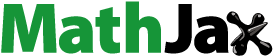
ABSTRACT
Biodiesel is considered environmentally benign, biodegradable, and greener fuel derived from vegetable oils, animal fats, and algae, superseding petroleum products. Hybridisation of vegetable oil has not been widely examined to produce biodiesel with ameliorated quality. This study aims at hybridising Azadirachta indica and Moringa stenopetala seed oils to produce the hybrid biodiesel with improved quality. The Box-Behnken Design was used in design of the experiment and analysis of results. Catalyst dose, oil hybridisation ratio, and reaction time were experimental variables with 3 levels, whereas, agitation speed, oil-to-methanol molar ratio, and reaction temperature were constants. The oil hybridisation ratios were: 25% A. indica and 75% M. stenopetala oil, 50% A. indica and 50% M. stenopetala oil, and 75% A. indica and 25% M. stenopetala oil. Seventeen experimental runs were conducted and the quadratic model was developed at P < 0.0001(significant). The model was determined by analysis of variance (ANOVA) and determination coefficients at 5% LSD.
1. Introduction
Energy is a fundamental, vital ingredient, and the backbone for economic growth, social and environmental welfare as well and it plays a great role in achieving the civilisation of mankind worldwide. The existence of mankind, global civilisation, and socio-economic growth necessitated the sustainable production and utilisation of energy (Caetano et al. Citation2017; Singh, Nyuur, and Richmond Citation2019; Güney and Kantar Citation2020). The extraction and utilisation of fossil fuels (i.e. coal, natural gas, liquefied petroleum gas, and nuclear energy) have been regarded as the main source of energy supply for several years (Oliveira and Moutinho Citation2021; Mizik and Gyarmati Citation2021). Nonetheless, when used, fossil fuels are environmentally unfriendly (harmful gaseous emissions into the atmosphere), non-renewable, scarce (limited crude oil reserves), and unevenly distributed across the globe (Zou, Zhao, and Zhang Citation2016; Holechek and Geli Citation2022; Wang et al. Citation2021). Furthermore, the rise in energy demand, the geometric increase in global population, the markedly decreasing sources of non-renewable energy, environmental pollution, greenhouse gas emissions, and gas flaring, fluctuations in fuel prices, the hastened global warming, and the global energy crisis are challenges associated with the use of fossil fuels (Peterson and Ercan Citation2023; Lola, Aleh, and Jessica Citation2022; Bekirogullari, Fragkopoulos, and Pittman Citation2017). To overcome these challenges, the world is strongly looking forward to sustainable production and utilisation of biofuels thereby superseding non-renewable petroleum products (Motevali et al. Citation2023; Ozturk Citation2014; Mamoona et al. Citation2023).
Biodiesel has been identified as a suitable fuel that is a greener, eco-friendly, cleaner, renewable, and alternative fuel that can replace the non-renewable and limited oil reserves (Oliveira and Moutinho Citation2021; Degnechew, Citation2021a; Brahma et al. Citation2022). It is a liquid biofuel derived and synthesised from biological resources like non-edible vegetable oils, algae, and animal fats through the transesterification process (Neupane Citation2023; Azadbakht, Ardebili, and Rahmani Citation2023). It is a fatty acids mono-alkyl ester mixture produced from potential feedstocks like triglycerides of the non-edible vegetable oils undergoing the transesterification reaction by employing appropriate alcohol and a basic-catalyst providing glycerol (C3H8O3), a byproduct of the reaction process (Okechukwu et al. Citation2022; Domínguez et al. Citation2019; American Society for Testing Materials [ASTM] D6751-Citation23 Citation2023). Biodiesel synthesis through hybridisation of feedstocks has not been extensively studied all over the globe. The process of hybridisation in the transesterification reaction involves a novel combination or mixing of two feedstocks (bi-hybridisation), three feedstocks (tri-hybridisation), or many feedstocks (poly-hybridisation) at various ratios to synthesise a new product with improved attributes (i.e., the hybrid biodiesel) derived from the original feedstocks (Brahma et al. Citation2022; Andrew, Eboka, and Inambao Citation2016). Thus, blending of feedstocks before the transesterification process (i.e. in situ hybridisation) improves the quality of the resulting fuel. This approach is new and hasn’t been tried extensively in previous work and paves the way for biofuel processing industries to produce and develop biodiesel products from various feedstocks. Hybridising feedstocks like Azadirachta indica and Moringa stenopetala seed oils to synthesise biodiesel will enhance fuel stability and engine performance (fuel combustion and compression ignition) during utilisation (Kumar, Babu, and Kumar Citation2018; Zhang et al. Citation2021). Moreover, hybridisation of appropriate feedstocks results in improved and ameliorated biodiesel quality that is better than the one synthesised from a mono-feedstock (Andrew, Eboka, and Inambao Citation2016). Vegetable oil that constituents sufficient mono-unsaturated fatty acids alongside the fatty acid profiles of oil (a higher percentage of oleic acid) is considered a suitable ingredient to obtain biodiesel exhibiting good fuel quality (Kumar, Babu, and Kumar Citation2018; Adepoju and Olawale Citation2015; Andinet et al. Citation2010). For instance, the crude oil obtained from Azadirachta indica seeds constituents about 51% of oleic acid (C18H32O2) (Adepoju and Olawale Citation2015); and this will be improved and ameliorated upon blending with the higher oleic acid content of 76% C18H32O2 in the oil extracted from Moringa stenopetala seeds (Andinet et al. Citation2010). In light of this explanation, the experiment was conducted to examine the effectiveness and suitability of the hybridised non-edible oils of A. indica and M. stenopetala seeds, thereby synthesizing the improved biodiesel through the transesterification reaction as a new approach to product design, processing, developing, and utilising the biofuel.
2. Materials and methods
2.1. Sample collection and preparation
The Azadirachta indica seeds were collected in January 2023 from Amibara, Afar Regional State, in the eastern part of Ethiopia. The sample collection area has a latitude of 09°15′ North, a longitude of 40°10′ East, and an altitude of 740 m a.s.l. The Moringa stenopetala seeds were collected in January 2023 from Sidama Regional State, south part of Ethiopia, situated at the latitude of 7°4′ north, longitude of 38°24′ east, and an altitude of 1,712 m a.s.l. The seeds were taken to the Laboratory of Addis Ababa Institute of Technology. Then, the collected seeds were cleaned of unwanted substances like stones, weed seeds, molds, other potential contaminants, and the seeds were decorticated (i.e. the seed kernels were separated from the husk). The seed kernels were then subjected to drying at about 100 ± 5°C for twenty-four hours using the oven dryer until the moisture in the seed kernels was below five percent (Adejumo and Abayomi Citation2012). Finally, the amount of moisture content in the seed kernels was computed as follows:
(1)
(1) W0 – sample weight (g) before drying; W1 – sample weight (g) after drying
For the ease of oil extraction process, the dried seed kernels were subjected to comminution using a hammer mill at various ranges of particle sizes, 0.2–0.4 mm, 0.4–0.6 mm and 0.6–0.8 mm thereby enhancing the surface area-to-volume ratio of the particles. The vibrating sieve shaker was used to obtain the required range of particle size and then the powder of each sample was labelled and kept appropriately until dispatch.
2.2. Extraction and purification of oil for biodiesel synthesis
In the oil extraction process, the Soxhlet extractor was used with the extraction solvent n-Hexane (n-C6H14). A 150 g sample powder was put into the extraction thimble and placed in the Soxhlet. A 750 mL n-C6H14 was added into a flask of 1000 mL and attached to the Soxhlet apparatus. The reflux condenser was also attached to the upper part of the Soxhlet, and the complete extraction set-up was adjusted and placed in the boiler. The temperature of the boiler was set at 74 °C, which is relatively higher than the boiling point of n-Hexane, and oil extraction was allowed to take place continuously for about four hours until the desired amount of crude oil was obtained. As heating continues, the temperature of extraction rises gradually, and the solvent is vaporised and refluxed to the sample in the thimble by the condenser. Then, the rotary evaporator was employed to separate the mixture of crude oil and solvent (n-hexane). The yield of oil (%) at every extraction step was computed as follows (Xiao et al. Citation2022; Kabutey, Herák, and Mizera Citation2022):
(2)
(2) The obtained oil was subjected to the oil purification process by employing the centrifuge to remove unnecessary substances like suspended fine particles. The trace solvent or n-hexane was also liberated and removed from the crude oil by heating and boiling the oil. Then, before to the transesterification reaction, the crude oils were subjected to the oil degumming, esterification reaction (acid pretreatment), and neutralisation processes for further purification and refining of the extracted oil.
In the oil degumming process, phospholipids and gums were removed from A. indica and M. stenopetala oils. A 2%v/v (distilled water/oil) was poured into extracted oils and stirred for five minutes in a hot water bath at 65 °C. Then, a 1.3%v/v (phosphoric acid/oil) was added to the oil and distilled water mixture and stirred (i.e. at 200 revolutions per minute) in the hot water bath for 1 h. The degummed oils were esterified to diminish the amount of free fatty acid (FFA) content. In the esterification reaction, oils were heated for ten minutes at 60 °C in a hot water bath. Then, 45%v/v methanol was poured into esterified oil and stirred for five minutes. A 1.2%v/v sulfuric acid was added to the oil mixture and stirred in the hot water bath for an additional 45 min. The mixture was added into a separating funnel and allowed to settle down for four hours. The refined oil was obtained from the bottom portion of the mixture in the funnel and stored in an air-tight waterproof container. The refined oil was neutralised by adding 0.1 N sodium hydroxide solution into the esterified oil, heated at 60 °C, and stirred at 250 rpm for about thirty minutes. Finally, the neutralised oil mixture was allowed to settle down using the separating funnel thereby obtaining the neutralised oils for the synthesis of the biodiesel (Degnechew Citation2021b).
2.3. Biodiesel synthesis via base-catalyzed transesterification reaction
2.3.1. Experimental design
The Response Surface Methodology (RSM), Box-Behnken Design (BBD), was employed in the design of the experiment. The operational variables in the study were: oil hybridisation ratio (A), reaction time (B), and catalyst dose (C), with three experimental levels for each variable, low (−1), medium (0), and high (+1) and the yield of biodiesel (%) was the response of the experiment (). The influence of operational variables on the response was examined along with their interaction effects. A total of seventeen experimental runs were conducted (i.e., three factors with three levels and five central replication points) following the BBD method (Aslan and Cebeci Citation2007):
(3)
(3) N – total experimental runs; K – number of operational variables; and Cp – central replication point
Table 1. Levels of experiment for the considered factors of study.
The refined crude oils of Azadirachta indica (A) and Moringa stenopetala (M) seeds were obtained in various proportions and allowed to undergo the transesterification process at different operational variables, such as oil hybridisation ratio, reaction time, and catalyst dose. The considered reaction times (in minutes) were: 30, 60, and 90 min; the catalyst doses were: 0.50, 2.00, and 3.50%w/v; and the oil hybridisation ratios (%w/w) were: A25M75 (25% A. indica seed oil hybridised with 75% M. stenopetala seed oil), A50M50 (50% A. indica seed oil hybridised with 50% M. stenopetala seed oil), and A75M25 (75% A. indica seed oil hybridised with 25% M. stenopetala seed oil), with their respective yields of biodiesel (BAM) products: BA25M75, BA50M50, and BA75M25. Besides, the other operational variables like agitation speed (rpm), oil-to-methanol molar ratio, and reaction temperature (°C) were allowed to be constants during experimentation ().
Table 2. The influence of factors of study on yield (%) of synthesised hybrid biodiesel from hybridised oils of A. indica and M.stenopetala
seeds.
2.3.2. Experimental procedure for the transesterification process
The refined oils of A. indica and M. stenopetala were hybridised and trans-esterified into methyl ester by applying the appropriate basic catalyst, sodium hydroxide (NaOH) flakes, and methanol (CH3OH). A three-necked round-bottom glass reactor was employed to conduct the base-catalyzed transesterification reaction. A 0.50, 2.50, and 3.50%w/v sodium-methoxide (CH₃ONa) solution were used in the transesterification reaction (). The weighed 100 g hybridised oil was added into the glass reactor. Then, the prepared basic catalyst, CH₃ONa solution, was poured turn-by-turn into the glass reactor containing a sample of the hybridised oils (%w/w) at a 1:6 oil-to-methanol molar ratio. The mixture was allowed to undergo the transesterification reaction at 60 °C and an agitation speed of 300 revolutions per minute (rpm) for 30, 60, and 90 min, respectively. To quench the reaction from occurring further (i.e. beyond the prescribed reaction time), about three droplets of hydrochloric acid were mixed with the solution in the glass reactor. This procedure was repeated for all experimental runs, and their corresponding biodiesel was obtained as per the information provided below (). The trans-esterified solution was transferred into a funnel and kept for 24 h to remove glycerol. Then, the obtained hybrid biodiesel was washed four times at a 3:1 biodiesel-to-distilled water ratio and heated at 60 °C for further purification of the fatty acid methyl ester (FAME) from traces of NaOH and other impurities. Then, the purified FAME was heated to 100 ± 5 °C thereby removing traces of washing water and subjected to the evaluation of physicochemical characteristics and Fourier transform infrared radiation (FTIR) spectrometer.
2.4. Evaluation of the physicochemical characteristics of the synthesised FAME
Determination of the hybrid biodiesel physicochemical properties was conducted according to ASTM D6751 and the European Committee for Standardization of Biodiesel (EN 14214). These properties include kinematic viscosity, higher heating value, specific gravity, cetane number, flash point, acid number, free glycerin, total glycerin, cloud point, water and sediment, methanol content, oxidation stability, pour point, carbon residues, magnesium, and calcium contents. Besides, the FTIR spectrometer was used to analyse functional groups of A. indica and M. stenopetala oils, and the hybrid biodiesel synthesized from the hybridised oils. The FTIR analysis was executed for each sample, using 5 ml of oils and biodiesel at a wave number ranging from 4000–400 with 1 cm−1 resolution.
3. Results and discussion
3.1. The statistical analysis of synthesised biodiesel using the RSM
The RSM, Box-Behnken Design, was employed to analyse and interpret the experimental results. The analysis of variance (ANOVA), determination of coefficients of the developed model equation of the synthesised biodiesel from hybridised oils and regression analysis were carried out using the RSM, BBD method. Results of ANOVA were also used to evaluate the adequacy of the developed model equation. The coefficients of determination (R2), predicted coefficient of determination (Pred-R2), coefficient of variations (CV), F- test, and adjusted coefficients of determination (adj-R2) were used to explain the model quality and its statistical significance, provided that the main comparisons were carried out at 5% of the least significant difference (LSD).
The actual yield of biodiesel synthesised from each and every single experimental run () was computed as follows (Xuejun, He, and Zhu Citation2007):
(4)
(4) The obtained results indicated that the maximal biodiesel yield, 92.00%, was recorded for experimental run 7 () at A25M75 (25%w/w Azadirachta indica oil was hybridised with 75%w/w Moringa stenopetala oil), 90 min of reaction time, and 2%w/v of catalyst dose; whereas, the minimal percentage of biodiesel yield, 60.96%, was obtained for the experimental run 4 at A50M50 (50%w/w Azadirachta indica oil was hybridised with 50%w/w Moringa stenopetala oil), 30 min reaction time and 0.5%w/v catalyst dose.
3.1.1. Development and analysis of the model equation for the synthesised FAME
The ANOVA results were used to develop the model equation and analyse its significance and suitability or fitness using the BBD.
Results of ANOVA showed that the model equation is significant with an F-value of 559.30 and a P < 0.0001. This shows only a 0.01% chance that the model F-value is large, and it could happen due to noise. The model terms with a P < 0.05 are considered significant. Thus, the model terms A, B, C, AB, AC, A2, B2, and C2 are significant as the P-value < 0.05, whereas BC is not a significant term of the model as the P-value > 0.05 (). The insignificant terms of the model are not counted and would be diminished to ameliorate the model equation. The F-value of ‘lack of fit’ = 3.80 shows that ‘lack of fit’ is statistically insignificant with a P-value = 0.1150 in comparison to pure error. There is only an 11.50% chance that the ‘lack of fit F-value’ is large, and this could occur because of noise. The model fit summary was evaluated for the obtained experimental results, resulting in the selection of the second-order model equation with statistically significant terms (F-value of 464.53 at P < 0.000), where the model is not aliased ().
Table 3. Analysis of variance for the synthesised hybrid biodiesel at different factors of study.
Table 4. The developed model for the synthesised hybrid biodiesel at various experimental variables.
Moreover, the prediction coefficients (Pred-R2 of 0.9830) were in agreement with adjusted coefficients of determination (Adj-R2 of 0.9968). The value of ‘adequate precision’ (80.284) measures the signal-to-noise ratio and the model desirability, and hence, the ratio of 80.284 shows an adequate signal for the model as it was greater than four (); and the equation can be employed to navigate the design space for the production biodiesel at various experimental factors.
Table 5. The developed model adequacy measures for the synthesised hybrid biodiesel.
The obtained value of R2 (0.9986) shows that about 99.86% of the overall variations that occurred in the experimental responses (yield of FAME) were associated with the factors considered in the study. Besides, the minimum value of the coefficient of variations (0.63%), the ratio of the estimated standard error to the average value of biodiesel yield, indicated that the conducted experiment to synthesise biodiesel via the transesterification reaction was precise, reliable, and reproducible. Therefore, the model equation is considered reproducible and it can be used at various experimental variables with different levels to synthesise the hybridised FAME (Aslan and Cebeci Citation2007):
The developed model equation with coded factors to synthesize the hybrid biodiesel according to BBD, Yield of biodiesel (Y):
(5)
(5)
3.1.2. The diagnostics case statistics of the synthesised FAME
To determine the model’s properties and the nature of its statistical distribution, the plot of normal probability versus residual error was used following the BBD method. The obtained plot indicated that the normal distribution of residual errors were in linear patterns ((a)). Therefore, for the developed model equation, a set of data points was anticipated to be in a linear pattern with no sign of abnormality involved in the experimental data distributions. In addition, the obtained coefficient of determination, R2 = 0.9986 (a high correlation coefficient) showed that the predicted FAME yields were in agreement with actual experimental results (); and a set of obtained data well fits the model, offering a precise prediction of the desired response or yield of biodiesel for the conducted experiment while considering the range of experimental parameters ((b)).
Figure 1. (a) Normal probability versus residual errors plot (b) Predicted values versus actual experimental values plot.

Table 6. The diagnostics case statistics of synthesizeed hybrid biodiesel.
3.2. The influence of experimental factors on the yield of the FAME
3.2.1. The influence of oil hybridisation ratio and reaction time on the yield of the FAME
The results of the analysis of variance indicated that oil hybridisation or mixing ratio and reaction time significantly affect yield of FAME with ‘P-value = 0.0334’, which is less than 0.05 (). The effect was obtained by keeping the catalyst dose at 2.00%w/v. The maximum biodiesel yield (92%) was obtained when oil hybridisation ratio of A25M75 (mixture of 25%w/w A. indica oil and 75%w/w M. stenopetala oil) reacts with 2.00%w/v of catalyst dose for about 90 min; whereas, the minimum biodiesel yield (73%) was obtained when the oil hybridisation ratio (A25M75) reacted with a 2.00%w/v catalyst dose for 30 min (). The response surface plot showed that increasing the oil hybridisation ratio decreases the yield of biodiesel and increasing the reaction time until an optimum point increases the yield of biodiesel and vice-versa. Nonetheless, a longer reaction time beyond the optimum point decreases the FAME yield as the transesterification reaction is reversible.
3.2.1. The influence of oil hybridisation ratio and catalyst dose on the FAME yield
The ANOVA result signified that the oil hybridisation ratio and catalyst dose affects the yield of biodiesel significantly with ‘P = 0.0129’ (). The reaction time was kept constant at 60 min. The maximum yield of biodiesel (90%) was obtained when the oil hybridisation ratio of A25M75 (mixture of 25%w/w A. indica oil and 75%w/w M. stenopetala oil) reacted with 3.5%w/v catalyst dose for 60 min, whereas, the minimum yield of biodiesel (72.65%) was obtained for the reaction of the oil hybridisation ratio A75M25 (a mixture of 75%w/w of A. indica oil and 25%w/w of M. stenopetala oil) with 0.50%w/v of catalyst dose (). The 3D plot showed that, for experimental factors taken into account, increasing the oil hybridisation ratio diminishes the yield of biodiesel, and as the amount of catalyst rises from 0.50 to 3.50%w/v, the FAME yield (%) will also be enhanced, and vice-versa.
3.2.2. The influence of reaction time and catalyst dose on the FAME yield
The experimental variables (reaction time and catalyst dose) affect the FAME yield significantly at P < 0.0001 (). The oil hybridisation ratio was kept constant at A50M50 (a mixture of 50%w/w A. indica oil and 50%w/w M. stenopetala oil) to observe the effect of the considered variables on the yield of FAME. The 3D plot of BBD signifies that increasing the reaction time and catalyst dose enhances the yield of FAME and vice-versa. The maximum yield of biodiesel (89.74%) was achieved when the transesterification reaction was conducted for 90 min with a 3.50%w/w catalyst dose, and the minimum product yield (60.96%) was recorded when the experiment was conducted with a 0.50%w/w catalyst for about 30 min ().
3.3. Determination of the physicochemical characteristics of the optimised yield of the FAME:
3.3.1. The Fourier transforms infrared radiation (FTIR) spectrometer
The FT-IR spectrometer was used to analyse the functional group of the synthesised biodiesel or FAME, the A. indica, and M. stenopetala seed oils (Oyerinde and Bello Citation2015; Rabelo et al. Citation2015). The determination of FTIR was carried out at 1 cm−1 resolution for the transmission band ranging from 4000 to 400 cm−1 wave number using 5 ml samples of the biodiesel, A. indica, and M. stenopetala seed oils (). The existence of various functional groups in the extracted oils of Azadirachta indica and Moringa stenopetala seeds and the synthesised FAME were analysed by peak points of the infrared radiation spectrum for the specified range of a wave number (Rosset and Perez-Lopez Citation2019). The transesterification reaction induces the transition of triglycerides from precursors (the refined non-edible oils) into their corresponding FAME, which in turn causes the occurrence of variations in the infrared spectrum (Rabelo et al. Citation2015; Jaggi and Vij Citation2006). The wave number between 4000 and 3125 cm−1 showed the presence of a single bond or hydrogen bond (O-H) in the refined oils. This band of the spectrum signifies the presence of moisture or hydrate (H2O) in the refined oils of A. indica and M. stenopetala. Nonetheless, there is no hydrate in the synthesised biodiesel from hybridised oils due to the absence of a peak point in the specified range of the infrared spectrum. In the region of the infrared spectrum from 3125 to 1900 cm−1 wave number, the transmission band of the refined oils was similar to that of the synthesised biodiesel. In addition, the existence of the carboxylic acid functional group in A. indica oil, M. stenopetala oil, and the synthesised fatty acid methyl ester in the transmission band of 3125–1900 cm−1 indicated that the refined oils can be trans-esterified into the alkyl ester (biodiesel) via the transesterification reaction (Rabelo et al. Citation2015; Jaggi and Vij Citation2006). The peak of infrared spectrum in the range of frequencies 1900–10 cm−1 signifies the existence of C-H stretching vibration of the alkene ( = CH2) functional group in the refined oils and biodiesel (Dube et al. Citation2004). Moreover, the region of transmission band between the frequencies of 10–750 cm−1 was considered as a ‘fingerprint’ region, indicating the existence of the C-H bending vibration of an alkane (- CH2 -) functional group in the synthesised fatty acid methyl ester and refined oils of A. indica and M. stenopetala seeds (). The obtained result was in compliance with the findings of Vlachos et al. (Citation2006). The presence of a C-H-aromatic bending vibration functional group in refined oils and synthesised fatty acid methyl ester was indicated by a peak in the transmission band with a wave number ranging from 750 to 400 cm−1 (O’Donnell et al. Citation2013).
3.3.2. The physicochemical characteristics of synthesised biodiesel from hybridised oils of A. indica and M. stenopetala seeds
The physicochemical characteristics of the synthesised biodiesel were evaluated according to ASTM and EN 14214 standards for the maximum FAME yield (92%) obtained when the oil hybridisation ratio of A25M75 (a mixture of 25% A. indica and 75% M. stenopetala seed oils) reacted with a 2%w/v catalyst dose for 90 min as per experimental run 7 (). The experimental results were recorded as one standard deviation from the mean value (mean ± SD). The evaluated physicochemical characteristics were kinematic viscosity (3.42 ± 0.08 mm2/s), a higher heating value (41.86 ± 0.12 MJ/kg), specific gravity (0.89 ± 0.01), cetane number (62.48 ± 0.38), acid number (0.18 ± 0.04 mg KOH/g), flash point (172.65 ± 1.42 °C), free glycerin (0.014 ± 0.002%mass), total glycerin (0.018 ± 0.003%mass), cloud point (5.24 ± 0.80 °C), water and sediment (0.04 ± 0.01%vol.), methanol content (0.03 ± 0.01%), oxidation stability (7.68 ± 0.40 h), pour point (3.86 ± 0.78 °C), Mg2+ and Ca2+ (4.12 ± 0.18 mg/kg) and the sulfur content (0.04 ± 0.008 mg/kg) (). These results signified that the fuel quality of synthesised biodiesel was in compliance with the standard limit of the FAME quality specification. Therefore, biodiesel produced by mixing or hybridising of Azadirachta indica and Moringa stenopetala seed oil was appropriate fuel that could supersede petroleum and could be utilised as the transport fuel in the engine.
Table 7. Analysis of Physicochemical characteristics of the synthesised FAME.
In this experimentation, the production of FAME involves the hybridisation of A. indica and M. stenopetala seed oils at various proportions (%w/w) while synthesising the biodiesel with ameliorated fuel quality than biodiesel obtained from single feedstocks. The improved fuel quality of the newly synthesised biodiesel includes higher heating value (41.86 ± 0.12 MJ/kg), specific gravity (0.89 ± 0.01), cetane number (62.48 ± 0.38), flash points (172.65 ± 1.42 °C), and other parameters. This improvement is induced by high amount of mono-unsaturated fatty acids (C18H32O2 – oleic acid) comprised in A. indica and M. stenopetala oils. For instance, the presence of about fifty-one percent C18H32O2 in A. indica seed oil (Adepoju and Olawale Citation2015) and seventy-six percent C18H32O2 in M. stenopetala seed oil (Andinet et al. Citation2010) made a significant contribution to meliorate the fuel quality of the synthesised FAME from the hybridised or mixed non-edible oils of the considered feedstocks. Hence, the produced biodiesel exhibits the improved fuel characteristics better than the FAME synthesised from a single non-edible vegetable oil.
4. Conclusion
Biodiesel is a mixture of mono-alkyl esters produced through a base-catalyzed transesterification of animal fats and vegetable oils in the presence of suitable alcohol. It has been perceived as biodegradable, eco-friendly, greener, cleaner, and an alternative source of energy. When synthesised and utilised sustainabley, biodiesel can supersede petroleum, and hence, it can make a significant contribution to overcoming challenges associated with energy and economic crises, hastened global warming and environmental defilement because of the particulate matter and gaseous emissions during utilisation of conventional diesel fuels. This study aims at producing the FAME with ameliorated fuel characteristics via mixing or hybridisation of A. indica and M. stenopetala seed oils (i.e. in situ hybridisation) in the presence of CH3OH and NaOH thereby replacing the conventional diesel fuels.
To conduct the experimentation, the Box-Behnken Design was used in the design of the experiment and evaluation of experimental results. The considered experimental variables were the oil hybridisation ratio (%w/w), reaction time (minutes), and catalyst dose (%w/v) with 3 levels for each variable, −1 (low), 0 (medium), and +1 (high). The hybridised oil ratios involved in the base-catalyzed transesterification reaction were A25M75 (a mixture of 25%w/w A. indica and 75%w/w M. stenopetala oils), A50M50 (a mixture of 50%w/w A. indica and 50%w/w M. stenopetala oils), and A75M25 (a mixture of 75%w/w A. indica and 25%w/w M. stenopetala oils) with their respective biodiesel yield, BA25M75, BA50M50, BA75M25, whereas, the reaction times were 30, 60 and 90 min, and the catalyst doses (NaOH) of a 0.50%w/v, 2.00%w/v, and 3.50%w/v. The other experimental factors, such as agitation speed (300 rpm), molar ratios of oil-to-methanol (1:6), and reaction temperature (60 °C), were kept constant during the experimentation. Seventeen experimental runs were conducted and the biodiesel yield (%) was obtained for each experimental run. The results of the ANOVA signified that the quadratic model expression was developed for the executed experiment with a P < 0.0001 (significant). The quality and adequacy of the developed model were determined by the determination coefficients: R2 = 0.9986, Pred-R2 = 0.9830, Adj-R2 = 0.9968, and a lack of fit test with a P = 0.1150 (insignificant) at 5% the LSD. The prediction coefficient, ‘Pred-R2 of 0.9830’ is in agreement with the adjusted coefficients of determination, ‘Adj-R2’ of 0.9968, showing that the obtained model expression can be employed for the navigation of the design space. The minimum percentage of FAME yield (60.96%) was obtained when the oil hybridisation ratio, A50M50 (a mixture of 50%w/w A. indica oil and 50%w/w M. stenopetala seed oil) reacted with a 0.5%w/w of NaOH catalyst for 30 min whereas, the maximum percentage of the FAME yield (92%) was obtained when the oil hybridisation ratio, A25M75 (i.e. a mixture of 25%w/w A. indica oil and 75%w/w M. stenopetala seed oil) reacted with a 2%w/w NaOH catalyst for 90 min. The analysis of the FTIR () and evaluation of physicochemical properties were executed following the ASTM and EN 14214 standard methods for the synthesised biodiesel (). The quality of biodiesel synthesised from the hybridised oils was in compliance with the standard limits of biodiesel specification showing that the biodiesel can be employed in the diesel engine.
Acknowledgements
The author is indebted to the Forest Products Innovation Center of Excellence (FPICE), Ethiopian Forestry Development and Addis Ababa Institute of Technology for providing logistics and laboratory facilities to conduct the study. The author would also like to extend gratitude for the laboratory personnel of FPICE, for their cooperation and fruitful contributions during experimentation and data analysis.
Disclosure statement
No potential conflict of interest was reported by the author(s).
Data availability
The data and materials of this research are available by requesting from the corresponding author.
Additional information
Funding
References
- Adejumo, A., and D. Abayomi. 2012. “Effect of Moisture Content on Some Physical Properties of Moringa Oleifera Seed.” Journal of Agriculture and Veterinary Science 1: 12–21. http://www.iosrjournals.org/iosr-javs/papers/vol1-issue5/B0151221.pdf.
- Adepoju, A., and O. Olawale. 2015. “Optimization and Predictive Capability of Rsm Using Controllable Variables in Azadiracha Indica Oilseeds Extraction Process.” International Journal of Chemistry and Materials Research 3: 1–10. https://doi.org/10.18488/journal.64/2015.3.1/64.1.1.10.
- American Society for Testing and Materials, (ASTM, D445-21e1). 2022. “Standard Test Method for Kinematic Viscosity of Transparent and Opaque Liquids (and Calculation of Dynamic Viscosity).” https://doi.org/10.1520/D0445-21E01.
- American Society for Testing and Materials, ASTM D5853-17a. 2017. “Standard Test Method for Pour Point of Crude Oils.” https://doi.org/10.1520/D5853-17A.
- American Society for Testing aterials, ASTM D6584-21. 2021. “Standard Test Method for Determination of Total Monoglycerides, Total Diglycerides, Total Triglycerides, and Free and Total Glycerin in B-100 Biodiesel Methyl Esters by Gas Chromatography.” https://doi.org/10.1520/D6584-21.
- American Society for Testing Materials, ASTM D1298-12b(2017)e1. 2023. “Standard Test Method for Density, Relative Density, or API Gravity of Crude Petroleum and Liquid Petroleum Products by Hydrometer Method.” https://doi.org/10.1520/D1298-12BR17E01.
- American Society for Testing Materials, ASTM D240. 2019. “Standard Test Method for Heat of Combustion of Liquid Hydrocarbon Fuels by Bomb Calorimeter.” https://doi.org/10.1520/D0240-19.
- American Society for Testing Materials, ASTM, D2500. 2023. “Standard Test Method for Cloud Point of Petroleum Products and Liquid Fuels.” https://doi.org/10.1520/D2500-23.
- American Society for Testing Materials, ASTM D2709. 2022. “Standard Test Method for Water and Sediment in Middle Distillate Fuels by Centrifuge.” https://doi.org/10.1520/D2709-22.
- American Society for Testing Materials, ASTM D4052. 2022. “Standard Test Method for Density, Relative Density, and API Gravity of Liquids by Digital Density Meter.” https://webstore.ansi.org/standards/astm/astmd405222?source=blog&_gl=1*hcgd2v*_gcl_au*MTE5NjQ1MTA3MC4xNjkzMzAzMzE3.
- American Society for Testing Materials, ASTM D5453. 2019. “Standard Test Method for Determination of Total Sulfur in Light Hydrocarbons, Spark Ignition Engine Fuel, Diesel Engine Fuel, and Engine Oil by Ultraviolet Fluorescence.” https://doi.org/10.1520/D5453-19A.
- American Society for Testing Materials, ASTM D613. 2023. “Standard Test Method for Cetane Number of Diesel Fuel Oil.” https://doi.org/10.1520/D0613-23.
- American Society for Testing Materials, ASTM D664. 2019. “Standard Test Method for Acid Number of Petroleum Products by Potentiometric Titration.” https://doi.org/10.1520/D0664-18E02.
- American Society for Testing Materials, ASTM D93. 2020. “Standard Test Methods for Flash Point by Pensky-Martens Closed Cup Tester0”. https://doi.org/10.1520/D0093-20.
- American Standards of Testing Materials, ASTM D6751. 2023. “Standard Specification for Biodiesel Fuel Blend Stock (B100) for Middle Distillate Fuels.” https://doi.org/10.1520/D6751-23.
- Andinet, E., A. Asfaw, N. Asfaw, and P. Licence. 2010. “Moringa stenopetala Seed Oil as a Potential Feedstock for Biodiesel Production in Ethiopia.” Green Chemistry 12 (2): 316–320. https://doi.org/10.1039/B916500B.
- Andrew, C., E. Eboka, and F. L. Inambao. 2016. “Hybridization of Feedstocks – A New Approach in Biodiesel Development: A Case of Moringa and Jatropha Seed Oils.” Energy Sources, Part A: Recovery, Utilization, and Environmental Effects 38: 1495–1502. https://doi.org/10.1080/15567036.2014.934413.
- Aslan, N., and Y. Cebeci. 2007. “Application of Box–Behnken Design and Response Surface Methodology for Modeling of Some Turkish Coals.” Fuel 86 (1-2): 90–97. https://doi.org/10.1016/j.fuel.2006.06.010.
- Azadbakht, M., S. M. Ardebili, and M. Rahmani. 2023. “A Study on Biodiesel Production Using Agricultural Wastes and Animal Fats.” Biomass Conversion and Biorefinery 13 (6): 4893–4899. https://doi.org/10.1007/s13399-021-01393-1.
- Bekirogullari, M., I. S. Fragkopoulos, and J. K. Pittman. 2017. “Production of Lipid-Based Fuels and Chemicals from Microalgae: An Integrated Experimental and Model-Based Optimization Study.” Algal Research 23: 78–87. https://doi.org/10.1016/j.algal.2016.12.015.
- Brahma, S., B. Nath, B. Basumatary, B. Das, P. Saikia, K. Patir, and S. Basumatary. 2022. “Biodiesel Production from Mixed Oils: A Sustainable Approach Towards Industrial Biofuel Production.” Chemical Engineering Journal Advances 10: 100284. https://doi.org/10.1016/j.ceja.2022.100284.
- Caetano, N. S., T. M. Mata, A. A. Martins, and M. C. Felgueiras. 2017. “New Trends in Energy Production and Utilization.” Energy Procedia 107: 7–14. https://doi.org/10.1016/j.egypro.2016.12.122.
- Degnechew, G. 2021a. “Factors Affecting Biodiesel Production from Non-Edible Vegetable Oil Via Base-Catalyzed Transesterification Process: Synthesis.” International Journal of Sustainable Energy 10: 85–91. https://doi.org/10.11648/j.ijrse.20211003.11.
- Degnechew, G. D. 2021b. “Production and Characterization of Biodiesel by Hybridization of Moringa stenopetala and Azadirachta indica Seed Oil. Addis Ababa Institute of Technology (AAiT) School of Chemical and Bio-Engineering, Master’s thesis.” http://etd.aau.edu.et/bitstream/handle/123456789/27681/Degnechew%20Genene.pdf?isAllowed=y&sequence=1.
- Domínguez, Y., D. Tabio García, E. Fernández-Santana, M. Rondon Macias, L. Goyos Pérez, R. Piloto-Rodríguez, and T. Fischer. 2019. “Rheological Behavior and Properties of Biodiesel and Vegetable Oil from Moringa oleifera Lam.” Afinidad, Journal of Chemical Engineering, Theoretical and Applied Chemistry 76: 587. https://raco.cat/index.php/afinidad/article/view/361480.
- Dube, M., S. Zheng, D. McLean, and M. Kates. 2004. “A Comparison of Attenuated Total Reflectance-FTIR Spectroscopy and GPC for Monitoring Biodiesel Production.” Journal of the American Oil Chemists’ Society 81 (6): 599–603. https://doi.org/10.1007/s11746-006-0948-x.
- European Union’s, EN 14110. 2019. “Fat and Oil Derivatives, Fatty Acid Methyl Esters, Determination of Methanol Content.” https://www.techstreet.com/standards/bs-en-14110-2019?product_id=2042571.
- European Union’s EN 14112. 2020. “Fat and Oil Derivatives - Fatty Acid Methyl Esters (FAME) - Determination of Oxidation Stability (Accelerated Oxidation Test).” https://standards.iteh.ai/catalog/standards/cen/aa4b70be-e467-4a60-83ef-c2543b937f19/en-14112-2020.
- European Union’s EN 14538. 2006. “Fat and Oil Derivatives - Fatty Acid Methyl Ester (FAME) - Determination of Ca, K, Mg and Na Content by Optical Emission Spectral Analysis with Inductively Coupled Plasma (ICP OES).” https://global.ihs.com/doc_detail.cfm?document_name=DIN%20EN%20138&item_s_key=00489408.
- Güney, T., and K. Kantar. 2020. “Biomass Energy Consumption and Sustainable Development.” International Journal of Sustainable Development 27: 762–767. https://doi.org/10.1080/135009.2020.1753124.
- Holechek, J. L., and H. M. Geli. 2022. “A Global Assessment: Can Renewable Energy Replace Fossil Fuels by 2050?” Sustainability 14 (8): 4792. https://doi.org/10.3390/su14084792.
- Jaggi, N., and D. Vij. 2006. Fourier Transform Infrared Spectroscopy. Boston: Springer, 411–450. https://doi.org/10.1007/0-387-37590-2_9.
- Kabutey, A., D. Herák, and Č Mizera. 2022. “Determination of Maximum Oil Yield, Quality Indicators and Absorbance Spectra of Hulled Sunflower Seeds Oil Extraction Under Axial Loading.” Foods (basel, Switzerland) 11: 2866. https://doi.org/10.3390/foods11182866.
- Kumar, M. V., V. A. Babu, and R. P. Kumar. 2018. “The Impacts on Combustion, Performance and Emissions of Biodiesel by Using Additives in Direct Injection Diesel Engine.” Alexandria Engineering Journal 57 (1): 509–516. https://doi.org/10.1016/j.aej.2016.12.016.
- Lola, N., C. Aleh, and J. Jessica. 2022. “Phases of Fossil Fuel Decline: Diagnostic Framework for Policy Sequencing and Feasible Transition Pathways in Resource Dependent Regions.” Oxford Open Energy 1: oiac002. https://doi.org/10.1093/ooenergy/oiac002.
- Mamoona, M., S. Muhammad, A. Mushtaq, et al. 2023. “Cleaner Production of Biodiesel from Novel non-Edible Seed Oil (Carthamus lanatus L.) via Highly Reactive and Recyclable Green Nano CoWO3@rGO Composite in Context of Green Energy Adaptation.” Fuel 332: 126265. https://doi.org/10.1016/j.fuel.2022.126265.
- Mizik, T., and G. Gyarmati. 2021. “Economic and Sustainability of Biodiesel Production- A Systematic Literature Review.” Clean Technologies 3: 19–36. https://doi.org/10.3390/cleantechnol3010002.
- Motevali, A., N. Hooshmandzadeh, E. Fayyazi, M. Valipour, and J. Yue. 2023. “Environmental Impacts of Biodiesel Production Cycle from Farm to Manufactory: An Application of Sustainable Systems Engineering.” Atmosphere 14: 399. https://doi.org/10.3390/atmos14020399.
- Neupane, D. 2023. “Biofuels from Renewable Sources, a Potential Option for Biodiesel Production.” Bioenginering 10 (1): 29. https://doi.org/10.3390/bioengineering10010029.
- O’Donnell, S., I. Demshemino, M. Yahaya, I. Nwadike, and L. Okoro. 2013. ““A Review on the Spectroscopic Analyses of Biodiesel.” European Journal of Engineering Science and Technology 2 (7): 137–146.
- Okechukwu, O., E. Joseph, U. C. Nonso, and N. Kenechi. 2022. “Improving Heterogeneous Catalysis for Biodiesel Production Process.” Cleaner Chemical Engineering 3: 100038. https://doi.org/10.1016/j.clce.2022.100038.
- Oliveira, H., and V. Moutinho. 2021. “Renewable Energy, Economic Growth and Economic Development Nexus: A Bibliometric Analysis.” Energies 14 (15): 78. https://doi.org/10.3390/en141578.
- Oyerinde, A. Y., and E. I. Bello. 2015. “Use of Fourier Transformation Infrared (FTIR) Spectroscopy for Analysis of Functional Groups in Peanut Oil Biodiesel and Its Blends.” Current Applied Sciences 13: 1–14. https://doi.org/10.9734/BJAST/2016/22178.
- Ozturk, H. 2014. “Energy Analysis for Biodiesel Production from Rapeseed Oil.” Energy Exploration & Exploitation 32 (6): 1005–1031. https://doi.org/10.1260/0144-5987.32.6.1005.
- Peterson, K. O., and O. Ercan. 2023. “Global Energy Crisis: Impact on the Global Economy. In Book: The Impact of Climate Change and Sustainability Standards on the Insurance Market.” SSRN Electronic Journal, https://doi.org/10.2139/ssrn.4309828.
- Rabelo, S. N., V. P. Ferraz, L. S. Oliveira, and A. S. Franca. 2015. “FTIR Analysis for Quantification of Fatty Acid Methyl Esters in Biodiesel Produced by Microwave-Assisted Transesterification.” International Journal of Environmental Science and Development 6: 964–969. https://doi.org/10.7763/IJESD.2015.V6.730.
- Rosset, M., and O. W. Perez-Lopez. 2019. “FTIR Spectroscopy Analysis for Monitoring Biodiesel Production by Heterogeneous Catalyst.” Vibrational Spectroscopy 105: 102990. https://doi.org/10.1016/j.vibspec.2019.102990.
- Singh, N., R. Nyuur, and B. Richmond. 2019. “Renewable Energy Development as a Driver of Economic Growth: Evidence from Multivariate Panel Data Analysis.” Sustainability 11: 2418. https://doi.org/10.3390/su11082418.
- Vlachos, N., Y. Skopelitis, M. Psaroundaki, V. Konstantinidou, A. Chatzilazarou, and E. Tegou. 2006. “Applications of Fourier Transform-Infrared Spectroscopy to Edible Oils.” Analytica Chimica Acta 573-574: 459–465. https://doi.org/10.1016/j.aca.2006.05.034.
- Wang, F., J. D. Harindintwali, Z. Yuan, et al. 2021. “Technologies and Perspectives for Achieving Carbon Neutrality.” The Innovation 2 (4): 100180. https://doi.org/10.1016/j.xinn.2021.100180.
- Xiao, J., J. Wu, Y. Chao, R. Liu, C. Li, and Z. Xiao. 2022. “Evaluation of Yields and Quality Parameters of Oils from Cornus wilsoniana Fruit Extracted by Subcritical n-Butane Extraction and Conventional Methods.” Grain & Oil Science and Technology 5 (4): 204–212. https://doi.org/10.1016/j.gaost.2022.09.003.
- Xuejun, L., H. He, and S. Zhu. 2007. “Transesterification of Soybean oil to Biodiesel Using SrO as a Solid Base Catalyst.” Catalysis Communications 8 (7): 1107–1111. https://doi.org/10.1016/j.catcom.2006.10.026.
- Zhang, Y., Y. Zhong, J. Wang, D. Tan, Z. Zhang, and D. Yang. 2021. “Effects of Different Biodiesel-Diesel Blend Fuel on Combustion and Emission Characteristics of a Diesel Engine.” Processes 9. https://doi.org/10.3390/pr9111984.
- Zou, C., Q. Zhao, and G. Zhang. 2016. “Energy Revolution: From a Fossil Energy Era to a New Energy Era.” Natural Gas Industry B 3 (1): 1–11. https://doi.org/10.1016/j.ngib.2016.02.001.