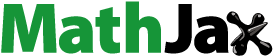
ABSTRACT
Demand for electricity and cooling is increasing due to population growth, which in turn increases pollution. Renewable energy sources are gaining popularity due to their lower levels of pollution. Resource utilisation efficiency is an important factor in maximising the efficiency of low-temperature heat sources. In this study, a vapour absorption cooling cycle and a Kalina power cycle were merged. At the economiser, heat is recovered from the heat exchanger and divided into two basic solution streams. The high-pressure flow passes through the boiler evaporator to produce wet steam, while another stream is throttled to an intermediate pressure. The thermal efficiency of the cycle improves with increasing separator temperature, separator pressure, concentration, and heat recovery temperature, but there is an optimal separator temperature for resource utilisation capacity. Increasing separator pressure and heat recovery temperatures improves resource utilisation efficiency. The proposed cycle has a better temperature match in the heating process. The resource utilisation efficiency of the present cycle is 31.13%, which is 90.63% higher than the reference study.
Nomenclature
E | = | exergy |
rec | = | rectifier |
abs | = | absorber |
P | = | pressure |
T | = | temperature |
h | = | specifc enthalpy |
η | = | efficiency |
x | = | concentration |
w | = | work |
m | = | mass |
Subscripts | ||
1,2. | = | number |
o | = | output |
i | = | input |
ref | = | refrigeration |
c | = | cold water |
l | = | liquid |
amb | = | ambient |
p | = | pump |
t | = | turbine |
isen | = | isentropic |
r | = | rich |
s | = | saturated |
ps | = | power stream |
bs | = | basic solution |
1. Introduction
The world’s energy consumption is increasing rapidly, with most of this energy coming from fossil fuels (Tabasi et al. Citation2018). However, the consumption of fossil fuel resources has become problematic due to pollution. Renewable energy resources are a cleaner alternative, but their poor efficiency hinders immediate transformation. In light of this, there has been a growing emphasis on the effective utilisation of energy resources and renewable energy. Low-grade heat, such as industrial waste and solar heat, has emerged as a promising solution to this problem. Multi-generation and poly-generation systems have gained popularity due to their advantages over conventional generation systems (Colakoglu and Durmayaz Citation2021). These systems enable the generation of electricity, cooling, heating, drying, etc. from a single source.
The conventional power generation cycle is not the right option for generating power from a low-grade heat source. Some research has been done in this area in an attempt to build systems that are suitable for generating electricity from low-grade heat sources. In these studies, organic Rankine cycle (Saleh et al. Citation2007) and Kalina cycles (Kalina Citation1984; Zhang, He, and Zhang Citation2012) have received wide attention from researchers.
Peng et al. (Citation2012) proposed a gas turbine-Kalina cycle combination with intercooling. In this study, solar energy is used for boosting the performance of the cycle. Sun, Ikegami, and Jia (Citation2012) studied the solar-powered Kalina cycle for power generation with a low-grade heat source. Arslan (Citation2011) investigated the low-grade geothermal heat source for power generation. In this study, the Kalina-34 cycle is optimised for life cycle cost analysis. The binary cycles (i.e. organic Rankine cycle and Kalina cycle) have higher exergy efficiency, especially in the case of a low-grade heat source. The Kalina cycle generates more power for the same heat input compared to the organic Rankine cycle (DiPippo Citation2004).
Shankar and Srinivas contributed some efforts to the implementation of a low-temperature heat source-based Kalina cycle (Ganesh and Srinivas Citation2012; Citation2013). The Kalina cycle produces maximum work at relatively low source temperatures than the higher efficiency offering source temperatures. In comparison to a series heat source arrangement, the parallel heat source temperature improves cycle performance. Guo et al. (Citation2015) analyzed the dual pressure Kalina power cycle. The studies reported that the cascade heat source arrangement with dual pressure stages enhances the heat source utilisation capability along with additional power generation. Zhu et al. (Citation2016) investigated the Kalina cycle with dual pressure vaporisation. In the mentioned cycle, the high-pressure economiser and low-pressure evaporator are arranged in parallel before the main evaporator.
To create power and cooling at the same time, Goswami and Xu (Citation1999) used a cogeneration cycle that combined the Rankine and vapour absorption refrigeration cycles. In this cycle, the vapour is expanded in the turbine to produce low temperatures. The limited refrigeration effect is generated with the proposed cycle.
Tamm and Goswami (Citation2003) used a combined power and cooling cycle with pressure and temperatures confined to 6 bar and 80°C for the experimental study. Vijayaraghavan and Goswami (Citation2005) investigated alternative working fluids for the combined cycles, with resource utilisation and exergy efficiencies chosen for comparison. Vijayaraghavan and Goswami (Citation2006) modified the cogeneration cycle by incorporating the distillation stage to improve resource utilisation efficiency. In this cycle, the ammonia-water mixture concentration is enriched in an intermediate stage absorber. Zheng et al. (Citation2006) developed a cycle to utilise the turbine exit heat. In this study, two streams of the basic solution are separated. One stream is passed through a reboiler-recuperator arrangement to produce rich vapour for the refrigeration cycle. The rich mixture and second stream form a rich solution in the second absorber. Zhang and Lior (Citation2007) investigated parallel Rankine and absorption cycles, studying not only cycle performance but also the temperature match of the heat source. The heat source temperature for this cycle was around 450°C.
Ahmadi, Dincer, and Rosen (Citation2012) investigated a cooling, heating, and power generation system. With increasing turbine inlet temperature and compressor pressure ratio, the exergy efficiency and sustainability index of the suggested trigeneration system improved. Jawahar et al. (Citation2013) analyzed the GAX-based cogeneration cycle with a split ratio of 0.5–0.5. Power and refrigeration streams operate in parallel to each other in the cycle. Pure ammonia is used for both power and refrigeration systems. The GAX arrangement improves internal heat recovery by around 20%. Yu et al. (Citation2014) studied an adjustable refrigeration-to-power ratio for the presented aqua-ammonia cogeneration system. They claimed that the combined efficiency and COP have improved by 6.6% and 100%, respectively, with the same heat input. Han et al. (Citation2014) investigated a power and cooling cogeneration test rig that operated using low-grade waste heat. Shankar and Srinivas (Citation2014) introduced the Triple Pressure Combined Power and Refrigeration Cycle. The proposed cycle would be beneficial for both cooling and power generation. The maximum temperature in the cycle does not affect the refrigeration output, but the power generation increases with superheating temperature. Chaiyat and Kiatsiriroat (Citation2015) tested a power, heating, and cooling trigeneration system experimentally. In the proposed study, the trigeneration cycle efficiency is increased by 7% over the organic Rankine cycle. Wang et al. (Citation2016) developed a power and cooling cogeneration cycle in which the second separator is incorporated after the turbine to separate the ammonia vapour for the refrigeration process. Higher exergy losses are reported in the heat exchangers of the system. Mohtaram, Chen, and Lin (Citation2017) analyzed a Rankine-absorption cogeneration system. In this cycle, the minimum heat source temperature is 550°K. The optimisation is done with a genetic algorithm to achieve maximum energy and exergy efficiencies. Cao et al. (Citation2017) studied a Kalina-based power and refrigeration cogeneration system. The Kalina power cycle is combined with the vapour compression refrigeration system and vapour absorption system individually. The heat is captured from saturated fluid generated in a separator to serve the cooling cycle in the Kalina-absorption cycle. The Kalina cycle is engaged with the vapour compression cycle for generating refrigeration. This study reports that the exergy efficiency of a Kalina-absorption cycle is greater than that of the Kalina-vapour compression refrigeration cycle. Zhang et al. (Citation2018) modified the Kalina cycle into a parallel power and refrigeration Kalina cycle (PPR-KC). The proposed cycle waste heat recovery efficiency is improved with parallel refrigeration technique. Multi-stage absorption is used to generate better concentration. The proposed cycle’s comprehensive power recovery efficiency reaches 27.2% with heat source temperatures of 400°C and ambient water temperature of 30°C, respectively. Parikhani, Ghaebi, and Rostamzadeh (Citation2018) introduced a power and refrigeration cogeneration cycle based on the vapour absorption cycle with a geothermal heat source. In the absorption-power cycle, the saturated liquid flashes into the low-pressure chamber to generate ammonia-water vapour for the cooling sub-cycle. Shankar and Rivera (Citation2020) implemented the power and refrigeration cascade cogeneration system, the cooling sub-cycle mass flow is boosted with a flash separator. The proposed cycle output is growing with the highest temperature and rich solution concentration. At the heat source temperature of 150°C and 0.96 vapour concentration, the implementation is offering a 30% increment in energy utilisation factor in comparison with triple pressure cooling cogeneration cycle. Raveendra Nath, Hemachandra Reddy, and Vijaya Bhaskar Reddy (Citation2022) have improved thermal efficiency of the cogeneration cycle with the help of parallel stream.
Cogeneration cycles work more efficiently than cooling cycles and energy cycles. Resource utilisation efficiency is another important parameter to improve system performance. Parallel cycles offer better performance than series cycles. Cycle efficiency increases with cascade heat source arrangement, The irreversibility between the heat source and working medium decreases with parallel evaporator arrangement. In the present study, the cooling cycle is separated in the evaporator and mounted parallel to the power cycle. Due to the cascade heat source configuration, resource utilisation efficiency improves.
2. System description
represents the outline of the cycle. In the presented diagram, the main cycle is separated into the power cycle (represented by 1–13) and the refrigeration cycle (14–24 & 9–11). The cycle is integrated from the absorber to the economiser. The absorber, pump, and heat exchanger are common devices for both cycles.
In the proposed cycle, the split fraction is to be calculated with the assumed heat recovery temperature (11) in the heat exchanger placed after the absorber. The splitter is arranged before the economiser to separate the streams for the power and refrigeration cycles. Now, the power stream is heated to the stipulated temperature (13). The refrigeration stream is heated to the possible extent in the economiser (15).
Now, the assumed solution at a prescribed pressure, temperature, and concentration is sent to the separator (1). The two-phase fluid is separated into saturated liquid and saturated vapour in the separator (1-2-5). The saturated vapour is passed through the superheater (2–3) before entering the turbine. In a superheater, the superheated vapour is generated by heating the saturated vapour further. Then, the high-pressure superheated vapour expands to a low pressure in the turbine (3–4). The saturated liquid separated in the separator is throttled (5–6) to the low pressure. The two streams then form together as a mixture (6-4-7) and flow into the solution heat exchanger. The solution temperature is dropped in the heat exchanger (7–8) before entering into the absorber, where the refrigeration stream solutions (23 & 24) and power stream (8) mixtures combine and form a basic solution (9) at low pressure.
Then the solution is passing through the pump (9–10) and solution heat exchanger (10–11). The basic solution is split into a power stream (12) and a refrigeration stream (14). The basic solution pressure reaches a higher value in the pump, then captures heat from the solution heat exchanger. The basic solutions are preheated in the economiser separately (12–13 & 14–15). The power stream solution is almost heated to saturation temperature, while the refrigeration stream is heated to a possible extent. Then the power stream passes through the boiler evaporator and continues the cycle. The refrigeration stream is throttled to flash pressure (16) to form the two-phase solution in the flash chamber. The flashed fluid is separated into vapour (17) and liquid (18). In the flash chamber, the generated vapour will pass through the rectifier to get enriched (20). The rich vapour from the rectifier is sent to the condenser, while the saturated liquid (19) comes back to the flash chamber and leaves along with the saturated liquid generated in the flashing. The enriched vapour is passed through the condenser (21) to cool down to a lower temperature. Then it is expanded to lower pressure (22) in the throttle valve. During the expansion, the solution temperature falls down to the lowest temperature in the cycle. To provide a cooling effect, it is directed to the refrigeration evaporator. In the evaporator, it absorbs some heat (23). The saturated liquid from the flash chamber is delivered to the absorber at low pressure (24) and fed to the absorber.
3. Methodology
The Kalina flash combination cycle is depicted as a line diagram in . and shows a comparative analysis between the results of the proposed model and those documented in the existing literature (Raveendra Nath, Hemachandra Reddy, and Vijaya Bhaskar Reddy Citation2022). The proposed model exhibits a noteworthy agreement with only slight deviations. The relevant data is presented in the respective tables. The system is designed using the principles of concentration, mass, and energy balancing. Some assumptions are made to simplify the system.
Table 1. Power.
Table 2. Refrigeration.
All the equipment in the system is treated as open systems, and the system is assumed to be in a steady state. The design disregards potential and kinetic energies, and pressure and heat losses are not taken into account during the design process. Equipment performance parameters are assumed to be constant. The assumed boundary conditions are tabulate in . In modelling, four key parameters are analyzed for the proposed cycle: separator temperature, pressure, basic solution concentration, flash pressure, and heat recovery temperature. The boundary conditions and basic solution concentration are used to compute the cycle’s low pressure.
Table 3. Boundary conditions for performance evaluation.
The split fraction for the refrigeration and separator streams is calculated based on the heat recovery temperature and energy balancing equations. The proposed model is developed using ammonia-water thermal properties estimated from REFPROP 9.1 and MATLAB software. In the heat exchangers, the concentration of hot and cold fluids is not changed. In this analysis, the split fraction of the refrigeration and power streams is derived from the heat exchanger for a given terminal temperature difference. The remaining governing equations for the energy and exergy balance are given by the following equations.
(1)
(1)
(2)
(2) In the separator and flash chamber, the main function is to separate the wet vapour into saturated vapour and liquid. The mass, energy, and concentration balancing equations are used to solve the mathematical model. The employed equations are given as follows.
(3)
(3)
(4)
(4)
(5)
(5)
Isenthalpic throttling process is assumed.
(6)
(6) The flash chamber and single-stage rectifier are assumed. Flash-rectifier mass and energy balance are as follows
(7)
(7)
(8)
(8)
(9)
(9) The effictiveness of dephlagmator (Shankar and Srinivas Citation2018) is given by
(10)
(10)
(11)
(11) The absorber mass, energy and concentration balance is as follows
(12)
(12)
(13)
(13)
(14)
(14)
(15)
(15) The work consumed by the pump is represented as
(16)
(16)
(17)
(17) The work generated by the turbine is represented as
(18)
(18)
(19)
(19) the split fraction is defined as the fraction of power stream mass flow rate and is given by
(20)
(20)
(21)
(21) The planned plant’s thermal efficiency is calculated as
(22)
(22) Heat supplied by the heat source is given by
(23)
(23) The refrigeration effect is calculated as
(24)
(24) The resource utilisation efficiency is defined (Vijayaraghavan and Goswami Citation2006) as
(25)
(25) Ein is given by
(26)
(26) Where Eref is defined as follows
(27)
(27)
4. Result and discussion
The proposed cycle is evaluated with changes in separator temperature, basic solution concentration, separator pressure, flash chamber pressure, and heat recovery temperature. The performance parameters are analyzed with turbine work, cooling effect, cooling exergy, thermal efficiency, and resource utilisation efficiency. The change in key variable parameters, i.e. mass split ratio, turbine mass flow rate, rich refrigerant mass flow rate, hot fluid exit temperature, and refrigeration stream temperature, are presented. The initial conditions for the cycle evaluation are tabulated in . The system properties at the state points are presented in .
Table 4. Properties of working fluid in the cycle.
4.1. Separator temperature
illustrates the effect of separator temperature on cycle performance. As the temperature of the separator rises, the specific enthalpy drop in the turbine and the mass flow rate of the turbine also increase while the heat input to the system decreases. As the heat available in the heat exchanger increases, the mass flow rate of the basic solution also increases. Turbine work, cooling effect, and cooling exergy all increase with increasing mass flow rate. Thermal efficiency increases with the increase in total output. However, the contribution of the cooling effect is limited in the resource utilisation efficiency. Resource utilisation efficiency increases gradually.
4.2. Separator pressure
describes the performance variation with a change in separator pressure in the cycle. As the separator pressure increases, the expansion ratio in the turbine increases, and the generation of ammonia vapour decreases in the separator. As a result, the turbine work output increases. The dryness fraction in the separator decreases due to the pressure rise, and the heat consumption decreases in the boiler. The mass split ratio increases due to the decrease in heat availability in the heat exchanger. Because of the increase in pressure, the boiling temperature of the basic solution increases, and the refrigeration stream temperature and pressure increase before flashing. Due to the change in the flash pressure ratio, the rich ammonia mass flow rate increases in the refrigeration stream. The refrigeration effect and refrigeration exergy grow with the increase in mass flow rate. The thermal and resource utilisation efficiencies grow with an increase in total output.
4.3. Basic solution concentration
shows how the concentration of the basic solution affects the proposed cycle’s performance. The dryness fraction in the separator increases as the basic solution concentration rises. The turbine work increases as the saturated ammonia vapour flow rate rises. The increase in the heat in the heat exchanger increases the basic solution mass flow rate, leading to a fall in the mass split fraction. The refrigeration stream temperature (bubble point temperature) drops due to the increase in concentration. The refrigeration effect increases with an increase in rich ammonia mass flow rate. However, the lowest pressure in the cycle increases with an increase in ammonia concentration, leading to a rise in the evaporator inlet temperature. The refrigeration exergy falls with a drop in the evaporator temperature difference. The thermal efficiency of the cycle increases with an increase in turbine work and the refrigeration effect, but the resource utilisation efficiency falls with a drop in refrigeration exergy.
4.4. Heat recovery temperature
displays the effect of heat recovery temperature on cycle performance. In this study, the heat recovery temperature is an important parameter in basic solution mass flow rate calculation. An increase in the heat recovery temperature decreases the mass flow rate of the basic solution, leading to an increase in mass split fraction. The power cycle is not affected by the change in heat recovery temperature. The decrease in the refrigeration stream mass flow rate helps to increase the refrigeration stream temperature until it reaches its maximum value, while the decrease in the basic solution mass flow rate increases the heat source exit temperature. Due to the increase in the refrigeration stream temperature, the mass flow rate of rich ammonia increases. After the refrigeration temperature is maintained at a constant value, the decrease in the basic solution mass flow rate causes a drop in the refrigeration effect and refrigeration exergy. The thermal and resource utilisation efficiencies initially increase and then decrease due to the drop in refrigeration output.
4.5. Comparision
represents the T-Q diagrams of heat supply processes in the cogeneration cycle. The proposed cycle is flashing from the economiser. The current cycle is compared to the cogeneration cycle which is flashing from the separator (Raveendra Nath, Hemachandra Reddy, and Vijaya Bhaskar Reddy Citation2022). The diagrams show the temperature changes with respective heat transfer in different components. Conditions at the outlet of the superheater, boiler, economiser, and inlet of the economiser are denoted by A, B, C, and D respectively for the working fluid. Similarly, the conditions at the inlet of the superheater, boiler, economiser, and the outlet of the economiser are denoted by A’, B’, C’, and D’, respectively, for the heat source. The T-Q diagrams of the two cogeneration cycles are compared with each other. The temperature changes in the superheater are similar in both cycles, but in the present cycle, the heat utilisation in the economiser is increased, which results in a decrease in the temperature difference (i.e. the difference between the heat source exit temperature and the working fluid inlet temperature) at the economiser. The cooling effect is increasing due to the increased heat consumption in the economiser, which is leading to an increase in resource utilisation efficiency.
illustrates the comparison between the separator-flash cycle and economiser-flash cycle.
Table 5. Comparing the evaporator-flash cycle with the separator-flash cycle.
Existing work and current work were compared with equal heat supply, 0.5 base solution concentration, and heat source temperature below 155°C. This cycle requires less exergy input for equal heat input due to higher resource utilisation efficiency. The heat rejection in the absorber is higher for the separator-flash cycle and higher in the condenser for the economiser-flash cycle. The present work has better heat recovery in the heat exchanger. The present cycle is producing three times more refrigerating effect than the separator-flash cycle. The turbine work for the present cycle is 62% less than the separator-flash cycle. The heat source exit temperature of the separator-flash cycle is 118.23°C, 70.71°C in the present cycle. In the present cycle, thermal and resource utilisation efficiencies are 49.71% and 90.63% higher than the separator-flash cycle.
5. Conclusion
The proposed cycle is designed to generate power and refrigeration using low-temperature heat sources such as solar energy, geothermal energy, biomass energies, etc. The heat source utilisation is increased with the help of novel arrangements. In this study, both the power cycle and the refrigeration cycle are run with similar concentrations. The refrigerant mass flow rate is increased by using the basic solution instead of saturated fluid. The turbine work is reduced compared to the reference cycle, while the refrigeration output is increased. A parametric investigation is performed on the Kalina flash cogeneration cycle. Increasing the separator pressure and heat recovery temperature leads to improved resource utilisation efficiency. The proposed cycle is capable of large heat recovery and utilisation, efficiently utilising heat below the bubble point temperature for the cooling cycle. The increased heat utilisation capability results in higher resource utilisation efficiency of the cycle, which is calculated to be 31.13%. The proposed cycle shows better resource utilisation efficiency and better temperature matching with the heat source compared to the reference cycle.
Data availability statement
Simulation data submitted for review. It is displayed in the form of graphs in the manuscript. The values of the cycle at the component connections are displayed in tabular form.
Disclosure statement
No potential conflict of interest was reported by the author(s).
References
- Ahmadi, Pouria, Ibrahim Dincer, and Marc A. Rosen. 2012. “Exergo-environmental Analysis of an Integrated Organic Rankine Cycle for Trigeneration.” Energy Conversion and Management 64:447–453. IREC 2011. The International Renewable Energy Congress. https://doi.org/10.1016/j.enconman.2012.06.001.
- Arslan, Oguz. 2011. “Power Generation from Medium Temperature Geothermal Resources: Ann-Based Optimization of Kalina Cycle System-34.” Energy 36 (5): 2528–2534. https://doi.org/10.1016/j.energy.2011.01.045.
- Cao, Liyan, Jiangfeng Wang, Hongyang Wang, Pan Zhao, and Yiping Dai. 2017. “Thermodynamic Analysis of a Kalina-based Combined Cooling and Power Cycle Driven by Low-grade Heat Source.” Applied Thermal Engineering 111:8–19. https://doi.org/10.1016/j.applthermaleng.2016.09.088.
- Chaiyat, Nattaporn, and Tanongkiat Kiatsiriroat. 2015. “Analysis of Combined Cooling Heating and Power Generation from Organic Rankine Cycle and Absorption System.” Energy 91:363–370. https://doi.org/10.1016/j.energy.2015.08.057.
- Colakoglu, Mert, and Ahmet Durmayaz. 2021. “Energy, Exergy and Environmental-based Design and Multiobjective Optimization of a Novel Solar-Driven Multi-generation System.” Energy Conversion and Management 227:113603. https://doi.org/10.1016/j.enconman.2020.113603.
- DiPippo, Ronald. 2004. “Second Law Assessment of Binary Plants Generating Power from Low-temperature Geothermal Fluids.” Geothermics 33 (5): 565–586. https://doi.org/10.1016/j.geothermics.2003.10.003.
- Ganesh, N. Shankar, and T. Srinivas. 2012. “Design and Modeling of Low Temperature Solar Thermal Power Station.” Applied Energy 91 (1): 180–186. https://doi.org/10.1016/j.apenergy.2011.09.021.
- Ganesh, N. Shankar, and T. Srinivas. 2013. “Thermodynamic Assessment of Heat Source Arrangements in Kalina Power Station.” Journal of Energy Engineering 139 (2): 99–108. https://doi.org/10.1061/(ASCE)EY.1943-7897.0000100.
- Goswami, D. Yogi, and Feng Xu. 1999. “Analysis of a New Thermodynamic Cycle for Combined Power and Cooling Using Low and Mid Temperature Solar Collectors.”
- Guo, Zhanwei, Zhi Zhang, Yaping Chen, Jiafeng Wu, and Cong Dong. 2015. “Dual-pressure Vaporization Kalina Cycle for Cascade Reclaiming Heat Resource for Power Generation.” Energy Conversion and Management 106:557–565. https://doi.org/10.1016/j.enconman.2015.09.073.
- Han, Wei, Qiang Chen, Liuli Sun, Sijun Ma, Ting Zhao, Danxing Zheng, and Hongguang Jin. 2014. “Experimental Studies on a Combined Refrigeration/Power Generation System Activated by Low-grade Heat.” Energy 74:59–66. https://doi.org/10.1016/j.energy.2014.02.097.
- Jawahar, C.P., R. Saravanan, Joan Carles Bruno, and Alberto Coronas. 2013. “Simulation Studies on Gax Based Kalina Cycle for Both Power and Cooling Applications.” Applied Thermal Engineering 50 (2): 1522–1529. https://doi.org/10.1016/j.applthermaleng.2011.11.004.
- Kalina, A.I. 1984. “Combined-cycle System with Novel Bottoming Cycle.” Journal of Engineering for Gas Turbines and Power 106 (4) October: 737–742. https://doi.org/10.1115/1.3239632.
- Mohtaram, Soheil, Wen Chen, and Ji Lin. 2017. “Investigation on the Combined Rankine-Absorption Power and Refrigeration Cycles Using the Parametric Analysis and Genetic Algorithm.” Energy Conversion and Management 150:754–762. https://doi.org/10.1016/j.enconman.2017.08.011.
- Parikhani, Towhid, Hadi Ghaebi, and Hadi Rostamzadeh. 2018. “A Novel Geothermal Combined Cooling and Power Cycle Based on the Absorption Power Cycle: Energy, Exergy and Exergoeconomic Analysis.” Energy 153:265–277. https://doi.org/10.1016/j.energy.2018.01.153.
- Peng, Shuo, Hui Hong, Hongguang Jin, and Zhifeng Wang. 2012. “An Integrated Solar Thermal Power System Using Intercooled gas Turbine and Kalina Cycle.” Energy 44 (1): 732–740. Integration and Energy System Engineering. European Symposium on Computer-Aided Process Engineering 2011. https://doi.org/10.1016/j.energy.2012.04.063.
- Raveendra Nath, R., K. Hemachandra Reddy, and C. Vijaya Bhaskar Reddy. 2022. “A Cogeneration Cycle Comparative Analysis with Parallel Arrangement.” Alexandria Engineering Journal 61 (2): 1171–1181. https://doi.org/10.1016/j.aej.2021.06.018.
- Saleh, Bahaa, Gerald Koglbauer, Martin Wendland, and Johann Fischer. 2007. “Working Fluids for Low-temperature Organic Rankine Cycles.” Energy 32 (7): 1210–1221. https://doi.org/10.1016/j.energy.2006.07.001.
- Shankar, R., and W. Rivera. 2020. “Investigation of new Cooling Cogeneration Cycle Using NH3-H2O Mixture.” International Journal of Refrigeration 114:88–97. https://doi.org/10.1016/j.ijrefrig.2020.02.014.
- Shankar, R., and T. Srinivas. 2014. “Investigation on Operating Processes for a New Solar Cooling Cogeneration Plant.” Journal of Solar Energy Engineering 136 (3). https://doi.org/10.1115/1.4027423.
- Shankar, R., and T. Srinivas. 2018. “Novel Cooling Augmented Cogeneration Cycle.” International Journal of Refrigeration 91:146–157. https://doi.org/10.1016/j.ijrefrig.2018.05.026.
- Sun, Faming, Yasuyuki Ikegami, and Baoju Jia. 2012. “A Study on Kalina Solar System with an Auxiliary Superheater.” Renewable Energy 41:210–219. https://doi.org/10.1016/j.renene.2011.10.026.
- Tabasi, Sanaz, Alireza Aslani, Marja Naaranoja, and Hossein Yousefi. 2018. “Analysis of Energy Consumption in Finland Based on the Selected Economics Indicators.” International Journal of Ambient Energy 39 (2): 127–131. https://doi.org/10.1080/01430750.2016.1269675.
- Tamm, Gunnar, and D. Yogi Goswami. 2003. “Novel Combined Power and Cooling Thermodynamic Cycle for Low Temperature Heat Sources, Part II: Experimental Investigation.” Journal of Solar Energy Engineering 125 (2): 223–229. https://doi.org/10.1115/1.1564080.
- Vijayaraghavan, Sanjay, and D.Y. Goswami. 2005. “Organic Working Fluids for a Combined Power and Cooling cycle.”
- Vijayaraghavan, S., and D.Y. Goswami. 2006. “A Combined Power and Cooling Cycle Modified to Improve Resource Utilization Efficiency Using a Distillation Stage.” Energy 31 (8–9): 1177–1196. https://doi.org/10.1016/j.energy.2005.04.014.
- Wang, Jiangfeng, Jianyong Wang, Pan Zhao, and Yiping Dai. 2016. “Thermodynamic Analysis of a New Combined Cooling and Power System Using Ammonia–Water Mixture.” Energy Conversion and Management 117:335–342. https://doi.org/10.1016/j.enconman.2016.03.019.
- Yu, Zeting, Jitian Han, Hai Liu, and Hongxia Zhao. 2014. “Theoretical Study on a Novel Ammonia–Water Cogeneration System with Adjustable Cooling to Power Ratios.” Applied Energy 122:53–61. https://doi.org/10.1016/j.apenergy.2014.02.010.
- Zhang, Shaobo, Yaping Chen, Jiafeng Wu, and Zilong Zhu. 2018. “Thermodynamic Analysis on a Modified Kalina Cycle with Parallel Cogeneration of Power and Refrigeration.” Energy Conversion and Management 163:1–12. https://doi.org/10.1016/j.enconman.2018.02.035.
- Zhang, Xinxin, Maogang He, and Ying Zhang. 2012. “A Review of Research on the Kalina Cycle.” Renewable and Sustainable Energy Reviews 16 (7): 5309–5318. https://doi.org/10.1016/j.rser.2012.05.040.
- Zhang, Na, and Noam Lior. 2007. “Development of a Novel Combined Absorption Cycle for Power Generation and refrigeration.”
- Zheng, Danxing, Bin Chen, Yun Qi, and Hongguang Jin. 2006. “Thermodynamic Analysis of a Novel Absorption Power/Cooling Combined-cycle.” Applied Energy 83 (4): 311–323. https://doi.org/10.1016/j.apenergy.2005.02.006.
- Zhu, Zilong, Zhi Zhang, Yaping Chen, and Jiafeng Wu. 2016. “Parameter Optimization of Dual-pressure Vaporization Kalina Cycle with Second Evaporator Parallel to Economizer.” Energy 112:420–429. https://doi.org/10.1016/j.energy.2016.06.108.