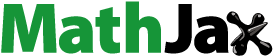
ABSTRACT
Cameroon’s electricity mix is dominated by hydropower sources despite the huge potentials of other sources like solar. The over-dependence on hydropower has led to frequent load shedding especially in the dry season as a result of low water levels. Interestingly, the government plans to diversify her power mix and provide sustainable energy to Cameroonians. It is in this framework that this study evaluates the integration of solar PV into the Southern Interconnected Grid of Cameroon using dynamic analysis. The ETAP tool was used in developing the model. A power loss assessment was done through successive injection of solar PV into the candidate busbars while monitoring the active power losses in the grid. Then, a transient stability analysis was conducted so as to assess the grid’s response to induced instability after the penetration of large-scale solar PV. The study showed that the use of static performance indices to evaluate the solar PV hosting capacity do not safeguard the grid’s technical robustness. The results indicated an optimum solar PV penetration level of 30% (211.8 MW) at the Ngousso 93 kV busbar. The findings will be useful to system operators and regulators in developing low-carbon pathways in the electricity network in Cameroon.
1. Introduction
Grid capacity expansion in a conventional manner would involve placing new transmission lines and addition of transformers with higher power-carrying capacity (Iweh et al. Citation2021). However, due to power quality issues, there exist a series of supplementary technical options for increasing renewable energy (RE) penetration today: some centred on the use of power equipment that are flexible and others based on intensive utilisation of smart technology (Aman et al. Citation2013). The trends in the power grid predict increased installation of distributed generators (DGs) in the near future due to the role they play in system reliability, grid flexibility, system efficiency and power system diversity (Abujubbeh and Fahrioglu Citation2019). Previously, storing power was a huge challenge as generated energy was used up by loads directly, in so doing compelling system operators to continuously find ways to sustain the tradeoff between power demand and supply (Baker Citation2000). The use of fossil fuel-based power plants has necessitated the injection of renewables into the grid as a means to reduce the environmental impacts of emissions from these plants. However, the integration of electricity from renewable sources into the power grid, could potentially pose a problem to the stability of the network (Baker Citation2000). For instance, arbitrarily placing renewables in the network could lead to increased power loss and voltage instability situation in the grid (Bayer and Marian Citation2020). Therefore, the integration of renewables requires adequately conducting analysis to determine the capacity and the point of connection to the grid. Literature on grid integration of renewables has raised concerns on the so much emphasis on deploying technology, rather than the necessary adaptations to sail through this transition (International Energy Agency / Organisation for Economic Co-operation and Development (IEA/OECD) Citation2014). Scholars have recognised the benefits of these technologies in the energy network (Lund et al. Citation2015; Lynch, Tol, and O’Malley Citation2012). However, the subject of integrating renewables into the power system has not been adequately addressed, particularly in developing nations (Iweh et al. Citation2021). Most of these countries have rich natural resource potential that can support the development of variable renewables and their subsequent integration into the grid. For Cameroon, hydropower has dominated electricity generation with approximately 53.7 TWh, representing 63% of the total electricity production (Energy of Cameroon (ENEO) Citation2019). This makes the Cameroonian electricity mix different from many countries. Nevertheless, this is equally a high risk because of the dependence of hydropower on weather conditions. A clear evidence is the frequent power outages in the dry season due to low water levels at Songloulou and the Edea Hydropower stations. Therefore, there is a need for research to explore the expansion of a diversified energy matrix, in order to reduce the over-dependence on hydropower.
Several studies have been conducted by various researchers in an attempt to explore and lessen the impacts of distributed generator (DG) integration into the grid. Grids located in remote areas have lower hosting capacity values than urban grids because of their weak topology and electrical features causing overvoltage challenges at extensive feeder terminals (Asano, Yajima, and Kaya Citation1996). The impacts of high solar PV penetration were used in Asano, Yajima, and Kaya (Citation1996) to evaluate how this affects the grid’s frequency regulation. It was observed that the solar PV break-even cost was high and only reduced when the solar PV penetration level became greater than or equal to 10%. Thus, solar PV penetration level needed to be augmented while simultaneously assessing and mitigating the impacts (Latheef et al. Citation2006). Another study in Chant et al. (Citation2011) has examined the challenges encountered when injecting small-scale solar PV in urban grids. They found that increasing solar PV injection caused voltage increase on the low-voltage (LV) network, rise in harmonic distortion and, eventually load rejection. The authors in Luhmann et al. (Citation2015) suggested a means of up scaling solar-wind-biomass systems into the German grid using low-cost solutions. In order to determine the amount of renewable energy (RE) penetration into the modelled grid, they iteratively added quantities of the DGs into the grid until the system could no longer support (voltage stability limits or transformers and cables loading limits). Another study was conducted by Shafiullah et al. (Citation2014) in Australia with the aim of assessing possibilities for integrating rooftop solar PV in the distribution network. They used the ETAP software to perform analysis and their major findings were that solar PV injection from customer buildings caused network issues such as overload of distribution transformers, voltage instabilities, reactive power compensation, phase imbalance and harmonic injections. In a study conducted by Rajaram, Sathish Kumar, and Rajasekar (Citation2015), modified plant growth algorithm (MPGA) was used to simulate a 33-bus distribution system of radial architecture on MatLab. Their aim was to find optimal ways in which rooftop solar systems or wind turbines could be connected to the distribution network and hence promote bidirectional power flow. After comparing the results obtained with other algorithms such as genetic algorithm (GA), plant growth simulation algorithm (PGSA) and refined genetic algorithm (RGA), they realised that their proposed approach gave the least reduction in real power loss and the solution converged faster. Similar studies were conducted by Muntathir and Chokri (Citation2020) on the optimum placement and penetration levels of photovoltaic (PV) systems on an IEEE 30-bus system by using the Electrical Transient Analysis Program (ETAP). They reported a 50% penetration level and concluded that this amount was satisfactory. While this research has considered a critical aspect of integrating variable RE into the power network, it has however, used a somewhat ideal network (IEEE – 30 Bus) which truncates the grip on what could be obtained on a real-life power network.
It is observed from the reviewed literature that several studies were conducted mostly in the United States, Australia and Europe. However, the features of the Cameroonian grid are not similar to those of developed countries in terms of architecture, loading (customer density) and management. Consequently, studies done in other countries cannot be directly applied and adopted without additional study that suits the Cameroonian context. Besides, most of the power generated from DGs is injected into distribution networks. This is a good engineering practice for DGs to be closer to load centres as this reduces the possibility of power to be transmitted over long distances with high potential of losses. However, most distribution grids are fragile and have a radial architecture especially in developing countries, with a low short-circuit capacity. Hence, power injection in these networks is relatively limited if one borders not to compromise the power quality and the stability of the network. In order to mitigate this difficulty, EHV, HV and MV are used to inject DGs since they are reliable and have a relatively high short-circuit capacity as opposed to distribution networks. This permits the penetration of more DG capacity into the transmission grid. Nonetheless, these transmission grids still have a limit to which power can be injected and hence, there is a need to determine the possible permissible DG penetration level on this network. Grid systems equally considered in most studies are test beds of IEEE with limited studies on real-life grids. These test beds of IEEE only mimic the functionality of grid operation and could potentially ignore the plethora of challenges encountered in a real-life grid system. Also, most of the DG hosting capacity studies found in the literature have used only steady-state analyses. The DG hosting capacity depends on performance indices such as the state of the network, reference parameters, network topology and the solar PV injection scenario conception. Hence, the value obtained as hosting capacity depends on the selection of suitable indices and method (Ismael et al. Citation2019). The method used in the study has adapted the application of transient stability analysis to further confirm the suitability of the selected busbar for solar PV interconnection to the grid as opposed to the commonly used power loss minimisation and voltage profile enhancement. This study shows that determining the DG hosting capacity based exclusively on static parameters (voltage limits violation, thermal overloading and power loss) is insufficient, as this capacity is only theoretical, and would lead to instabilities when the grid is subjected to extreme operational conditions. A summary of the contribution and novelty presented in this study are as follows:
Construct a procedural approach for assessing the solar PV hosting capacity of an interconnected grid with the consideration of dynamic analysis.
Evaluate the grid’s technical robustness against substantial disturbances under optimum solar PV penetration.
Present a systematic path to the injection of distributed solar PV for the growing loads to address the need to decarbonise the grid, which would, ultimately reduce emissions from backup thermal plants.
The paper presents an analytical approach to identifying the location and capacity of a distributed solar PV with the aim of minimising power losses. This approach can easily be adapted to the integration of different types of renewables for power loss minimisation. The proposed method can be used globally; however, the simulation has been applied on the Southern Interconnected Grid of Cameroon. Nonetheless, no studies have been conducted on the Cameroon grid in order to assess possibilities of the integration of solar PV into the national grid. Therefore, along the lines of government’s agenda to install 25% of renewables in the country’s power generation mix by 2035, this study develops a model using ETAP to investigate the level of solar PV penetration into the HV and MV of the SIG.
The maximum hosting capacity determined from the ETAP model was 30% at the Ngousso 93 kV bus with an active power loss of 26.234 MW. The DG hosting capacity of any grid is the highest DG generation capacity that can be reliably connected to the power system without the possibility of violating technical parameters in the network. The results obtained after transient stability at this busbar showed no stability limit violations. The results will assist the power utility (ENEO) and the public to build an environmentally friendly grid. The starts with a background of the solar PV integration into the grid where literature surrounding the subject is explored in order to situate the problem and clearly state the contribution of the study. The adopted method then followed. The method explained pertinent considerations used in the model conception. The results are then presented with the discussion of the findings. Finally, the study ends with a conclusion.
1.1. Stability of the electric power system
Grid stability is the ability of the grid to sustain an operational equilibrium state after being perturbed (Rodriguez and Amaratunga Citation2004). After perturbations, the machine’s rotor will swing before the governor intervenes. During the governor intervention, the rotor will oscillate until the machine’s state of synchronism is lost. A power system with a stable voltage implies that the system is able to sustain steady bus voltages in the network after perturbation from the initial operating conditions (Kundur et al. Citation2004). Perturbations could be small (changes in the daily load) or large (the outage of an equipment). shows the system stability versus time curve.
The perturbations may last from less than a second to several minutes, potentially causing either short-term or long-term voltage instability issues (Kundur et al. Citation2004). For voltage stability issues that last for short-term periods, their common causes include rapid variation in loads such as loads that are controlled by electronic means, induction motors and HVDC converters. Alternatively, voltage stability issues that last for long-term periods have common causes such as components with a slow response capability like loads controlled by thermostatic means, tap changing transformers and current limiters at the level of the generator. The origin of these long-term instabilities stems from insufficient reactive power at buses or other parts of the grid (Andersson Citation2008) extending for several minutes.
2. Modelling approach
The study used historical data of the SIG comprising of generator ratings, transformer ratings, line length and current capacities, and capacitor bank ratings. The modelling and simulation of the power system were done in the Electrical Transient Analyzer Program (ETAP). ETAP is one of the complete power simulation tools with robust capabilities in grid design, generation analysis, power transmission and distribution. ETAP has witnessed applications in power system studies in some developing countries (Moh, Sujito, and Made Citation2020; Airoboman et al. Citation2019; Sajid, Iftikhar, and Rehman Citation2019). The tool has been extensively used in RE integration (Muntathir and Chokri Citation2020; Shrestha et al. Citation2017; Sibildas Citation2018; Khan et al. Citation2019; Mujtaba et al. Citation2020), load flow analysis (Abubakar et al. Citation2019; Barsoum and Lee Citation2013; Bamdad Citation2014; Sukumaran et al. Citation2018), power system contingency studies (Moh, Sujito, and Made Citation2020; Airoboman et al. Citation2019; Sucita and Mulyadi Citation2017), transient stability analysis (Refaat, Abu-Rub, and Mohamed Citation2017) and micro-grid design (Bista Citation2019; AL-REFAI Citation2016; Mosbah Citation2018). Load flow analysis using Newton–Raphson’s algorithm was applied on the network, first, without the RE injection (pre-integration) in order to assess the system’s ability to satisfactorily feed the load and avert overloading of equipment. The power flow helped in analysing the system states at no contingencies (i.e. normal operation mode). This was followed by solar PV injection scenarios while monitoring the active power loss, transient stability and voltage limits of busbars. Candidate busbars for the solar PV injection were buses that had either heavy loads or connected to overloaded transmission lines. The solar PV was injected at intervals of 10% and continued up to 100%. shows the schematic of the methodology.
2.1. Case study description
The SIG is powered by three main hydroelectric plants (Song Loulou, Memve‘ele and Edéa) with an installed power of 859 MW. These hydropower stations are supported by thermal generators with a total installed capacity of 564.9 MW and these thermal plants use Heavy/Light Fuel Oil and natural gas as fuel. The total installed power in the SIG is 1423.9 MW (Energy of Cameroon (ENEO) Citation2019). The SIG has a transmission system consisting of 90 kV lines of length of 1064 km (68% of the entire network) and 225 kV lines of length 480 km (32% of the network). This transmission grid serves about 20 substations that relay power to the distribution network (Energy of Cameroon (ENEO) Citation2019). The peak load in the network has grown from 670 MW to 880 MW within 2012–2019 and this represents an annual growth rate of 5.4%. Cases of peak load on the grid are mostly observed in the month of December. Power distribution is done through medium and low voltages of 30 kV in inter-urban centres, 15 kV in urban areas and 400–220 V to residential customers. The littoral region has a critical role in terms of peak demand and accounts for over 46% of the total peak of the SIG. The average transmission losses in the network are 6.3% with 32.03% (Energy of Cameroon (ENEO) Citation2019) for the distribution network giving a total of 38.33%. The power delivered by the grid is insufficient due to rising demand as a result of population growth. A projected extra 595 MW is required to power the industrial and part of the residential sector. The local electricity demand has grown by 6% annually over the past few years (Ministry of Energy and Water Resources (MINEE) Citation2015). Also, droughts and sedimentation reduce the water content in the reservoirs and therefore lessens the quantity of power generated. The inefficient use of electricity in public buildings further adds to the peak demand and causes instability at some sections of the network.
The Southern Interconnected Grid of Cameroon modelled in this study is made up of 82 busbars and 44 transmission lines. The aggregated power demand of the grid was approximately 705.999 MW located on various load buses in the grid. The penetration levels were calculated as percentages of grid-aggregated power demand. The schematic of the SIG is shown in Appendix 6.
2.2. Power flow analysis
Power flow analysis was used in this study. It is a useful tool for grid planning and expansion as it helps in determining the best operating conditions of the network. In power systems, load flow analysis is a numerical study of the flow of electric power in a grid system (Das Citation2006). The main data gotten from a load flow study is the magnitude and phase angle of the voltage at each bus, and the real and reactive power flowing in each line.
The Newton–Raphson (NR) method is famous in providing load flow solutions because of high precision, fast convergence rate and relatively less iterations (Sukumaran et al. Citation2018). The NR method offers a speedy solution void of calculating the superior derivatives for solving system of all sizes and gives efficient results for computational cost minimisation (Khan et al. Citation2019; Kumar et al. Citation2019). Moreover, the results from the NR method are more reliable with a higher success rate of convergence as compared to those from the other power flow algorithms. Therefore, the load flow method adopted in this paper was the NR method. This method starts with initially guessing the unknown quantities in the network such as voltage angle/magnitude at load bus bars and voltage angles at generator buses. The linear system of equations in the analysis is presented below. In order to calculate the net injected power into bus k, the following analysis was performed using a similar approach as in (Das Citation2006).
(1)
(1) where S is the apparent power; Ik is the current injection at bus k; Vk is the voltage magnitude at bus k.
The real power injections at bus k as;
(2)
(2) The reactive power injections at bus k;
(3)
(3) where θki is the admittance angle between lines k and I; δk is the voltage angle at bus k; δi is the voltage angle at bus i.
(4)
(4)
As variables continue to increase, y′ is substituted by the partial derivatives of Pi and Qi with respect to the elements of vector x. The matrix, y′, also called the Jacobian matrix is presented in Equation (5).
(5)
(5) The power residuals which are the difference between scheduled and calculated values at bus i are given by;
(6)
(6)
(7)
(7) The equation for the iteration of the bus voltage magnitudes and angles are;
(8)
(8)
(9)
(9)
(10)
(10) where ΔP is the real power mismatch; ΔQ is the reactive power mismatch; y is the Jacobian matrix representing partial derivatives; Δδ is the change in phase angle; |V| is the voltage magnitude.
The solution of the linearised system of equations is continuously being searched to obtain the next guess (n + 1) of voltage magnitude and angles based on,
(11)
(11)
(12)
(12) The iteration process continues running until when the output of the mismatch equations is less than a specified tolerance value as given in Equation (13).
(13)
(13) where ε = 0.00001.
2.3. Transient stability analysis
Due to the fact that generated power from distributed renewables would displace the generated power from the conventional generators in the network (Sibildas Citation2018), grids having high DG penetration and enormous disturbance could lead to power system instability. The transient analysis is aimed at determining the grid’s dynamic response and stability limits after the modifications (optimum solar PV injection). Usually, power system engineers use the results obtained from this analysis to make critical decisions on what changes are needed in the grid. Some of the grid disturbances include generator outage, short-circuitry or other faults, abrupt change in bulky loads, etc. (Refaat, Abu-Rub, and Mohamed Citation2017; Chang, Chu, and Cauley Citation1995).
In this study, a transient stability analysis was conducted by creating a three-phase fault on the Kribi 225 kV busbar while injecting distributed solar PV at the bus which had the least active power loss (optimum location). In order to generate the swing equation, reference is made to the synchronous generator in . The generators develop an electric torque, Tele, as well the electric power Pele, at the synchronous speed, wsyn. The mechanical power (input power) is Pi.
If the prime mover provides the generator shaft with an input Ti, then at steady state (i.e. no disturbance);
(14)
(14) With the occurrence of a fault, then,
(15)
(15) where Pi is the input power.
Therefore, Equation (14) is not equal to zero and gives rise to an accelerating torque. If Pa is the accelerating (or decelerating) power, then;
(16)
(16) where M is the inertia constant; D is the damping coefficient; θele is the electrical angular position of the rotor.
Since the measurement of the rotor’s angular position is more suitable when taking reference from a synchronously spinning frame.
(17)
(17)
(18)
(18) where δ is the generator’s power angle.
Ignoring the damping coefficient (i.e. D = 0) and substituting Equation (18) in (16), it gives;
(19)
(19) Equation (19) presents the dynamics of the rotor in a synchronous generator and it is called the swing equation.
2.4 General scenario setting and system constraints
The overall active power loss is the main objective function in this study where the impact of solar PV integration into the SIG was measured. The benchmark is the percentage injection and bus location with the lowest active power loss. The objective function is subject to the voltage limits, current limits and power flow constraints. The active power losses in the SIG are the sum of all the active power losses in the lines as presented in the equation below.
where Poverall_loss is the overall grid active power loss; n is the total number of transmission lines in the grid; Pn is the active power loss at bus n.
For the active power loss of the nth transmission line, the equation is given by;
The voltage profiles of bus bars are used in the determination of candidate bus bars in which the distributed energy systems will be injected. Bus bars having a voltage magnitude not falling within the range of allowed values are selected as candidate bus bars which will be used for solar PV injection. A similar approach was used by Abujubbeh and Fahrioglu (Citation2019). High solar PV penetration causes severe voltage issues such as voltage flickers, voltage sags/swells and most commonly overvoltages at feeder terminals (Iweh et al. Citation2021). Various regional blocks and countries have fixed voltage standards in order to manage the range of voltage fluctuation during grid operation such as +3% in Germany, ±10% in Europe, ±5% in America, −6%/+10% in Australia and ±6% in Canada (Hoke et al. Citation2013). The voltage limits used in this study were ±5%. The voltage constraint is presented below;
where Vmin is the minimum voltage of bus k; Vmax is the maximum voltage of bus k.
The ampacity of transformers and transmission lines creates a limit on the amount of solar PV that can be integrated into the grid. Various regional blocks and countries have set their overload restrictions, on nominal current of transformers and transmission lines, to integrate solar PV into the grid such as 85%, 100%, 150% and 187.5% (Iweh et al. Citation2021; Hoke et al. Citation2013). This study used a nominal value of 100% as thermal limits and the inequality is presented below;
where Ik,k + 1 is the current flowing through the line linking bus k to bus k + 1; Ik,k + 1,max is the maximum tolerable current flowing through the line linking bus k to bus k + 1 (thermal limits).
The power balance constraint is given by the equation;
where PGrid is the total generated active power in the grid; PPV is the total power generated by the solar PV; PDemand is the active power demand in the network; Ploss is the total active power losses in the network.
2.5. Calculation of the penetration level
The level of solar PV penetration was evaluated as the ratio of generated active power of the solar PV to the total active power generated by the grid (Hoke et al. Citation2013) as shown in Equation (20).
(20)
(20) where Plevel is the penetration level; PSolar is the injected solar PV power; Pgrid is the grid’s generated active power; Plevel of 0% signifies that the system loads are completely supplied by the grid while a 100% Plevel means the total system loads are fully powered by the solar PV.
3. Results and discussion
The determination of optimal solutions within the network amidst the several obstacles requires the conception of numerous possible simulation scenarios and the application of the suitable solution to the physical system. Here, the SIG was represented in a single-line diagram using ETAP. Load flow study was conducted using the Newton–Raphson’s method while the grid parameters such as bus voltage profile, equipment loading and power losses were monitored. It should be noted that both the physical and electrical inputs of the grid such as tower height, transmission line spacing, line length, line resistance and reactance, capacitor ratings, generator ratings, and transformer rating were considered in the model. This helps to give a representative model of the grid under consideration.
3.1. Base case scenario
This is a scenario where there was no connected solar PV to the grid and its aim was to help in the identification of the candidate busbars for subsequent solar PV injection. The power flow was simulated without the solar PV and the overall real and reactive power demand, real and reactive power losses and the voltage profiles were noted. The total real and reactive power losses after this scenario were 38.974 MW and 87.584 Mvar. The total real power demand of the loads was 705.999 MW while the total reactive power demand by the loads was −9.066 Mvar. The initial simulation of the SIG where components in red represent violations in operating limits is shown in Appendix 7. shows the components and the base case results.
Table 1. Result for base case scenario.
It was observed that the SIG before solar PV integration had a total active power loss of 38.974 MW in the network. Moreover, there were eight under-voltage busbars with voltage magnitudes falling out of the set range. The busbars were categorised from the most affected buses having a high deviation to the least affected buses having the lowest deviation. The busbar with the highest deviation was the Ngousso 93 kV busbar with a percentage deviation of 2.46%. From the results obtained from the base case, shows the candidate busbars for the solar PV injection. The busbars selected for solar PV injection are load busbars with the worst voltage profile (voltage variation out of the range specified) or busbars connected to an overloaded line.
Table 2. Candidate busbars for the solar PV penetration.
3.2. The active power losses due to PV penetration level
The PV system was connected separately to all the candidate busbars and injections were made at intervals of 10% penetration level. The active power losses for all the scenarios were plotted in order to determine the busbar and solar PV penetration with the least active power loss. As the solar PV injection initially rises, the circulation of line currents will reduce as well as the current produced by conventional generators. However, though the increase in solar PV will limit the current generated from conventional generators, the active power losses will increase at a certain injection percentage as the generated current from the solar PV starts circulating in the grid. After plotting all the scenarios, it was observed that the least active power loss was obtained at the Ngousso 93 kV busbar (optimum location). The active power loss obtained at this busbar was 26.234 MW at a 30% solar PV penetration level as seen in . shows the total active power loss at various solar PV penetration levels. After the identification of the busbar with the least active power loss, there is a need to analyse the transient stability of the network under this condition. This is done in the next section.
3.3. Transient stability analysis and the assessment of the optimum solar PV capacity
From the combination of active power losses in , it shows that the Ngousso 93 kV and the Oyomabang 93 kV busbars are potentially the best candidate busbars to optimally place PV due to their observably low overall active power loss reduction after PV penetration. Although the Ngousso 93 kV and the Oyomabang 93 kV busbars are the best connection points for PV injection without any violations, there will be challenges such as transient stability limitation that needs to be investigated in order to ascertain the ability of the grid to accommodate the injected PV power. In order to carry out the investigation, a three-phase fault was generated at the Kribi 225 kV busbar, which is a severe fault scenario. The Kribi generators 1, 2, 3, 4 and 5 have a lower inertia than the Songloulou slack bus. Consequently, the response of the Kribi generators to the fault will be faster than the Songloulou slack bus. The transient stability of the grid was examined by creating the fault for 0.1 s (i.e. between 2 and 2.1 s). The reaction of the rotor angle for the Kribi generators at the optimum location was analysed for stability. According to , the least active power losses (26.234 MW) were obtained at a PV penetration of 30% (211.8 MW) when the connection point was Ngousso 93 kV busbar and therefore, 30% was considered for the analysis. After the three-phase fault, it was observed that the rotor angle of all the five generators connected to the Kribi 255 kV bus had a value of 82.76 degrees before the fault occurred. At 2 s (time of fault occurrence), the oscillation amplitude went as high as 176.79° before slowly being stabilised after 4 s. show the rotor angles of Kribi Gen. 1, Kribi Gen. 2, Kribi Gen. 3, Kribi Gen. 4 and Kribi Gen. 5, respectively, against a time duration of 10 s. The curves show that the generator rotor angles have been disturbed as a result of the fault, suffering a rotor angle swing. After fault clearing, there were oscillations in the rotor angles before gradually being damped to stability afterwards. The disturbance curves decayed with time (t).
3.3.1 Bus voltage stability at 30% solar PV integration
The integration of solar PV into the grid could potentially cause variation in the grid’s steady-state bus voltages. The major cause of these obnoxious voltage profiles is the grid’s inability to meet the reactive power needs of the network (Kundur Citation1994). Even though voltage instability is a localised challenge, its impacts can be extensive. The set limit for voltage in this study was between a range of 95% and 105%. The solar PV power is used in grid stability analyses to assess how solar power interacts with the power system and the extent to which they affect bus voltage stability. Consequently, it is essential to perform analysis which could assist engineers in planning grids with high penetration of solar PV. To perform the analysis, a three-phase fault was created at the Kribi 93 kV busbar at a time of 2 s, under 30% PV penetration at the Ngousso 93 kV busbar. It was observed that the pre-fault voltage of the Bekoko 93 kV busbar had a percentage of 100.34%. After the fault (above 2 s), the percentage bus voltage oscillated within a range of 105.74 and 41.43%, before gradually stabilising as from 4 s. The grid was able to self-restore its bus voltages after the occurrence of the three-phase fault. illustrates the voltage response of the grid to a three-phase fault under 30% PV penetration.
3.3.2 Frequency response at 30% PV penetration at the Ngousso 93 kV busbar
In general, the frequency response of a grid consists of diverse time-based responses like inertial response, primary frequency response, secondary frequency response and tertiary frequency response. The inertial response has the capability of resolving the initial frequency deviation in the generator after a fault before the governor of the synchronous generator could intervene. The interconnection of renewables in the grid spatially causes the displacement of conventional power plants, leading to falling system rotating mass and hence, creates grid instability issues, especially at high renewable penetration. Therefore, the injection of solar PV into the SIG demands that the frequency stability of the network be investigated. The Cameroonian grid operates within a narrow frequency range of 49.90–50.05 Hz, with expectations of further being narrowed down to a range of 49.95–50.05 Hz as the country transitions towards economic emergence in the future. In order to analyse the frequency stability of the system under 30 PV penetration at the Ngousso 93 kV busbar, a three-phase fault was created at the Kribi 225 kV busbar. The fault started at 2 s and was cleared at 2.1 s. It was observed that the frequency of all five generators connected to the Kribi 225 kV busbar had a pre-fault stable frequency of 50 Hz. At 2 s (time of fault occurrence), the frequency flipped between 53.31 and 47.42 Hz before gradually attaining stability as from the fifth second. shows the frequency response of the Bekoko 93 kV and the Ngousso 93 busbars.
3.3.3. Response of rotor angle at 50% solar PV injection
In order to assess how excess PV penetration could affect the transient response of the grid, 50% PV penetration was injected on the Ngousso 93 kV busbar and a three-phase fault was created at the Kribi 225 kV busbar. The fault was created at 2 s and was eventually cleared at 2.1 s. The transient response of the generators connected to the Kribi 225 kV busbar was studied. It was observed that the pre-fault rotor angle (i.e. before 2 s) was stable and became uncontrollable even after the fault was cleared. This is a serious problem in the grid as the generator output is not synchronised and would gradually lead to a grid collapse if the situation surpasses the critical fault time. The results are shown in Appendix 1–5.
4. Conclusion
The study has used the ETAP software to model successive solar PV injections into the Southern Interconnected Grid (SIG) of Cameroon in order to determine the solar PV hosting capacity of the grid. A novel approach has been proposed to determine the PV hosting capacity while taking into consideration power system disturbance and recovery. Active power loss and transient stability analysis were used to identify the optimum busbar that was capable of hosting the maximum permissible solar PV in the SIG. The maximum hosting capacity determined from the ETAP model was 30% at the Ngousso 93 kV bus with an active power loss of 26.234 MW. The results obtained after transient stability at this busbar showed no stability issues. The integration of solar PV on suitable locations in the grid would reduce the power losses in the network. Nevertheless, the extent of power loss reduction varies with the injection busbar. When injected on a suitable load bus, the solar PV impact on the power losses will decrease as penetration level increases. This shows that the solar PV penetration level is inversely proportional to the power losses only at a certain penetration, beyond which their relationship becomes that of direct proportionality. Hence, there is an optimum DG penetration level beyond which, increase in the solar PV penetration level will increase power losses in the grid. However, there were equally extreme cases, such as the Limbe 93 kV busbar, where power losses immediately started rising as the solar PV penetration level increased. This is a clear indication of a wrong choice of injection location.
The solar PV market is rising at a geometric rate. Cameroon’s policy and regulatory framework have been increasingly encouraging the development of renewables especially solar PV. Therefore, solar PV penetration into Cameroon’s grid is anticipated to be a reality with the current hybridisation of some thermal plants with solar PV systems by the utility company. This study is a timely endeavour as the country is already committed to sustainable development.
RE deployment is not only centred on electricity generation but also involves the optimisation of all components in the grid network. Consequently, to have a successful solar PV integration requires an all-inclusive approach combining policy, technology and regulation. Cameroon’s RE penetration level needs to boom if she must achieve the much anticipated ‘vision 2035’. Also, an increase in RE penetration levels would be problematic without appropriate policy, technology and regulation to fully carter for it. The sustainable supply of electricity in Cameroon would be achieved through a collective effort involving government, utility companies and solar project developers. Finally, electricity consumers equally have a significant role in this goal either through paying electricity bills or contributing in electricity generation as prosumers (producers and consumers).(Intergovernmental Panel on Climate Change (IPCC) Citation2022)
Acknowledgements
The authors acknowledge the support from the World Bank through the African Centers of Excellence (ACE) – Impact Project with the Regional Center for Energy and Environmental Sustainability (RCEES), University of Energy and Natural Resources, Sunyani, Ghana, the West African Research Association (WARA) and other anonymous contributors for their input. The authors are equally grateful to ENEO staff for cooperatively sharing information in this research.
Disclosure statement
No potential conflict of interest was reported by the author(s).
References
- Abubakar, S., X. Yonghai, A. Waseem, H. Muhammad, A. Adnan, and M.M. Hafiz. 2019. “Load Flow Analysis of 132/11 KV Grid Station Bahawalpur Region Pakistan and its Voltage Improvement Through FACTS Devices Using ETAP.” 2019 IEEE PES Innovative Smart Grid Technologies Asia.
- Abujubbeh, M., and M. Fahrioglu. 2019. “Determining Maximum Allowable PV Penetration Level in Transmission Networks: Case Analysis – Northern Cyprus Power.” 2019 1st Global Power, Energy and Communication Conference (IEEE GPECOM2019), Cappadocia, Turkey.
- Airoboman, A.E., P. James, I.A. Araga, C.L. Wamdeo, and I.K. Okakwu. 2019. “Contingency Analysis on the Nigerian Power Systems Network.” 2019 IEEE PES/IAS PowerAfrica.
- Al-Refai, S.M. 2016. Design of a Large Scale Solar PV System and Impact Analysis of Its Integration into Libyan Power Grid. Nicosia: near east university.
- Aman, M.M., G.B. Jasmon, A.H.A. Bakar, and H. Mokhlis. 2013. “A new Approach for Optimum DG Placement and Sizing Based on Voltage Stability Maximization and Minimization of Power Losses.” Energy Conversion and Management 70: 202–210. doi:10.1016/j.enconman.2013.02.015.
- Andersson, G. 2008. “Modelling and Analysis of Electrical Power Systems,” Zurich.
- Asano, H., K. Yajima, and Y. Kaya. 1996. “Influence of Photovoltaic Power Generation on Required Capacity for Load Frequency Control.” IEEE Transactions on Energy Conversion 11: 188–193. doi:10.1109/60.486595.
- Baker, P.P. 2000. “Determining the Impact of Distributed Generation on Power Systems – Part 1: Radial Distribution Systems.” Proceedings of IEEE PES Summer Meeting, Seattle.
- Bamdad, E. 2014. High Penetration Photovoltaic System Analysis. Northridge: California State University.
- Barsoum, N., and C.Z. Lee. 2013. “Simulation of Power Flow and Protection of a Limited Bus Grid System with Injected Solar Power.” Energy and Power Engineering 5: 59–69. doi:10.4236/epe.2013.51008.
- Bayer, B., and A. Marian. 2020. “Innovative Measures for Integrating Renewable Energy in the German Medium-Voltage Grids.” Energy Reports 6: 336–342. doi:10.1016/j.egyr.2019.12.028.
- Bista, S. 2019. Supply Adequacy Assessment of an Islanded Micro-Grid. Perth, WA: Murdoch University.
- Chang, H.D., C.C. Chu, and G. Cauley. 1995. “Direct Stability Analysis of Electric Power Systems Using Energy Functions: Theory, Applications, and Perspective.” Proceedings of the IEEE 83 (11): 1497–1529. doi:10.1109/5.481632.
- Chant, T.I., G.M. Shafiullah, A.M.T. Oo, and B. Harvey. 2011. “Impacts of Increased Photovoltaic Panel Utilization on Utility Grid Operations – A Case Study for Central Queensland.” Innovative Smart Grid Technologies Conference (ISGT 2011), Perth, Australia.
- Das, D. 2006. Electrical Power Systems. New Delhi: New Age International Publishers.
- Energy of Cameroon (ENEO). 2019. “2019 Annual Report: Eneo is Transforming the Electricity Service,” ENEO, Douala, Cameroon.
- Energy of Cameroon (ENEO). 2020. “ENEO 2020 Annual Report,” ENEO, Douala, Cameroon.
- Hoke, A., R. Butler, J. Hambrick, and B. Kroposki. 2013. “Steady-State Analysis of Maximum Photovoltaic Penetration Levels on Typical Distribution Feeders.” IEEE Transactions on Sustainable Energy 4 (2): 350–357. doi:10.1109/TSTE.2012.2225115.
- Intergovernmental Panel on Climate Change (IPCC). 2022. “IPCC Guidelines for National Greenhouse Gas Inventories – Task Force on National Greenhouse Gas Inventories,” IPCC, [Online]. Accessed 10 August 2022. https://www.ipcc-nggip.iges.or.jp/public/2006gl/index.html.
- International Energy Agency/Organisation for Economic Co-operation and Development (IEA/OECD). 2014. “The Power of Transformation: Wind, Sun and the Economics of flexible power systems,” IEA/OECD, Paris, France.
- Ismael, S.M., A.S.H.E. Abdel, A.Y. Abdelaziz, and A.F. Zobaa. 2019. “State-of-the-Art of Hosting Capacity in Modern Power Systems with Distributed Generation.” Renewable Energy 130: 1002–1020. doi:10.1016/j.renene.2018.07.008.
- Iweh, C.D., S. Gyamfi, E. Tanyi, and E. Effah-Donyina. 2021. “Distributed Generation and Renewable Energy Integration Into the Grid: Prerequisites, Push Factors, Practical Options, Issues and Merits.” Energies 14 (5375): 1–34. doi:10.3390/en14175375.
- Khan, S., S.U. Rehman, A.U. Rehman, and H. Khan. 2019. “Optimal Placement of Distributed Generation in Power System for Power System Loss Reduction Using ETAP.” International Journal of Engineering and Technologies 16: 7–19. doi:10.56431/p-qpo00i.
- Kumar, S.A., M.S.S. Narayana, B.A. Naidu, and G.V.S. Reddy. 2019. “Optimal Power Flow for IEEE – 9 bus System Using ETAP.” International Journal of Recent Technology and Engineering 6: 368–370.
- Kundur, P. 1994. Power System Stability and Control. New York: McGraw-Hill Inc.
- Kundur, P., J. Paserba, V. Ajjarapu, G. Anderson, A. Bose, C. Canizares, N. Hatziargyriou, et al. 2004. “Definition and Classification of Power System Stability IEEE/CIGRE Joint Task Force on Stability Terms and Definitions.” IEEE Transactions on Power Systems 19 (3): 1387–1401. doi:10.1109/TPWRS.2004.825981.
- Latheef, A.A., D.A. Robinson, V.I. Gosbell, and V. Smith. 2006. “Harmonic Impact of Photovoltaic Inverters on Low Voltage Distribution Systems.” 12th International Conference on Harmonics and Quality of Power, Lisbon, Portugal.
- Luhmann, T., T.E. Wieben, R. Treydel, M. Stadler, and T. Kumm. 2015. “An Approach for Cost-Efficient Grid Integration of Distributed Renewable Energy Sources.” Engineering Sciences Press 1 (4): 447–452. doi:10.15302/J-ENG-2015099.
- Lund, P.D., J. Lindgren, J. Mikkola, and J. Salpakari. 2015. “Review of Energy System Flexibility Measures to Enable High Levels of Variable Renewable Electricity.” Renewable and Sustainable Energy Reviews 45: 785–807. doi:10.1016/j.rser.2015.01.057.
- Lynch, MÁ, R.S.J. Tol, and M.J. O’Malley. 2012. “Optimal Interconnection and Renewable Targets for North-West Europe.” Energy Policy 51: 605–617. doi:10.1016/j.enpol.2012.09.002.
- Ministry of Energy and Water Resources (MINEE). 2015. “Situation énergétiques du Cameroun,” Ministère de l’Eau et de l’Energie (MINEE), Yaounde, Cameroon.
- Moh, Z.F., I. Sujito, and W. Made. 2020. “Contingency Analysis on 150 kV Electricity Power System on the Madura Island Based on the 1P1Q Method.” Atlantis Highlights in Engineering 7: 202–207.
- Mosbah, F. 2018. Design and Analysis of a Hybrid Power System for Western Libya. Newfoundland: Memorial University of Newfoundland.
- Mujtaba, G., Z. Rashid, F. Umer, S.K. Baloch, G.A. Hussain, and M.U. Haider. 2020. “Implementation of Distributed Generation with Solar Plants in a 132 kV Grid Station at Layyah Using ETAP.” International Journal of Photoenergy - Hindawi, 1–8. doi:10.1155/2020/6574659.
- Muntathir, A.T., and A.B. Chokri. 2020. “Optimal PV Penetration for Power Losses Subject to Transient Stability and Harmonics.” Procedia Computer Science 175: 508–516. doi:10.1016/j.procs.2020.07.072.
- Rajaram, R., K. Sathish Kumar, and N. Rajasekar. 2015. “Power System Reconfiguration in a Radial Distribution Network for Reducing Losses and to Improve Voltage Profile Using Modified Plant Growth Simulation Algorithm with Distributed Generation (DG).” Energy Reports 1: 116–122. doi:10.1016/j.egyr.2015.03.002.
- Refaat, S.S., H. Abu-Rub, and A. Mohamed. 2017. “Transient Analysis and Simulation of a Grid-Integrated Large-Scale Photovoltaic (PV) Energy System.” QScience Connect 8: 1–10. doi:10.5339/connect.2017.qgbc.8.
- Rodriguez, C., and A.J. Amaratunga. 2004. “Dynamic Stability of Grid Connected Photovoltaic Systems.” IEEE Power and Energy Society General Meeting.
- Sajid, M., K. Iftikhar, and A.U. Rehman. 2019. “Analysis to Integrate Microhydro Power Plant as Distributed Generation with 11 kV Utility Feeder in Dow Bandow, Upper Dir, Pakistan Using ETAP.” International Journal of Scientific & Engineering Research 10 (4): 146–151.
- Shafiullah, G.M., M.T.O. Amanullah, A. Stojcevski, and A.A.B.M. Shawkat. 2014. “Integration of Roof-top Solar Photovoltaic Systems Into the low Voltage Distribution Network.” Journal of Renewable & Sustainable Energy 6. doi:10.1063/1.4884904.
- Shrestha, B., B. Paudyal, N.R. Karki, and N.P. Gyawali. 2017. “Steady State Analysis of PV Integration in Hydro Dominated INPS Grid Using ETAP.” 7th International Conference on Power Systems (ICPS), Pune, India.
- Sibildas, M. 2018. “Distributed Generation and its Impact on the Grid”.
- Sucita, T., and Y. Mulyadi. 2017. “Contingency Analysis of Electric Power Systems Sub-System 150 KV West Java Area II.” 1st Annual Applied Science and Engineering Conference.
- Sukumaran, S., I. Vidya, M.D. Sangeetha, and K. Renu-priya. 2018. “Optimal Power Flow Analysis for 23MW Microgrid Using ETAP.” International Journal of Innovative Science and Research Technology 3 (3): 570–575.