experimental diabetic nephropathy murine models established in same environment. Biochim Biophys Acta Mol Basis Dis. 1866(6):165764. doi: 10.1016/j.bbadis.2020.165764.
- Li YJ, Chen X, Kwan TK, Loh YW, Singer J, Liu Y, Ma J, Tan J, Macia L, Mackay CR, et al. 2020a. Dietary fiber protects against diabetic nephropathy through short-chain fatty acid-mediated activation of G protein-coupled receptors GPR43 and GPR109A. J Am Soc Nephrol. 31(6):1267–1281. doi: 10.1681/ASN.2019101029.
- Lin JR, Wang ZT, Sun JJ, Yang YY, Li XX, Wang XR, Shi Y, Zhu YY, Wang RT, Wang MN, et al. 2022. Gut microbiota and diabetic kidney diseases: pathogenesis and therapeutic perspectives. World J Diabetes. 13(4):308–318. doi: 10.4239/wjd.v13.i4.308.
- Liu J, Gao LD, Fu B, Yang HT, Zhang L, Che SQ, Xu Y, Du X, Liu ZC, Xue Y, et al. 2022. Efficacy and safety of Zicuiyin decoction on diabetic kidney disease: a multicenter, randomized controlled trial. Phytomedicine. 100:154079. doi: 10.1016/j.phymed.2022.154079.
- Liu J, Miao H, Deng D, Vaziri N, Li P, Zhao Y. 2021. Gut microbiota-derived tryptophan metabolism mediates renal fibrosis by aryl hydrocarbon receptor signaling activation. Cell Mol Life Sci. 78(3):909–922. doi: 10.1007/s00018-020-03645-1.
- Lopes R, Balbino K, Jorge M, Ribeiro A, Martino H, Alfenas R. 2018. Modulation of intestinal microbiota, control of nitrogen products and inflammation by pre/probiotics in chronic kidney disease: a systematic review. Nutr Hosp. 35(3):722–730. doi: 10.20960/nh.1642.
- Lu C, Hu Z, Wang R, Hong Z, Lu J, Chen P, Zhang J, Li X, Yuan B, Huang S, et al. 2020. Gut microbiota dysbiosis-induced activation of the intrarenal renin-angiotensin system is involved in kidney injuries in rat diabetic nephropathy. Acta Pharmacol Sin. 41(8):1111–1118. doi: 10.1038/s41401-019-0326-5.
- Lu C, Ma K, Ruan X, Liu B. 2018. Intestinal dysbiosis activates renal renin-angiotensin system contributing to incipient diabetic nephropathy. Int J Med Sci. 15(8):816–822. doi: 10.7150/ijms.25543.
- Lu J, Chen P, Zhang J, Li X, Wang G, Yuan B, Huang S, Liu X, Jiang T, Wang M, et al. 2021. GPR43 deficiency protects against podocyte insulin resistance in diabetic nephropathy through the restoration of AMPKα activity. Theranostics. 11(10):4728–4742. doi: 10.7150/thno.56598.
- Luo LP, Suo P, Ren LL, Liu HJ, Zhang Y, Zhao YY. 2021. Shenkang injection and its three anthraquinones ameliorates renal fibrosis by simultaneous targeting IƙB/NF-ƙB and Keap1/Nrf2 signaling pathways. Front Pharmacol. 12:800522. doi: 10.3389/fphar.2021.800522.
- Lv Q, Li Z, Sui A, Yang X, Han Y, Yao R. 2022. The role and mechanisms of gut microbiota in diabetic nephropathy, diabetic retinopathy and cardiovascular diseases. Front Microbiol. 13:977187. doi: 10.3389/fmicb.2022.977187.
- Lymperopoulos A, Suster MS, Borges JI. 2022. Short-chain fatty acid receptors and cardiovascular function. Int J Mol Sci. 23(6):3303. doi: 10.3390/ijms23063303.
- Mafi A, Namazi G, Soleimani A, Bahmani F, Aghadavod E, Asemi Z. 2018. Metabolic and genetic response to probiotics supplementation in patients with diabetic nephropathy: a randomized, double-blind, placebo-controlled trial. Food Funct. 9(9): 4763–4770. doi: 10.1039/c8fo00888d.
- Marquardt A, Al-Dabet M, Ghosh S, Kohli S, Manoharan J, ElWakiel A, Gadi I, Bock F, Nazir S, Wang H, et al. 2017. Farnesoid X receptor agonism protects against diabetic tubulopathy: potential add-on therapy for diabetic nephropathy. J Am Soc Nephrol. 28(11):3182–3189. doi: 10.1681/ASN.2016101123.
- Meng X, Ma J, Kang A, Kang S, Jung H, Park Y. 2020a. A novel approach based on metabolomics coupled with intestinal flora analysis and network pharmacology to explain the mechanisms of action of Bekhogainsam Decoction in the improvement of symptoms of streptozotocin-induced diabetic nephropathy in mice. Front Pharmacol. 11:633. doi: 10.3389/fphar.2020.00633.
- Meng X, Ma J, Kang S, Jung H, Park Y. 2020b. Jowiseungki decoction affects diabetic nephropathy in mice through renal injury inhibition as evidenced by network pharmacology and gut microbiota analyses. Chin Med. 15(1):24. doi: 10.1186/s13020-020-00306-0.
- Miao H, Zhang YM, Yu XY, Zou L, Zhao YY. 2022. Membranous nephropathy: systems biology-based novel mechanism and traditional Chinese medicine therapy. Front Pharmacol. 13:969930. doi: 10.3389/fphar.2022.969930.
- Miraghajani M, Zaghian N, Dehkohneh A, Mirlohi M, Ghiasvand R. 2019. Probiotic soy milk consumption and renal function among type 2 diabetic patients with nephropathy: a randomized controlled clinical trial. Probiotics Antimicrob Proteins. 11(1):124–132. doi: 10.1007/s12602-017-9325-3.
- Miraghajani M, Zaghian N, Mirlohi M, Feizi A, Ghiasvand R. 2017. The impact of probiotic soy milk consumption on oxidative stress among type 2 diabetic kidney disease patients: a randomized controlled clinical trial. J Ren Nutr. 27(5):317–324. doi: 10.1053/j.jrn.2017.04.004.
- Mishima E, Fukuda S, Mukawa C, Yuri A, Kanemitsu Y, Matsumoto Y, Akiyama Y, Fukuda N, Tsukamoto H, Asaji K, et al. 2017. Evaluation of the impact of gut microbiota on uremic solute accumulation by a CE-TOFMS-based metabolomics approach. Kidney Int. 92(3):634–645. doi: 10.1016/j.kint.2017.02.011.
- Moon S, Tsay JJ, Lampert H, Md Dom ZI, Kostic AD, Smiles A, Niewczas MA. 2021. Circulating short and medium chain fatty acids are associated with normoalbuminuria in type 1 diabetes of long duration. Sci Rep. 11(1):8592. doi: 10.1038/s41598-021-87585-1.
- Moravejolahkami AR, Kermani M, Zehi ZB, Mirenayat S, Mansourian M. 2021. The effect of probiotics on lipid profile & anthropometric indices in diabetic nephropathy; a systematic review and meta-analysis of clinical trials. J Diabetes Metab Disord. 20(1):893–904. doi: 10.1007/s40200-021-00765-8.
- Mosterd C, Kanbay M, van den Born B, van Raalte D, Rampanelli E. 2021. Intestinal microbiota and diabetic kidney diseases: the role of microbiota and derived metabolites inmodulation of renal inflammation and disease progression. Best Pract Res Clin Endocrinol Metab. 35(3):101484. doi: 10.1016/j.beem.2021.101484.
- Nagase N, Ikeda Y, Tsuji A, Kitagishi Y, Matsuda S. 2022. Efficacy of probiotics on the modulation of gut microbiota in the treatment of diabetic nephropathy. World J Diabetes. 13(3):150–160. doi: 10.4239/wjd.v13.i3.150.
- Navid MA, Zahra ED, Hamid T, Reza SC, Alireza S, Zatollah A. 2019. The effects of probiotic honey consumption on metabolic status in patients with diabetic nephropathy: a randomized, double-blind, controlled trial. Probiotics Antimicrob Proteins. 11:1195–1201.
- Ni Y, Zheng L, Nan S, Ke L, Fu Z, Jin J. 2022. Enterorenal crosstalks in diabetic nephropathy and novel therapeutics targeting the gut microbiota. Acta Biochim Biophys Sin. 54(10):1406–1420. doi: 10.3724/abbs.2022140.
- Oliphant K, Allen-Vercoe E. 2019. Macronutrient metabolism by the human gut microbiome: major fermentation by-products and their impact on host health. Microbiome. 7(1):91. doi: 10.1186/s40168-019-0704-8.
- Patel D, Bose M, Cooper M. 2020. Glucose and blood pressure-dependent pathways-the progression of diabetic kidney disease. Int J Mol Sci. 21(6):2218. doi: 10.3390/ijms21062218.
- Paul P, Kaul R, Chaari A. 2022. Renal health improvement in diabetes through microbiome modulation of the gut-kidney axis with biotics: a systematic and narrative review of randomized controlled trials. Int J Mol Sci. 23(23):14838. doi: 10.3390/ijms232314838.
- Persson P, Palm F. 2017. Hypoxia-inducible factor activation in diabetic kidney disease. Curr Opin Nephrol Hypertens. 26(5):345–350. doi: 10.1097/MNH.0000000000000341.
- Phull AR, Ali A, Rafiq M, Tahir T, Majid A, Seo SY, Park HJ. 2021. Antioxidant potential, urease and acetylcholine esterase inhibitory activity and phytochemical analysis of selected medicinal plants from the Republic of Korea. Exp Res Hypot Med. 6:51–59.
- Pugin B, Barcik W, Westermann P, Heider A, Wawrzyniak M, Hellings P, Akdis C, O’Mahony L. 2017. A wide diversity of bacteria from the human gut produces and degrades biogenic amines. Microb Ecol Health Dis. 28(1):1353881. doi: 10.1080/16512235.2017.1353881.
- Qi C, Mao X, Zhang Z, Wu H. 2017. Classification and differential diagnosis of diabetic nephropathy. J Diabetes Res. 2017(2017):8637138–8637137. doi: 10.1155/2017/8637138.
- Rysz J, Franczyk B, Ławiński J, Olszewski R, Ciałkowska-Rysz A, Gluba-Brzózka A. 2021. The impact of CKD on uremic toxins and gut microbiota. Toxins. 13(4):252. doi: 10.3390/toxins13040252.
- Saleem T, Dahpy M, Ezzat G, Abdelrahman G, Abdel-Aziz E, Farghaly R. 2019. The profile of plasma free amino acids in type 2 diabetes mellitus with insulin resistance: association with microalbuminuria and macroalbuminuria. Appl Biochem Biotechnol. 188(3):854–867. doi: 10.1007/s12010-019-02956-9.
- Salguero M, Al-Obaide M, Singh R, Siepmann T, Vasylyeva T. 2019. Dysbiosis of gram-negative gut microbiota and the associated serum lipopolysaccharide exacerbates inflammation in type 2 diabetic patients with chronic kidney disease. Exp Ther Med. 18(5):3461–3469. doi: 10.3892/etm.2019.7943.
- Sato E, Hosomi K, Sekimoto A, Mishima E, Oe Y, Saigusa D, Ito S, Abe T, Sato H, Kunisawa J, et al. 2020. Effects of the oral adsorbent AST-120 on fecal p-cresol and indole levels and on the gut microbiota composition. Biochem Biophys Res Commun. 525(3):773–779. doi: 10.1016/j.bbrc.2020.02.141.
- Seong CN, Kang JW, Lee JH, Seo SY, Woo JJ, Park C, Bae KS, Kim MS. 2018. Taxonomic hierarchy of the phylum Firmicutes and novel Firmicutes species originated from various environments in Korea. J Microbiol. 56(1):1–10. doi: 10.1007/s12275-018-7318-x.
- Shi R, Tao Y, Tang H, Wu C, Fei J, Ge H, Gu HF, Wu J. 2022. Abelmoschus manihot ameliorates the levels of circulating metabolites in diabetic nephropathy by modulating gut microbiota in non-obese diabetes mice. Microb Biotechnol. 16(4):813–826. doi: 10.1111/1751-7915.14200.
- Singh H, Miyamoto S, Darshi M, Torralba M, Kwon K, Sharma K, Pieper R. 2020. Gut microbial changes in diabetic db/db mice and recovery of microbial diversity upon Pirfenidone treatment. Microorganisms. 8(9):1347. doi: 10.3390/microorganisms8091347.
- Snelson M, Tan S, Higgins G, Lindblom R, Coughlan M. 2020. Exploring the role of the metabolite-sensing receptor GPR109a in diabetic nephropathy. Am J Physiol Renal Physiol. 318(3):F835–F842. doi: 10.1152/ajprenal.00505.2019.
- Su X, Yu W, Liu A, Wang C, Li X, Gao J, Liu X, Jiang W, Yang Y, Lv S. 2021. San-Huang-Yi-Shen capsule ameliorates diabetic nephropathy in rats through modulating the gut microbiota and overall metabolism. Front Pharmacol. 12:808867. doi: 10.3389/fphar.2021.808867.
- Sun G, Yin Z, Liu N, Bian X, Yu R, Su X, Zhang B, Wang Y. 2017. Gut microbial metabolite TMAO contributes to renal dysfunction in a mouse model of diet-induced obesity. Biochem Biophys Res Commun. 493(2):964–970. doi: 10.1016/j.bbrc.2017.09.108.
- Tan Y, Gao Y, Teo G, Koh H, Tai E, Khoo C, Choi K, Zhou L, Choi H. 2021. Plasma metabolome and lipidome associations with type 2 diabetes and diabetic nephropathy. Metabolites. 11(4):228. doi: 10.3390/metabo11040228.
- Tang G, Du Y, Guan H, Jia J, Zhu N, Shi Y, Rong S, Yuan W. 2022. Butyrate ameliorates skeletal muscle atrophy in diabetic nephropathy by enhancing gut barrier function and FFA2-mediated PI3K/Akt/mTOR signals. Br J Pharmacol. 179(1):159–178. doi: 10.1111/bph.15693.
- Thomas RJ, Kim H, Maillard P, Decarli CS, Heckman EJ, Karjadi C, Fang T, Ang A, Au R. 2021. Digital sleep measures and white matter health in the Framingham Heart Study. Explor Med. 2(3):253–267. doi: 10.37349/emed.2021.00045.
- Tofte N, Vogelzangs N, Mook-Kanamori D, Brahimaj A, Nano J, Ahmadizar F, van Dijk K, Frimodt-Møller M, Arts I, Beulens J, et al. 2020. Plasma metabolomics identifies markers of impaired renal function: a meta-analysis of 3089 persons with type 2 diabetes. J Clin Endocrinol Metab. 105(7):2275–2287. doi: 10.1210/clinem/dgaa173.
- Toyohara T, Suzuki T, Morimoto R, Akiyama Y, Souma T, Shiwaku H, Takeuchi Y, Mishima E, Abe M, Tanemoto M, et al. 2009. SLCO4C1 transporter eliminates uremic toxins and attenuates hypertension and renal inflammation. J Am Soc Nephrol. 20(12):2546–2555. doi: 10.1681/ASN.2009070696.
- Velasquez MT, Ramezani A, Manal A, Raj DS. 2016. Trimethylamine N-oxide: the good, the bad and the unknown. Toxins. 8(11):326. doi: 10.3390/toxins8110326.
- Vlachou E, Ntikoudi A, Govina O, Lavdaniti M, Kotsalas N, Tsartsalis A, Dimitriadis G. 2020. Effects of probiotics on diabetic nephropathy: a systematic review. Curr Clin Pharmacol. 15(3):234–242. doi: 10.2174/1574884715666200303112753.
- Walker A, Schmitt-Kopplin P. 2021. The role of fecal sulfur metabolome in inflammatory bowel diseases. Int J Med Microbiol. 311(5):151513. doi: 10.1016/j.ijmm.2021.151513.
- Wang P, Wang T, Zheng X, Cui W, Shang J, Zhao Z. 2021. Gut microbiota, key to unlocking the door of diabetic kidney disease. Nephrology. 26(8):641–649. doi: 10.1111/nep.13874.
- Wang X, Edelstein M, Gafter U, Qiu L, Luo Y, Dobrinskikh E, Lucia S, Adorini L, D’Agati V, Levi J, et al. 2016. G protein-coupled bile acid receptor TGR5 activation inhibits kidney disease in obesity and diabetes. J Am Soc Nephrol. 27(5):1362–1378. doi: 10.1681/ASN.2014121271.
- Wang X, Wang D, Luo Y, Myakala K, Dobrinskikh E, Rosenberg A, Levi J, Kopp J, Field A, Hill A, et al. 2018. FXR/TGR5 dual agonist prevents progression of nephropathy in diabetes and obesity. J Am Soc Nephrol. 29(1):118–137. doi: 10.1681/ASN.2017020222.
- Wang Y, Liu H, Ren L, Suo P, Zou L, Zhang Y, Yu X, Zhao Y. 2022a. Shenkang injection improves chronic kidney disease by inhibiting multiple renin-angiotensin system genes by blocking the Wnt/β-catenin signalling pathway. Front Pharmacol. 13:964370. doi: 10.3389/fphar.2022.964370.
- Wang Y, Zhang Z, Liu H, Guo Z, Zou L, Zhang Y, Zhao Y. 2022b. Integrative phosphatidylcholine metabolism through phospholipase A2 in rats with chronic kidney disease. Acta Pharmacol Sin. 44(2):393–405. doi: 10.1038/s41401-022-00947-x.
- Wang Y, Zhao J, Qin Y, Yu Z, Zhang Y, Ning X, Sun S. 2022c. the specific alteration of gut microbiota in diabetic kidney diseases–a systematic review and meta-analysis. Front Immunol. 13:908219. doi: 10.3389/fimmu.2022.908219.
- Wei H, Wang L, An Z, Xie H, Liu W, Du Q, Guo Y, Wu X, Li S, Shi Y, et al. 2021. QiDiTangShen granules modulated the gut microbiome composition and improved bile acid profiles in a mouse model of diabetic nephropathy. Biomed Pharmacother. 133:111061. doi: 10.1016/j.biopha.2020.111061.
- Wexler HM. 2007. Bacteroides: the good, the bad, and the nitty-gritty. Clin Microbiol Rev. 20(4):593–621. doi: 10.1128/CMR.00008-07.
- Winther S, Øllgaard J, Tofte N, Tarnow L, Wang Z, Ahluwalia T, Jorsal A, Theilade S, Parving H, Hansen T, et al. 2019. Utility of plasma concentration of trimethylamine N-oxide in predicting cardiovascular and renal complications in individuals with type 1 diabetes. Diabetes Care. 42(8):1512–1520. doi: 10.2337/dc19-0048.
- Winther SA, Øllgaard JC, Hansen TW, von Scholten BJ, Reinhard H, Ahluwalia TS, Wang Z, Gæde P, Parving HH, Hazen S, et al. 2021. Plasma trimethylamine N-oxide and its metabolic precursors and risk of mortality, cardiovascular and renal disease in individuals with type 2-diabetes and albuminuria. PLOS One. 16(3):e0244402. doi: 10.1371/journal.pone.0244402.
- Wu C, Fei J, Xu Q, Tao Y, Zhou Z, Wang Y, Wu J, Gu HF. 2022. Interaction between plasma metabolomics and intestinal microbiome in db/db mouse, an animal model for study of type 2 diabetes and diabetic kidney disease. Metabolites. 12(9):775. doi: 10.3390/metabo12090775.
- Xiao H, Sun X, Liu R, Chen Z, Lin Z, Yang Y, Zhang M, Liu P, Quan S, Huang H. 2020. Gentiopicroside activates the bile acid receptor Gpbar1 (TGR5) to repress NF-kappaB pathway and ameliorate diabetic nephropathy. Pharmacol Res. 151:104559. doi: 10.1016/j.phrs.2019.104559.
- Xu KY, Xia GH, Lu JQ, Chen MX, Zhen X, Wang S, You C, Nie J, Zhou HW, Yin J. 2017. Impaired renal function and dysbiosis of gut microbiota contribute to increased trimethylamine-N-oxide in chronic kidney disease patients. Sci Rep. 7(1):1445. doi: 10.1038/s41598-017-01387-y.
- Yang MX, Zhang R, Zhuang CF, Wu YY, Yang Q, Yu ZY, Liu J, Zha BB, Gong QH, Yang B, et al. 2021. Serum trimethylamine N-oxide and the diversity of the intestinal microbial flora in type 2 diabetes complicated by diabetic kidney disease. Clin Lab. 68(5):2373–2382. doi: 10.7754/Clin.Lab.2021.210836.
- Yu XY, Sun Q, Zhang YM, Zou L, Zhao YY. 2022. TGF-β/Smad signaling pathway in tubulointerstitial fibrosis. Front Pharmacol. 13:860588. doi: 10.3389/fphar.2022.860588.
- Zaky A, Glastras SJ, Wong MYW, Pollock CA, Saad S. 2021. The role of the gut microbiome in diabetes and obesity-related kidney disease. Int J Mol Sci. 22(17):9641. doi: 10.3390/ijms22179641.
- Zhang J, Fu Y, Li L, Liu Y, Zhang C, Yu D, Ma Y, Xiao Y. 2019. Pharmacokinetic comparisons of major bioactive components after oral administration of raw and steamed rhubarb by UPLC-MS/MS. J Pharm Biomed Anal. 171:43–51. doi: 10.1016/j.jpba.2019.04.002.
- Zhang L, Wang Z, Zhang X, Zhao L, Chu J, Li H, Sun W, Yang C, Wang H, Dai W, et al. 2022. Alterations of the gut microbiota in patients with diabetic nephropathy. Microbiol Spectr. 10(4):e0032422. doi: 10.1128/spectrum.00324-22.
- Zhao J, Zhang Q, Shen J, Wang K, Liu J. 2019. Magnesium lithospermate B improves the gut microbiome and bile acid metabolic profiles in a mouse model of diabetic nephropathy. Acta Pharmacol Sin. 40(4):507–513. doi: 10.1038/s41401-018-0029-3.
- Zhao T, Zhang H, Yin X, Zhao H, Ma L, Yan M, Peng L, Wang Q, Dong X, Li P. 2020. Tangshen formula modulates gut microbiota and reduces gut-derived toxins in diabetic nephropathy rats. Biomed Pharmacother. 129:110325. doi: 10.1016/j.biopha.2020.110325.
- Zhao YY. 2022. Recent advances of gut microbiota in chronic kidney disease patients. Explor Med. 3:260–274. doi: 10.37349/emed.2022.00090.
- Zhong C, Bai X, Chen Q, Ma Y, Li J, Zhang J, Luo Q, Cai K. 2022. Gut microbial products valerate and caproate predict renal outcome among the patients with biopsy-confirmed diabetic nephropathy. Acta Diabetol. 59(11):1469–1477. doi: 10.1007/s00592-022-01948-2.
- Zhong C, Dai Z, Chai L, Wu L, Li J, Guo W, Zhang J, Zhang Q, Xue C, Lin H, et al. 2021. The change of gut microbiota-derived short-chain fatty acids in diabetic kidney disease. J Clin Lab Anal. 35(12):e24062.
- Zhou Q, Yang F, Li Z, Qu Q, Zhao C, Liu X, Yang P, Han L, Shi Y, Shi X. 2022. Paecilomyces cicadae-fermented Radix Astragali ameliorate diabetic nephropathy in mice by modulating the gut microbiota. J Med Microbiol. 71(5):1–10.