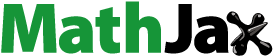
ABSTRACT
Thermal storage systems are needed to overcome solar intermittency and make this energy source more flexible and competitive for concentrated solar power plants. Single-tank sensible heat storage using both fluids and materials is a promising option for reducing storage costs and promoting the development of concentrated solar power. This work is a thorough review on the parameters influencing the performance of a dual-medium thermocline storage system for concentrated solar power plants. Thus, indicators such as efficiency, utilisation rate, thermocline thickness and energy efficiency of the storage system are presented in order to quantify the performance of the system. In addition, the impact of geometric and operating parameters on the performance of the thermocline storage system has been discussed.
Nomenclature
Table
1. Introduction
As a result of technological progress, energy demand is increasing exponentially. Up still now, the energy demand is based on fossil resources. Fossil resources exploration and utilisation can increase environmental pollution. Solar energy is considered one of the sustainable energy sources to reduce greenhouse gas emissions from the use of fossil fuels (Gil et al. Citation2010). Solar thermal technology, such as concentrated solar power (CSP), is a promising solution for producing clean energy (Tiskatine et al. Citation2017). This is a technology based on converting direct solar radiation into heat to produce electricity. According to the operating principle of the CSP, mirrors concentrate the direct sunlight on a small area called the receiver where a heat transfer fluid is heated to a high temperature. The heated heat transfer fluid transfers the heat to the steam generator which, thanks to an alternator, produces electricity. The intermittency of solar radiation has a direct impact on the process and hinders the large-scale industrial commercialisation of this technology (Reddy and Pradeep Citation2021). Therefore, the use of a Thermal Energy Storage (TES) system in this technology compensates for the unpredictability of solar energy and ensures the continuity of steam generation for electricity production. The TES system not only minimises the unpredictable effect of solar insolation by distributing the power plant’s output but also reduces the cost of electricity (Bradshaw and Siegel Citation2016; Jemmal, Zari, and Maaroufi Citation2016). TES is composed of three main elements (Hoffmann Citation2015): a storage principle, an energy transfer mechanism and a containment system. The storage principle is based on the storage of thermal energy in the form of latent, sensible and thermochemical heat. Transfer mechanism plays the role of charging and discharging the heat from the storage system in an appropriate manner. Containment system holds the two components together and allows them to be isolated from the outside. Sensible heat storage is the most mastered and mature in terms of complexity and feasibility (Rodrigues and De Lemos Citation2020; Erregueragui, Boutammachte, and Bouatem Citation2016). The technology for storing thermal energy in the form of sensible heat has two types of configurations: two-tank configuration and single-tank configuration. The two-tank system performs better than the single-tank system, but has huge costs that can make CSP less accessible to developing countries (Cocco and Serra Citation2015). One-tank configuration is 35% less expensive than the two-tank configuration (Nandi, Bandyopadhyay, and Banerjee Citation2018). The key operating characteristic of single-tank storage is the principle of stratified buoyancy, which provides thermal separation (Calderón-Vásquez et al. Citation2021). However, the stratification feature added to the non-uniform flows and the imperfect heat exchange between fluid and solid in the single tank system decrease the performance and lower the efficiency of the storage system. Thus, numerical and experimental simulation studies were carried out on the single-tank storage system to find parameters that could improve the efficiency of thermocline storage.
This work aims to review the main characteristics and design parameters of the packed thermal storage system that generate major effects on the efficiency of the thermocline through previous numerical and experimental work. The aim is to provide, through the literature, the optimum conditions for increasing the efficiency of the single-tank sensible heat storage system that will make CSP a reliable technology.
2. Single-tank sensible heat storage system
The enormous costs associated with the huge quantities of thermal fluids involved in two-tank systems led researchers to develop the one-tank system. This promising approach, tested in the 1980s on a pilot scale, allows the cost of the system to be reduced by combining the hot tank and the cold tank into a single tank (Angelini, Lucchini, and Manzolini Citation2014; Medrano et al. Citation2010). The principle of this system is such that during charging a hot fluid such as molten salt or thermal oil is pumped into the top of the tank in order to expel the cold fluid which is sucked downwards, and during the discharge the cold fluid passes through the bottom of the system and drives out the hot fluid (Yang et al. Citation2016). The cohabitation of hot and cold fluid creates a thermal gradient within the system and is ideally stabilised and preserved by buoyancy effects (Qin et al. Citation2012; Bayón and Rojas Citation2014). This system is called thermocline storage because of the thermal gradient that develops within the system (Yang et al. Citation2016). During charging or discharging, the thermocline created rises or falls until it exits the vessel as shown in (Xu et al. Citation2012b).
When only fluid is used as a storage medium in the system then it is called Single Medium Thermocline (SMT) (Boubou et al. Citation2021). However, thermal convection within the single-tank system prevents ideal temperature stratification from being achieved. A solid packing material can therefore be inserted to maintain the gradient and reduce natural convection in the fluid. The use of solid material and fluid in a single-tank storage system is called Dual Media Thermocline (DMT) (Boubou et al. Citation2021). shows the difference between the DMT system and the SMT system. The DMT system is economically viable compared to the SMT system because part of the fluid is substituted by solid materials. In addition, the addition of solid materials increases the theoretical storage energy density by about 50% compared to a two-tank storage system (Cocco and Serra Citation2015; Calderón-Vásquez et al. Citation2021; Galione et al. Citation2015). With low-cost solid materials, it would be possible to reduce the overall capital cost of the thermal storage system (Calderón-Vásquez et al. Citation2021). Fasquelle et al. (Citation2018a) showed that the Thermocline single-tank system reduced annual electricity production at maximum storage capacity by only 3.4%, but expenses are reduced by an average of 30% mainly due to the reduced amount used in the single-tank system. These findings are similar to those of Brosseau et al. (Citation2005) who reported a 10-35% reduction in costs, depending on the method and storage capacity considered.
Figure 2. Difference between SMT and DMT (Fasquelle Citation2017).

Despite economic gain obtained with the single-tank system, several technical problems continue to limit the large-scale use of the compact thermocline bed system. For instance, the thermocline transition zone tends to gradually widen or deteriorate after multiple charge–discharge cycles. In order to resolve these difficulties, it is essential to develop a comprehensive understanding of the system’s functioning and the intrinsically interconnected heat and matter transfer mechanisms.
3. Storage materials used in sensible single-tank storage
The efficiency of sensible heat storage is closely linked to the choice of materials. It is important not only that the heat transfer fluid and the solid material both have a high operating temperature to increase the efficiency of the cycle, but also that they are chemically stable enough to ensure the reversibility of the storage process over a large number of cycles (Gil et al. Citation2010; Medrano et al. Citation2010). In addition, solid materials must be available in large quantities so that the storage system is less costly. The heat transfer fluid and storage materials selected must also be long-lasting, have a negligible impact on the environment and be non-toxic and non-flammable (Fasquelle Citation2017). Other criteria mentioned in (Hoffmann et al. Citation2018) are also necessary for the choice of materials.
3.1. Heat transfer fluids
There are two types of heat transfer fluid: liquids and gases. The liquid heat transfer fluids generally used in CSPs are molten salts, thermal oils and water. Air and other gases are considered to be gaseous heat transfer fluids. presents some of the physical properties of heat transfer fluids. Fluids are selected according to the type of collectors used in the installation. In fact, molten salts and air with a high temperature range under atmospheric pressure are usually used in tower systems, whereas thermal oils used in parabolic trough or Fresnel collector system (Vannerem Citation2022).
Table 1. Thermophysical properties of fluid for sensible heat storage.
Water is the most abundant liquid heat transfer fluid and has a low cost (Mawire and McPherson Citation2009). It has a large storage capacity compared with other heat transfer fluids. Its use as a thermal fluid increases the efficiency and reduces the cost of electricity production (Modi and Haglind Citation2014). However, it cannot exceed 100°C at atmospheric pressure, beyond which it is transformed into steam. To use it in applications with temperatures above 100°C, the water has to be pressurised, a process that increases costs.
The thermal oils used in CSPs are mineral oils, silicone oils and synthetic oils. These oils are thermally stable up to 400°C and have a low viscosity (Modi and Haglind Citation2014; Tian and Zhao Citation2013). Their disadvantage is that they are relatively expensive and flammable, and their thermal properties deteriorate with heat, which reduces their lifetime.
Because of their storage capacity and high temperature range, molten salts are widely used as heat transfer fluids. Compared to synthetic oils, they are less expensive, non-toxic and non-flammable, which makes them the preferred choice (Vignarooban et al. Citation2015). In addition, the properties of molten salts are thermodynamically stable and can be accurately estimated using correlations confirmed by experiments (Wang, Yang, and Duan Citation2015). However, they are corrosive and the risk of pipe damage is high due freezing.
Air is the most abundant gaseous heat transfer fluid (Zunft et al. Citation2011). It is free, safe, non-flammable and can be used at atmospheric pressure, which reduces installation costs and safety risks (Liu et al. Citation2014). However, the thermal properties of air are very low compared with those of other fluids, which means that large surface areas and high flow rates are required to facilitate heat exchange.
3.2. Filling materials
presents the thermo physical properties of some solid materials. Natural rocks (quartzite, basalt, etc.) are commonly used in dual media thermocline storage systems because of their availability in large quantities and their low cost. They also have better thermal properties and are thermally stable. However, the irregular shape and size of these natural rocks is a disadvantage that reduces the performance of TES (Boubou et al. Citation2021).
Table 2. Thermophysical properties of packing materials for dual media thermocline sensible heat storage.
A number of studies have shown the potential of industrial waste for the production of ceramics as thermal storage materials whose shape can be controlled (Hoffmann Citation2015; Py et al. Citation2011; Calvet et al. Citation2015; Gutierrez et al. Citation2016; Calvet et al. Citation2013; Py and Olives Citation2016). Solid materials from industrial waste have better storage capacity by volume, but their price is prohibitive and their availability is not guaranteed. Recently, Bagré et al. have shown that ceramic balls made from sand, bottom ash and clay can be used as fillers in TES (Bagre Citation2021). These materials are locally available, less expensive and have interesting properties that can improve the performance of storage systems.
4. Parameters affecting the performance of the thermocline dual media storage system
4.1. Basic mathematical equations in the dual media storage system
There are several mathematical models for predicting the performance of thermocline dual media storage systems (Nandi, Bandyopadhyay, and Banerjee Citation2018; Esence et al. Citation2017; Sassine, Donzé, and Bruch Citation2017; Capocelli et al. Citation2019; Arpino et al. Citation2014; Fernández-torrijos, Sobrino, and Almendros-ibáñez Citation2017; Bagre et al. Citation2023; Mira-Hernández, Flueckiger, and Garimella Citation2014). Ismail and Stuginsky, presented and summarised many models of regenerative thermal storage (Ismail and Stuginsky Citation1999). Similarly, Esence et al. (Citation2017) and Calderón-Vásquez et al. (Citation2021) have reviewed the different numerical models used because of the assumptions made and the degree of simplification involved. Among many others, we have the Schumann model (eq 1 and eq2), which is the oldest and most common heat transfer model for lined beds (Ismail and Stuginsky Citation1999).
(1)
(1)
(2)
(2) Most of the mathematical models developed to predict the performances of DMT are based on Schumann model (Calderón-Vásquez et al. Citation2021; Esence et al. Citation2017).
4.2. Performance indicators for the thermocline dual media storage system
To evaluate the performance of a storage system, performance indicators are required. They vary according to some studies (Haller et al. Citation2009; Ibrahim and Marc Citation2011). Haller et al. (Citation2009) reviewed the various approaches (performance indicators) for evaluating the performance of a thermocline storage system. Commonly used indicators are energy and exergy efficiency, utilisation rate and thermocline thickness. In addition, the times at which charging and discharging are interrupted are also criteria to be taken into account when assessing storage performance.
The energy efficiency, is defined as the ratio between the energy restored during discharge
and the energy stored during charge
. This indicator is widely used in the literature to assess storage system performance.
(3)
(3) Exergy efficiency, also known as the efficiency rate of the second law, is a measure used to determine the efficiency of a process, taking into account the second law of thermodynamics.
According to Ibrahim and Marc (Citation2011), exergy analysis based on the second principle of thermodynamic makes it possible to detect the origins and extent of deficiencies in cycle processes and to specify that energy can undergo a change in quality that leads it to a state of total equilibrium with the environment, where it loses all usefulness for carrying out tasks. For TES, the exergy evaluation identifies the maximum capacity linked to the incoming thermal energy. Many studies have used exergy efficiency to assess the performance of storage systems (Haller et al. Citation2009; Rosen, Tang, and Dincer Citation2004; Rosen Citation2001; Rosen and Dincer Citation1997; Rosen Citation2012; Bejan Citation1978; Rosen Citation1999). In general, exergy efficiency is defined by comparing the exergy of the fluid leaving during discharge with that of the fluid entering during charging, as shown in equation 4 (Vannerem Citation2022):
(4)
(4)
(5)
(5) The utilisation rate is a criterion that is independent of the charging or discharging process. It is used to assess charging and discharging in isolation. For the charge, it is defined as the ratio (equations 6 and 7) (Vannerem Citation2022) between the quantity of energy actually stored in the reservoir
at the end of the process and the maximum quantity of energy
which could be stored if all the components (fluids, solids, wall) of the storage system were brought to the high temperature considered from the initial low temperature (equation 6 and 7).
(6)
(6)
(7)
(7) This expression in equation 7 can be written simply as equation 8 (Vannerem Citation2022).
(8)
(8) The discharge utilisation rate
(equation 9) is evaluated by comparing the energy extracted from the storage during the discharge process with the maximum energy that could be fully extracted if the storage were perfectly cooled to the lowest temperature. Its expression is similar to that of the charge utilisation rate.
(9)
(9) Two necessary conditions must be met in order to assess the cycle utilisation rate. Firstly, the temperature
at the start of the charge must be identical to the low temperature
. Secondly, the final charge temperature
must match the initial discharge profile
. These two conditions are only met for numerical studies. The cycle utilisation rate is then obtained by multiplying the charge and discharge utilisation rates according to equation 10 and 11 (Vannerem Citation2022).
(10)
(10)
(11)
(11)
By definition, the cycle utilisation rate is the comparison between the amount of energy recovered during discharge and the maximum amount of energy available between the initial charge temperature and the maximum discharge temperature.
The thickness of the thermocline zone is also a criterion used to assess performance, in particular the stratification zone in the reservoir, in accordance with the constraint of conditions in a specific temperature range before reaching the top or bottom of the reservoir (Xie and Xie Citation2022). This criterion is mainly defined as the temperature range between the charging temperature threshold and the discharging temperature threshold illustrated in equations 12 and 13 respectively (Fasquelle et al. Citation2018b).
(12)
(12)
(13)
(13) The choice of value is based on experimental data and the choice of temperatures upstream (solar panel) and downstream (power block) of the storage system (Fasquelle Citation2017; Vannerem Citation2022). This criterion has a significant impact on system performance. The dimensionless thickness of the thermocline zone is defined by equation 14.
(14)
(14) according to several studies, the percentage of the temperature threshold n% can be taken as 20% (Fasquelle et al. Citation2018a, Citation2018b; Hoffmann et al. Citation2017; Hoffmann et al. Citation2016; Xu et al. Citation2012a, Citation2012b). So equations 12 and 13 can be written as equations 15 and 16.
(15)
(15)
(16)
(16) Influence on performance indicators by geometric parameters, operating parameters and thermo-physical properties through numerical and experimental work according to the literature will be the subject of the next section.
4.3. Effect of geometric and operating parameters on the performance of dual media thermocline storage
Dual media thermocline sensitive heat storage is more economical, and many numerical and experimental studies have been carried out to improve its performance. below presents some numerical and experimental studies on the influence of geometric and operating parameters on the performance of storage systems and some of the results obtained.
Table 3. Studies of the impact of geometrical and operating factors on the sensitive heat dual media thermocline system.
4.3.1. Effect of geometrical parameters
4.3.1.1. Effect of tank configuration
The thermocline storage system can be designed in cylindrical, rectangular and truncated cone shapes according to the literature ().
Figure 3. Tank configuration, (a): cylindrical tank (Xie Citation2022), (b): conical tank (Zanganeh et al. Citation2015), (c): rectangular tank (Touzo et al. Citation2020).

Firstly, the cylindrical shape is the preferred configuration for storage tanks, as it effectively withstands the pressure exerted on the walls while eliminating edge effects and allowing the flow to be distributed evenly (Melanson and Dixon Citation1985). Moreover, the vertical layout of this type of configuration has the advantage of reducing the impact of natural convection by minimising the exchange of material between the cold lower region and the hot upper region. In addition, this configuration not only reduces heat loss but also saves some of the mechanical difficulties involved in the installation (Melanson and Dixon Citation1985).
Secondly, a conical tank can be used to reduce the effects of thermal ratcheting (Geissbühler et al. Citation2018). Thus, Zanganeh et al. (Citation2012) proposed a conical tank which reduces the ratio between the surface area and the volume of the upper part of the tank, the aim of which is to limit the mechanical stresses and heat losses of the hot thermocline storage part. Sassine et al. (Citation2018) have also shown that the use of this type of tank would lead to a 20% reduction in the mechanical stress exerted on surfaces compared to a cylindrical tank. However, the cost of this type of tank could be higher, and its construction more complex.
Finally, the rectangular shape of the storage tank in a vertical position is also mentioned in several studies (Odenthal et al. Citation2020a, Citation2020b; Nicolas et al. Citation2019; Touzo et al. Citation2020). The experimental and numerical study on a horizontal rectangular reservoir by Touzo et al. showed that no channelling effect and a moderate radial thermal gradient were detected, despite the reservoir having a horizontal geometry (Touzo et al. Citation2020). With a prototype horizontal air/bauxite thermocline tank, Lopez et al. also observed no thermal gradient or thermal stratification in the direction of fluid flow (Nicolas et al. Citation2019). They proved that the temperatures were virtually equal for a given axial coordinate. The horizontal position has a benefit associated with thermo mechanical consequences (Touzo Citation2021). According to the literature, the packing bed compresses during temperature variations, causing an increase in stress on the walls of the tank and this can cause damage to the tank and the materials that make up the bed (Esence, Desrues, et al. Citation2019). Reducing the vertical dimension of the tank in relation to its horizontal dimension naturally reduces the pressure on the wall caused by the settling of particles, which reduces the risk of being exposed to mechanical stress. the horizontal layout of the rectangular tank offers many advantages for its design and installation on a site, although it can provide poor thermal performance (Touzo Citation2021).
On a laboratory and industrial scale, the effect of the shape and dimensions of the tank on the performance of the storage system is quite remarkable. The cylindrical shape with the vertical position performs best in terms of discharge efficiency and energy storage capacity. This shape is therefore the most effective, although the truncated cone shape has advantages in terms of reducing mechanical stress.
4.3.1.2. Effect of tank height and size
The choice of reservoir height and diameter influences the performance of the storage system, according to these studies (Xu et al. Citation2012a; Yang and Garimella Citation2013; Cárdenas et al. Citation2018; Odenthal et al. Citation2020b; Nicolas et al. Citation2019). Chao et al. in 2012, numerically studied the effect of the height and diameter of a cylindrical storage system using molten salt and quartzite. The results showed that the energy storage capacity increased proportionally by 2.33 times as the height of the reservoir increased from 6 to 14 m. The system can therefore take longer to discharge, as shown in (a). The effective discharge time increases from 1.88 to 4.57 hours, or 2.43 times (Xu et al. Citation2012a). (b) confirms the improvement in discharge time with the increase in reservoir height, favoured by the thinness of the thermocline thickness obtained with the increase in this parameter. In the literature tank or
ratios are generally calculated for the cylindrical configuration in order to assess the storage performance (thickness of the thermocline). Some studies have shown that the thickness of the thermocline becomes thinner as the
aspect ratio increases (Xu et al. Citation2012a; Flueckiger and Garimella Citation2012; Chang et al. Citation2015). The numerical study by Yang et al. also confirmed the positive influence of increasing this aspect ratio (Yang and Garimella Citation2013). Zanganeh et al. (Citation2015) have shown that the lower
aspect ratio gives not only higher output temperatures but also the best overall efficiency for a cone-shaped storage system. For the different aspect ratios
and
, an increase in one translates into a decrease in the other, so the results of these previous studies corroborate each other. It is assumed that a high reservoir height improves the efficiency of the thermal storage system on a laboratory or pilot scale. However, high-rise tanks present structural challenges that make them impractical as well as compact, leaving them vulnerable to heat loss. This is why the height limit for industrial-scale foundations is twelve metres (Vannerem Citation2022). To achieve this, it is essential to find a compromise that minimises the proportional dimension of the thermocline in the high-bay tank, while taking into account the practical aspects. On an industrial scale, limiting the maximum height of a reservoir means increasing the diameter of the reservoir to increase storage capacity, to the detriment of the aspect ratio (Vannerem Citation2022).
Figure 4. Variations in the molten salt temperature at the outlet and the thermocline thickness with the discharging time using different tank heights (a). Variations in normalised thermocline thickness with the discharging time using different tank heights (b) (Xu et al. Citation2012a).

In addition, Cárdenas et al. (Citation2018) numerically studied the effect of geometrical parameters on the energy efficiency of an industrial-scale dual-media thermocline storage system and the results revealed that an aspect ratio between 0.5 and 0.8 improves the discharge efficiency.
The choice of minimum tank height and diameter will depend on the scale of applications. At laboratory or pilot scale, aspect ratios in excess of 1 up to 4 can be taken to obtain the best storage and destorage efficiencies. However, on an industrial scale, the aspect ratio would be less than unity to compensate for mechanical constraints.
4.3.1.3. Effect of particle size
The size and shape of the packing materials also influence the performance of the thermocline storage system. Most studies taking into account the spherical shape of solid materials have observed that smaller diameter spheres increase the efficiency of the storage system (Xu et al. Citation2013; Yang and Garimella Citation2013; Abdulla and Reddy Citation2017; Van Lew et al. Citation2011). In fact, a small size simultaneously increases the contact surface and the rate of interstitial heat transfer between the thermal fluid and the filler material. This is confirmed by the work of Van Lew et al. (Citation2011) and Yang and Garimella (Citation2010) who state that solids with very small diameters are effective in improving thermal stratification by increasing the total exchange surface between the fluid and the solid, while reducing the adimensional Biot number of the solids. D. Schlipf et al. studied a thermocline system using air as a heat transfer fluid and quartzite, sand and basalt as packing materials (Schlipf et al. Citation2015). Theoretical and experimental results () have shown that materials with particularly small grains offer very good heat storage potential thanks to the rapid diffusion of temperature in the material, which favours the generation of a thermocline with a very small thickness. Laube et al. (Citation2020) have carried out a numerical study on a laboratory and industrial scale of a high-temperature storage concept using several fluids (Solar salts, liquid metals) as thermal fluids and quartzite as fillers materials They observed that, on an industrial scale, particle diameter did not significantly affect the efficiency of the discharge. On the other hand, a clearer sensitivity to particle size was observed at laboratory scale, showing that small-diameter particles are more preferred. In addition, on an industrial scale, Bruno Cárdenas et al. showed numerically that particles with a diameter of 3.7 mm help to reduce the size of the thermocline and increase energy efficiency by more than 98.24% (Cárdenas et al. Citation2018).
Figure 5. (a): Temperature behaviour trough the packed-bed for 530 °C inlet temperature (basalt gravel with 4 mm, air flow: 25 Nm³/h); (b): Comparison of the temperature behaviour through the packed-bed (quartz gravel with 2 mm, silica sand < 1 mm, air flow: 35 Nm³/h) (Schlipf et al. Citation2015).

It is agreed that small-grained solid materials improve the performance of the thermocline storage system. However, very small particle sizes such as sand can cause substantial pressure drop and lower system efficiency (Cárdenas et al. Citation2019). In addition, Bruch, Fourmigué, and Couturier (Citation2014) analysed the flow of thermal oil through a 3 mm sand substrate and a 3 cm rock substrate in a pilot-scale storage system. The results showed that the smaller particles facilitate the diffusion of the fluid flow to increase the heat transfer surface area. However, the small size of the particles can cause a more significant increase in pressure losses (Bruch, Fourmigue, Couturier Citation2014). The size of the particle is a parameter to be taken into account when designing the thermocline storage system. It is accepted that, whatever the filling material, a small diameter particle (of the order of a millimetre for sands and a few centimetres for rocks) is always desirable to improve the performance of the storage system on a laboratory, pilot or industrial scale.
4.3.1.4. Effect of porosity
Filling materials can be arranged in bulk or not. Loose fill is characterised by a poorly organised arrangement of irregular solids, whereas structured fill is a well-organised arrangement of regular solids. The packing bed is mainly defined by porosity, which represents the percentage of the bed volume that is not filled by solid particles (Esence et al. Citation2017), i.e. the volume occupied by the fluid. Bulk packing beds composed of spherical solids of uniform size generally have a porosity of between 0.3 and 0.4 (Faas et al. Citation1986). According to some studies found in the literature, a porosity of 0.25 can be obtained by mixing solids of two different sizes (Bruch, Fourmigue, Couturier Citation2014; Raymon and Robert Citation1997; Pacheco, Showalter, and Kolb Citation2001; Bagre et al. Citation2023). Low porosity reduces costs in solid/liquid systems, where the liquid used is often more expensive than the solids, whereas in solid/gas systems, it increases storage density because the solids generally have a higher heat capacity by volume than the fluid. Jon T. Van Lew et al. (Touzo et al. Citation2020) studied numerically the behaviour of a storage system composed of Therminol VP-1 as a thermal oil and granites as packing materials with a storage capacity greater than that of the fluid (Van Lew et al. Citation2011). They observed that as porosity increases storage efficiency falls as shown in . However, increasing the porosity above 0.7 leads to an increase in storage efficiency although a void fraction of 0.7 is almost unachievable from a theoretical point of view. This phenomenon could be due to the fact that above a certain porosity, the dual media system behaves like a single media system composed of a fluid with a high storage capacity.
Figure 6. Effect of the pack bed void fraction on the thermal storage efficiency (track down) (Van Lew et al. Citation2011).

In contrast, Xu et al. (Citation2012a) have shown numerically that low porosity of a storage system composed of molten salt with a filler material with a lower heat capacity by volume than the salt results in a short discharge time and a large thickness of the thermocline, reflecting low storage efficiency ().
Figure 7. Variations in the molten salt temperature at the outlet and the thermocline thickness with the discharging time using different porosities (Xu et al. Citation2012a).

Recently, Bagré et al in 2023 also numerically studied the impact of porosity on the performance of a thermocline storage system based on jatropha oil and ceramic balls (Cárdenas et al. Citation2019). They showed that low porosity increases the efficiency of the discharge, favoured by the generation of a thin thermocline (Zhao et al. Citation2018).
In the light of these studies, it is clear that low porosity considerably reduces the cost of storage, but its effect on the efficiency of the dual media thermocline storage system is directly linked to the heat capacity of the packing material used in relation to that of the fluid. This parameter is taken into account when designing storage systems on a laboratory, pilot or industrial scale, taking into account the heat capacities of the fluid or solid materials.
4.3.1.5. Effect of tank wall and insulation
The temperature ranges used and the size of the storage system selected determine the type of material selected for the tank wall (Xie and Xie Citation2022). Most of the materials used in the design of storage tanks are metals such as carbon steel, given their high strength and ability to withstand high pressures in high-temperature applications (Baoshan et al. Citation2022). These metals have a high thermal conductivity, often higher than that of the fluid. The use of such a material can lead to high heat loss due to its high thermal conductivity. Baoshan et al. (Citation2022) and Motte, Bugler-Lamb, and Falcoz (Citation2015) evaluated the impact of wall material on the performance of a lined thermocline storage tank using three numerical models with two experimental set-ups, one at laboratory scale and the other at pilot scale. The wall of the pilot-scale tank is steel and that of the laboratory-scale tank is polycarbonate. Analyses show that whatever the material, a thin wall has a reduced effect on energy and exergy performance. In addition, the same study showed that better insulation considerably reduces the thickness of the thermocline by around 20% and increases energy efficiency from 5% to 7% and the capacity ratio from 3% to 5% (Motte, Bugler-Lamb, and Falcoz Citation2015). This is why a judicious choice of tank construction materials and adequate tank insulation are necessary, especially for laboratory-scale storage systems. It is desirable to take into account the effect of the wall during numerical studies to better predict the performance of storage systems.
4.3.2. Effect of operating parameters
Numerous numerical and experimental studies have investigated the effects of operating parameters on the performance of the thermocline storage system.
4.3.2.1. Effect of inlet temperature and temperature difference
The temperature difference between the hot and cold storage sources is a parameter that seems to influence the performance of thermocline storage systems according to the literature. A number of numerical and experimental studies have investigated the effect of varying the temperature difference on the operation of the thermal storage system. Studies by Bruch et al. Yang and Garimella (Citation2010), Flueckiger and Garimella (Citation2012), Bayón and Rojas (Citation2013), Xu et al. (Citation2012a), have shown that varying the temperature difference between the hot and cold source does not significantly influence the thickness of the thermocline ((a)), nor the temperature profiles at the end of the charging and discharging stages, nor the utilisation rate ((b)).
Figure 8. Effect of inlet temperature on thermocline thickness and discharge time (a) (Xu et al. Citation2012a) and on temperature profiles during a thermal cycle (b) (Bayón and Rojas Citation2013).

Contrary to previous studies, Yin et al., through a numerical study, observed that a charging with the lowest temperature variation had the highest charging efficiency (Yin et al. Citation2017). In addition, Abdulla and Reddy have numerically investigated the effect of a few geometrical and operating parameters on the performance of the TES system by observing the evolution of the thermocline and discharge efficiency (Abdulla and Reddy Citation2017). They found that the operating temperature difference is a crucial factor impacting the thermal performance of the TES system, since the overall efficiency decreases by 12% when the temperature difference increases from 50 K to 150 K. This can be seen in (b), which shows that the discharge efficiency is high at low operating temperatures and tends to decrease as the temperature difference increases. This naturally explains the increase in the thickness of the thermocline as the temperature difference increases ((a)) (Abdulla and Reddy Citation2017).
Figure 9. (a): Variation in thermocline thickness with different temperature ranges, (b): Variation of discharge efficiency with evolution temperature ranges (Abdulla and Reddy Citation2017).

In general, from study to another, there is some controversy about the effect of inlet temperature or operating temperature deviation on the performance of the dual media thermocline storage system. In view of the lack of consensus on the influence of this parameter, Vannerem Ségolem in his thesis in 2022 suggests that these predecessors may have omitted some important assumptions from the calculations of certain performance indicators, which led to a contradiction in their results. By reformulating the hypotheses and concepts of a number of indicators and using experimental and numerical studies, Vanerem Ségolem showed that the temperature difference had no major impact on the overall performance of the TES.
Although some studies agree that temperature has a limited effect on storage system performance, there is still no consensus on the overall trend in the effect of temperature difference, nor on the physical explanation for this phenomenon. The incomprehension of the impact of this parameter is maybe due to the use of different fluids with thermo-physical properties varying with temperature during the tests. Experimental and numerical studies are therefore still needed to clarify the impact of this parameter on the storage system performance. The variation of HTF properties as function of temperature could be a reason of these differences in results.
4.3.2.2. Effect of fluid velocity
The velocity of the fluid in the reservoir during charging or discharging is a parameter that has an impact on the performance of the thermal energy storage system. Indeed, a few numerical and experimental studies have shown its influence on performance indicators. Rocío Bayón and Esther Rojas have shown through a simulation study based on a single-phase model that increasing the fluid inlet velocity leads to a decrease in thermocline thickness and an increase in discharge efficiency (Bayón and Rojas Citation2013). Bruch et al. experimentally studied the influence of fluid velocity by assessing its impact on the utilisation rate and energy efficiency (Bruch et al. Citation2017). The results showed that fluid velocity had a non-significant effect on the utilisation rate, but energy efficiency increased as velocity increased. In opposition, the study by Abdulla and Reddy based on a two-dimensional biphasic model shows that an increase in fluid velocity leads to a considerable drop in discharge efficiency (Abdulla and Reddy Citation2017). Similarly, F. Motte et al. (Karim Citation2011), Yang and Garimella (Citation2010), Yin et al. (Citation2017) also observed a decrease in discharge efficiency with increasing fluid velocity.
These previous studies show a contradiction in results, revealing a lack of understanding of the phenomenon induced by fluid velocity. The difference in results is due to differences in the underlying assumptions of the numerical models, differences in the definitions of the performance indicators, and differences in the simulated operating conditions used by the authors.
To resolve this contradiction, some numerical and experimental investigations predict the existence of an optimal speed that improves performance (Fernández-torrijos, Sobrino, and Almendros-ibáñez Citation2017). Indeed, Hoffmann et al. (Citation2017) conducted an experimental study on a laboratory-scale storage system composed of quartzite and Rapeseed oil, with the aim of understanding the impact of fluid mass flow rate on system performance. The experimental and numerical results obtained show an optimum mass flow rate ((a)) (Hoffmann et al. Citation2017). In addition, Esence, Brunch, et al. (Citation2019) numerically evaluate the effect of fluid velocity on the utilisation rate for different heat loss coefficients ((b)). They noted a reduction in the utilisation rate at low fluid velocity, even for the adiabatic case, and showed that the ideal velocity relates to an equilibrium not only with stratification but also with heat losses.
Figure 10. (a): influence of mass flow rate on discharge efficiency, (b): evolution of utilisation rate with fluid velocity for different heat loss coefficients.

Recently, the thesis work of Vannerem (Citation2022), which was largely based on the study of the influence of operating parameters on the performance of a thermocline storage system, also highlighted the existence of an optimum speed depending on the chosen performance indicator (Vannerem, Neveu, and Falcoz Citation2021). Vannerem Ségolène observes that the cycle utilisation rate peaks at an interstitial velocity of 4.10−3, while the exergy efficiency is highest at a velocity of 6.7*10−3. According to the author, the difference in these values is due to heat loss, the thermal conductivity of the solid and the fluid and thermal convection between the fluid and the solid.
Studies on the effect of fluid velocity show that this parameter should be taken into account, despite the difficulty of reaching a clear consensus on its impact on system performance. Several experimental studies followed by meticulous numerical investigations with well-defined hypotheses will be needed to clarify the effect of fluid velocity on the performance of a storage system.
4.3.2.3. Effect of fluid distribution
The method of distributing the fluid can disrupt its flow within a thermocline storage system and affect its performance.
On one side, fluid distribution is extensively studied numerically or experimentally for single medium thermocline storage systems in the studies (Mawire and McPherson Citation2009; Li et al. Citation2013; Nellis and Klein Citation1977; Wildin Citation1996; García Mari et al. Citation2013; Li et al. Citation2014; Lou, Fan, and Luo Citation2020; Shah and Furbo Citation2003; Farmahini-Farahani Citation2012). The impact of this parameter is much more noticeable for single media thermocline storage systems using water as the storage medium (Li et al. Citation2013; Shah and Furbo Citation2003; Eames and Norton Citation1998; Hasan and Theeb Citation2021; Lou, Fan, and Luo Citation2020) for this type of configuration, the influence of fluid distribution is directly linked to the geometry or position of the diffuser used (García Mari et al. Citation2013; Farmahini-Farahani Citation2012). Using a single media thermocline storage system, Li et al. (Citation2014) showed experimentally that a slot-type diffuser geometry improves performance over single pipe and shower-type diffusers. Precisely as shown in , discharge efficiency drops from 71% to 25% for the shower-shaped diffuser, while it decreases from 90% to 64% for the slot configuration when the volume flow rate is multiplied by three, demonstrating the robustness of the slot diffuser compared with the others (Li et al. Citation2014). Wildin also investigated the effect of diffuser height and inlet Reynolds number on thermocline generation during loading for a water storage system (Wildin Citation1996). He found that thermocline generation is effective using a diffuser of reduced height.
Figure 11. Effective discharging effective of the tanks with different inlets(track down) (Li et al. Citation2014).

On the other hand, the effect of fluid distribution on the performance of a dual-media thermocline storage system has been less studied experimentally and much more numerically (Mira-Hernández, Flueckiger, and Garimella Citation2014; Wang, Yang, and Duan Citation2015; Bellenot et al. Citation2019; Vannerem Citation2022). Wang, Yang, and Duan (Citation2015) numerically investigate the effect of fluid distribution on the performance of a DMT system using annular diffusers with variable injection area and constant fluid flow rate. they achieved that despite considerable obstruction (80% of the surface area) at the entrance, the flow distribution has only a moderate impact on exploitable energy production (<3% variation). They also concluded that an axial distribution offers better performance than a uniform distribution. Based on a constant flow rate assumption, it is difficult to make differentiate difference between the impact of fluid velocity and that of the diffusers on the TES performance. The thesis work of Bellenot (Citation2021), based on the influence of fluid distribution on the thermo-hydraulic behaviour of a dual-media single-tank thermal storage reservoir, aimed to overcome this problem. An experimental series of tests on the STONE installation using different fluid injection and collection mechanisms () on a pre-industrial-sized thermal reservoir showed that there is no significant distinction between the various injection techniques during charging time. However, the amount of energy recovered during discharges differs from one distributor to another, as axial collection does not recover as much energy as radial collection. This difference in recovery energy during discharge is smaller, so the influence of the distributors is not so important. Gregoire Bellenot confirmed the conclusions from the experimental study by means of a multidimensional numerical study (Bellenot et al. Citation2019).
Figure 12. Schematic of the STONE system with the different injection modes (Bellenot et al. Citation2019).

To improve the understanding of the impact of fluid distribution, Ségolène Vannerem has undertaken numerical and experimental studies on the effect of this parameter (Vannerem Citation2022). By experimentally evaluating the influence of fluid velocity on storage behaviour with three types of diffusers (peripheral, central and uniform), he observed that no diffuser affected the behaviour of the storage system. According to the author, these results are due to the packing materials, which naturally act as a distributor and homogenise the velocity field, whatever the injection method used ().
Figure 13. (a) Evolution of the discharge utilisation rate with flow rate for the three distributors tested (b) Charge, discharge and cycle utilisation rates for the three distributors tested (Vannerem Citation2022).

From all the above, single medium thermocline storage systems are greatly affected by the geometry of the diffuser. However, the effect of fluid distribution is found to be insignificant on the performance of dual media thermocline systems. The packing homogenises fluid circulation for fluid collection and injection. DMT is therefore ideal for use in industrial-scale power plants.
4.3.2.4. Cycling operation effect
The cycling process involves alternating charges and discharges. Instead, the tank is heated until it reaches a charge temperature threshold, then cooled until it reaches a discharge temperature threshold, and this is repeated several times (Touzo Citation2021). This process has an impact on the performance of the storage system. It is during the thermal cycling process that the bed of solid materials expands in volume due to thermal expansion. These dilations induce a compression of the bed of solids as a result of the stresses on the wall. Performance degradation is therefore proportional to the number of cycles. Numerical and experimental work has been carried out to understand the effect of cycling on the performance of dual-media thermocline systems (Touzo et al. Citation2020; Bruch, Fourmigue, Couturier Citation2014; Cascetta et al. Citation2014). Fasquelle (Citation2017) has numerically demonstrated the effect of cycling on the performance of a storage system depending on the temperature thresholds for charging or discharging. It has been shown that the performance of the thermocline decreases rapidly if the temperature thresholds that determine charging and discharging shutdowns are very strict. This is shown in (a), where the charge and discharge curves are very close after 20 cycles, revealing that a large part of the tank is occupied by the thermocline zone. However, he showed that a flexible choice of threshold temperatures enables stability to be achieved after around 3 cycles ((b)). It should be noted that greater extraction from the thermocline zone during charging and discharging leads to good conservation of stratification, and better conservation of thermal stratification provides good storage system performance. Aubin Touzo et al. have numerically and experimentally evaluated the impact of cycling on system performance Touzo et al. Citation2020). His results seem to confirm the work of Thomas Fasquelle showing that a flexible choice of threshold temperature effectively promotes rapid stability of the storage system, thus reducing the degradation of system performance.
Figure 14. (a) Final temperature profiles for cycling with thresholds of 0.1 (b) Final temperature profiles for cycling with thresholds of 0.3 (Fasquelle Citation2017).

In the light of these studies, a judicious choice of threshold temperatures in a flexible mode would minimise the effect of cycling on the behaviour of the storage system.
4.3.3. Effect of the thermo-physical properties of the fluid and solid materials
The physical properties of the materials, such as thermal conductivity and thermal capacity, also influence the performance of the thermocline storage system, according to a few studies reported in the literature.
4.3.3.1. Effect of the thermal conductivity of fluids and solids
The thermal conductivity of the fluid or solid has an effect on the efficiency of the TES (Karim Citation2011). Aly and El-Sharkaw (Citation1990) have shown numerically that an increase in the thermal conductivity of solids induces a significant increase in the loading rate and the amount of stored energy, causing a rise in temperature throughout the bed during a given loading period, but which is completely reversed beyond the given period.
Gang Wang et al. carried out an exhaustive analysis of the parameters influencing the thermal and mechanical performance of a dual media thermocline reservoir (Wang et al. Citation2020). In this study, they evaluated the impact of variation of the thermal conductivity of the filler material on the thickness of the thermocline and on the output temperature during charging and discharging (Wang et al. Citation2020). As shown in , they observed that the increase in thermal conductivity did not significantly influence the output temperature during either charging or discharging. However, the thickness of the thermocline increases as the conductivity increases for a charging or discharging time of less than 4 hours, and this effect becomes virtually insignificant after 4 hours. In addition, Fernando A. Rodrigues and Marcelo J.S. De Lemos conducted a study on the effect of storage material properties on the thermal efficiencies of a thermocline storage system using air as the heat transfer fluid (Rodrigues and De Lemos Citation2020). With different Reynolds numbers, the authors numerically evaluated the effect of varying the ks/kf ratio on the thickness of the thermocline, on the charging efficiency and on the storage efficiency. The performance survey showed that a reduction of the ks/kf ratio, i.e. a lower thermal conductivity of the solid than that of the fluid, improved the efficiency of the system for small Reynolds numbers, whereas increasing the Reynolds number gradually reduced this effect (Rodrigues and De Lemos Citation2020).
Figure 15. Thermocline thickness and outlet molten salt temperature variations during discharging (a) and charging (b) processes under different thermal conductivities of filler particle.

Contrary to previous studies, Vannerem, Neveu, and Falcoz (Citation2021), through a numerical study, have shown that increasing the effective thermal conductivity means reducing the utilisation rate, favouring destratification and thus considerably reducing storage performance.
The effect of the thermal conductivity of the materials is not well understood, given the conclusions of the above studies. A more in-depth study of the effect of the thermal conductivity of the solid or fluid on the performance of the storage system should therefore be carried out to refine the choice of the best pairings (solid material or thermal fluid) in order to improve the performance of Dual Media Thermocline storage systems.
4.3.3.2 . Effect of the heat capacity of the fluid and the solid
Just as the thermal conductivity of the materials has an impact on the performance of the storage system, so the thermal capacity or specific heat also has an effect. Aly and El-Sharkaw (Citation1990) have examined how the properties of the storage substrate affect the thermal behaviour of packed systems during charging. The results showed that increasing the specific heat resulted in a significant improvement in the charging rate and an increase in the storage capacity of the packing bed, while reducing the rate at which the temperature of the solid rose. The influence of this parameter has also been demonstrated numerically by Wang et al. (Citation2020). They observed that increasing the specific heat of the particle led to a total increase in discharge and charge time, with a simultaneous decrease in the thickness of the thermocline. However, they also noticed that the high value of this parameter causes high mechanical stress. Therefore, a higher specific heat of the particle can lead to an improvement in thermal performance, but at the same time an increase in the maximum mechanical stress of the wall. In addition, Rodrigues and De Lemos (Citation2020), mentioned the influence of the ratio of the specific heat of the solid and that of the fluid on the performance when charging a TES. A high value of this ratio with a high Reynolds number increases the efficiency of the storage.
From the above, we can see that the influence of specific heat is closely linked to the mass flow rate or speed of the fluid, creating a trade-off between an increase in thermal storage capacity and an increase in mechanical stress. Therefore, to efficiently store and remove heat in a packed tank, a high specific heat of the solid materials compared to that of the fluid would be an advantage.
5. Conclusion
A review of the literature shows that there is a lack of experimental data on the effect of operating and geometric parameters on the performance of dual media storage systems. However, there are many numerical studies on the impact of these parameters. All these experimental and numerical studies have allowed some conclusions to be drawn:
Cylindrical tanks (tall and narrow for laboratory or pilot scale applications, short and wide for industrial scale) are the most widely used and are more efficient than truncated cone and horizontal rectangular tanks.
Better storage efficiency is achieved with very small spherical particles and better thermal insulation of the system, especially on a laboratory and pilot scale.
A porosity of less than 0.2 improves the efficiency of solid/gas systems, while a high porosity has no significant effect on solid/liquid systems.
There is still some incoherence about the effect of operating temperature or temperature difference on storage performance. Similarly, there is a contradictory prediction of the effect of fluid speed, despite some authors suggesting the existence of an optimum fluid velocity.
The effect of fluid distribution is very significant in single medium thermocline storage systems and less noticeable in dual media thermocline systems due to the packing, which homogenises fluid circulation for fluid collection and injection, whatever the type of diffuser.
Judicious selection of threshold temperatures in a flexible way minimises the effect of cycling on the behaviour of the storage system.
There is some controversy about the impact of thermal conductivity and this shows that the effect of this phenomenon on performance is not well understood. A more in-depth study of the effect of this parameter on the performance of the storage system is therefore required.
The effect of thermal capacity or specific heat on system performance is closely linked to the mass flow rate or fluid velocity and there is a trade-off between thermal storage capacity and the mechanical stresses generated.
The dual media thermocline storage system is undoubtedly a promising alternative for reducing the cost of storage systems and making CSP attractive and accessible to developing countries. A better understanding of this system is therefore necessary to increase its maturity in thermodynamic solar power plants. It is very important that, in the future, we focus on the compensatory effects of the various geometric and operational parameters through meticulous numerical studies validated by experimental studies in order to determine the optimum values of the parameters for improved efficiency of the dual media thermocline storage system. The compensatory effects of criteria such as porosity and fluid velocity; temperature difference and heat capacity of the fluid or solid; fluid velocity and variation in thermal conductivity or heat capacity; reservoir height and variation in thermal conductivity or heat capacity; particle diameter and thermal conductivity or heat capacity; porosity and temperature difference, etc. should be taken into account in future studies to better understand the phenomena that govern and act on the performance of the dual-media thermocline system.
Disclosure statement
No potential conflict of interest was reported by the author(s).
References
- Abdulla, A., and K. S. Reddy. 2017. “Effect of Operating Parameters on Thermal Performance of Molten Salt Packed-bed Thermocline Thermal Energy Storage System for Concentrating Solar Power Plants.” International Journal of Thermal Sciences 121: 30–44. https://doi.org/10.1016/j.ijthermalsci.2017.07.004.
- Adebiyi, G. A., E. C. Nsofor, W. G. Steele, and A. A. Jalalzadeh-Azar. 1993. “Parametric Study on the Operating Efficiencies of a Packed bed for High-Temperature Sensible Heat Storage.” The American Society of Mechanical Engineers Advanced Energy Systems Division AES 30: 59–71.
- Aly, S. L., and A. I. El-Sharkaw. 1990. “Effect of Storage Medium on Thermal Properties of Packed Beds.” Heat Recovery Systems and CHP 10 (5-6): 509–517. https://doi.org/10.1016/0890-4332(90)90201-T
- Angelini, G., A. Lucchini, and G. Manzolini. 2014. “Comparison of Thermocline Molten Salt Storage Performances to Commercial Two-Tank Configuration.” Energy Procedia 49: 694–704. https://doi.org/10.1016/j.egypro.2014.03.075.
- Arpino, F., N. Massarotti, A. Mauro, and L. Vanoli. 2014. “Modeling of Thermal Energy Storage: A Review of Different Systems.”
- Bagre, B. 2021. “Assessment of Different Sands Potentiality to Formulate an Effective Thermal Energy Storage Material (TESM).” Journal de Physique de la SOAPHYS 2 (2020): 1–7. https://doi.org/10.46411/jpsoaphys.2020.01.08.
- Bagre, B., et al. 2022. “Development of Sensible Heat Storage Materials Using Sand, Clay and Coal Bottom Ash.” Materials Sciences and Applications 13 (12): 603–626. https://doi.org/10.4236/msa.2022.1312038.
- Bagre, B., Ibrahim Kolawole Muritala, Tizane Daho, Jacques Nébié, Téré Dabilgou, Eric Mensah Mortey, Issoufou Ourma, et al. 2023. “Modelling and Simulation of a Sustainable Thermal Energy Storage System for Concentrating Solar Power (CSP) Plant Using Eco-materials.” JP Journal of Heat and Mass Transfer (31): 147–161. https://doi.org/10.17654/0973576323010.
- Baoshan, X., B. Nicolas, S. Jérôme, F. Yilin, and L. Lingai. 2022. “Wall Impact on Efficiency of Packed-bed Thermocline Thermal Energy Storage System.” Energy 247 (0360-5542): 123503. https://doi.org/10.1016/j.energy.2022.123503.
- Bayón, R., and E. Rojas. 2013. “Simulation of Thermocline Storage for Solar Thermal Power Plants : From Dimensionless Results to Prototypes and Real-size Tanks.” International Journal of Heat and Mass Transfer 60: 713–721. https://doi.org/10.1016/j.ijheatmasstransfer.2013.01.047.
- Bayón, R., and E. Rojas. 2014. “Analytical Function Describing the Behaviour of a Thermocline Storage Tank: A Requirement for Annual Simulations of Solar Thermal Power Plants.” International Journal of Heat and Mass Transfer 68: 641–648. https://doi.org/10.1016/j.ijheatmasstransfer.2013.09.070.
- Bejan, A. 1978. “Two Thermodynamic Optima in the Design of Sensible Heat Units for Energy Storage.” Journal of Heat Transfer 100 (4): 708–712. https://doi.org/10.1115/1.3450882.
- Bellenot, G. 2021. Etude de l ‘ influence de la distribution de fluide sur le comportement thermohydraulique d ‘ un réservoir de stockage thermique mono-cuve dual-media.
- Bellenot, G., F. Bentivoglio, P. Marty, A. Bruch, and M. Coudrais-duhamel. 2019. “Thermocline Energy Storage : Influence of Fluid Distribution into Porous Media Thermocline Energy Storage.” AIP Conference Proceedings 200006 (July): 200006-1–200006-8. https://doi.org/10.1063/1.5117721.
- Boubou, B., et al. 2021. “Review on Thermocline Storage Effectiveness for Concentrating Solar Power Plant.” Energy and Power Engineering 13 (10): 343–364. https://doi.org/10.4236/epe.2021.1310024.
- Bradshaw, R. W., and N. P. Siegel. 2016. “ES2008-54174”, 1–7.
- Brosseau, D., J. W. Kelton, D. Ray, M. Edgar, K. Chisman, and B. Emms. 2005. “Testing of Thermocline Filler Materials and Molten-Salt Heat Transfer Fluids for Thermal Energy Storage Systems in Parabolic Trough Power Plants.” Journal of Solar Energy Engineering 127 (1): 109–116. https://doi.org/10.1115/1.1824107.
- Bruch, A., J. F. Fourmigué, and R. Couturier. 2014. “Experimental and Numerical Investigation of a Pilot-Scale Thermal oil Packed bed Thermal Storage System for CSP Power Plant.” Solar Energy 105: 116–125. https://doi.org/10.1016/j.solener.2014.03.019.
- Bruch, A., J. F. Fourmigue, R. Couturier, and S. Molina. 2014. “Experimental and Numerical Investigation of Stability of Packed Bed Thermal Energy Storage for CSP Power Plant.” Energy Procedia 49: 743–751. https://doi.org/10.1016/j.egypro.2014.03.080.
- Bruch, A., Sophie Molina, T Esence, J.F Fourmigué, and R Couturier 2017. “Experimental Investigation of Cycling Behaviour of Pilot-Scale Thermal oil Packed-bed Thermal Storage System.” Renewable Energy 103 (2016): 277–285. https://doi.org/10.1016/j.renene.2016.11.029.
- Calderón-Vásquez, I., et al. 2021. “Review on Modeling Approaches for Packed-bed Thermal Storage Systems.” Renewable and Sustainable Energy Reviews 143 (February): 110902-1–110902-22. https://doi.org/10.1016/j.rser.2021.110902.
- Calvet, N., et al. 2013. “Compatibility of a Post-Industrial Ceramic with Nitrate Molten Salts for Use as Filler Material in a Thermocline Storage System.” Applied Energy 109: 387–393. https://doi.org/10.1016/j.apenergy.2012.12.078.
- Calvet, N., A. Gil, J. Rodríguez-aseguinolaza, A. Faik, and B. D. Aguanno. 2015. “Thermophysical Characterization of a By-product from the Steel Industry to be used as a Sustainable and Low-cost Thermal Energy Storage Material.” Energy 89 (2015): 601–609. https://doi.org/10.1016/j.energy.2015.05.153.
- Capocelli, M., G. Caputo, M. De Falco, I. Balog, and V. Piemonte. 2019. “Numerical Modeling of a Novel Thermocline Thermal Storage for Concentrated Solar Power.” Journal of Solar Energy Engineering 141 (October): 1–8. https://doi.org/10.1115/1.4043082.
- Cárdenas, B., et al. 2019. “Techno-economic Optimization of a Packed-bed for Utility-scale Energy Storage.” Applied Thermal Engineering 153 (February): 206–220. https://doi.org/10.1016/j.applthermaleng.2019.02.134.
- Cárdenas, B., T. R. Davenne, J. P. Rouse, and S. D. Garvey. 2018. “Effect of Design Parameters on the Exergy Efficiency of a Utility-Scale Packed bed.” Journal of Energy Storage 18 (December 2018): 267–284. https://doi.org/10.1016/j.est.2018.05.005.
- Cascetta, M., G. Cau, P. Puddu, and F. Serra. 2014. “Numerical Investigation of a Packed Bed Thermal Energy Storage System with Different Heat Transfer Fluids.” Energy Procedia 45: 598–607. https://doi.org/10.1016/j.egypro.2014.01.064.
- Chang, Z. S., X. Li, C. Xu, C. Chang, and Z. F. Wang. 2015. “The Design and Numerical Study of a 2MWh Molten Salt Thermocline Tank.” Energy Procedia 69: 779–789. https://doi.org/10.1016/j.egypro.2015.03.094.
- Clauser, C., E. Huenges, and L. Bodenforschung. 1995. “Thermal Conductivity of Rocks and Minerals.” J 0xj.
- Cocco, D., and F. Serra. 2015. “Performance Comparison of two-Tank Direct and Thermocline Thermal Energy Storage Systems for 1 MWe Class Concentrating Solar Power Plants.” Energy 81: 526–536. https://doi.org/10.1016/j.energy.2014.12.067.
- Eames, P. C., and B. Norton. 1998. “The Effect of Tank Geometry on Thermally Stratified Sensible Heat Storage Subject to low Reynolds Number Flows.” International Journal of Heat and Mass Transfer 41 (14): 2131–2142. https://doi.org/10.1016/S0017-9310(97)00349-9.
- Erregueragui, Z., N. Boutammachte, and A. Bouatem. 2016. “Packed-bed Thermal Energy Storage Analysis: Quartzite and Palm- Oil Performance.” Energy Procedia 99 (March): 370–379. https://doi.org/10.1016/j.egypro.2016.10.127.
- Esence, T., A. Bruch, J. F. Fourmigué, and B. Stutz. 2019. “A Versatile one-Dimensional Numerical Model for Packed-bed Heat Storage Systems.” Renewable Energy 133: 190–204. https://doi.org/10.1016/j.renene.2018.10.012.
- Esence, T., A. Bruch, S. Molina, B. Stutz, and J. F. Fourmigué. 2017. “A Review on Experience Feedback and Numerical Modeling of Packed-bed Thermal Energy Storage Systems.” Solar Energy 153: 628–654. https://doi.org/10.1016/j.solener.2017.03.032.
- Esence, T., T. Desrues, J. Fourmigué, G. Cwicklinski, A. Bruch, and B. Stutz. 2019. “Experimental Study and Numerical Modelling of High Temperature gas/Solid Packed-bed Heat Storage Systems.” Energy 180 (2019): 61–78. https://doi.org/10.1016/j.energy.2019.05.012.
- Faas, S. E., L. R. Thorne, E. A. Fuchs, and N. D. Gilbertsen. 1986. “10 MW/sub e/ Solar Thermal Central Receiver Pilot Plant: Thermal Storage Subsystem Evaluation. Final Report Other Inf. Portions this Doc. are Illegible Microfich. Prod. Orig. copy available until Stock is exhausted, p. Medium: X; Size: Pages: 113.
- Farmahini-Farahani, M. 2012. “Investigation of Four Geometrical Parameters on Thermal Stratification of Cold Water Tanks by Exergy Analysis.” International Journal of Exergy 10 (3): 332–345. https://doi.org/10.1504/IJEX.2012.046814.
- Fasquelle, T. 2017. Modélisation et caractérisation expérimentale d’une boucle solaire cylindro-parabolique intégrant un stockage de type thermocline., Thèse de Doctorat de Physique, Université de Perpignan Via Domitia.
- Fasquelle, T., Q. Falcoz, P. Neveu, and J. F. Hoffmann. 2018a. “Numerical Simulation of a 50 MWe Parabolic Trough Power Plant Integrating a Thermocline Storage Tank.” Energy Conversion and Management 172 (July): 9–20. https://doi.org/10.1016/j.enconman.2018.07.006.
- Fasquelle, T., Q. Falcoz, P. Neveu, and J. F. Hoffmann. 2018b. “A Temperature Threshold Evaluation for Thermocline Energy Storage in Concentrated Solar Power Plants.” Applied Energy 212 (2018): 1153–1164. https://doi.org/10.1016/j.apenergy.2017.12.105.
- Fernández-torrijos, M., C. Sobrino, and J. A. Almendros-ibáñez. 2017. “Simplified Model of a Dual-Media Molten-Salt Thermocline Tank with a Multiple Layer Wall.” Solar Energy 151: 146–161. https://doi.org/10.1016/j.solener.2017.04.072.
- Flueckiger, S. M., and S. V. Garimella. 2012. “Second-law Analysis of Molten-Salt Thermal Energy Storage in Thermoclines.” Solar Energy 86 (5): 1621–1631. https://doi.org/10.1016/j.solener.2012.02.028.
- Galione, P., C. Pérez-segarra, I. Rodríguez, S. Torras, and J. Rigola. 2015. “Numerical Evaluation of Multi-Layered Solid-PCM Thermocline-Like Tanks as Thermal Energy Storage Systems for CSP Applications.” Energy Procedia 69: 832–841. https://doi.org/10.1016/j.egypro.2015.03.099.
- García Mari, P., E. Gasque Albalate, M. Gutiérrez Colomer, R. P. Ibáñez Solís, and F. González Altozano. 2013. “A New Inlet Device that Enhances Thermal Stratification During Charging in a Hot Water Storage Tank.” Journal of the American Chemical Society. 123 (10): 2176–2181. https://doi.org/10.1016/j.applthermaleng.2013.08.023.The.
- Geissbühler, L., V. Becattini, G. Zanganeh, S. Zavattoni, M. Barbato, and A. Haselbacher. 2018. “Pilot-scale Demonstration of Advanced Adiabatic Compressed air Energy Storage, Part 1: Plant Description and Tests with Sensible Thermal-Energy Storage.” Journal of Energy Storage 17: 129–139. https://doi.org/10.1016/j.est.2018.02.004.
- Gil, A., et al. 2010. “State of the Art on High Temperature Thermal Energy Storage for Power Generation. Part 1—Concepts, Materials and Modellization.” Renewable and Sustainable Energy Reviews 14 (1): 31–55. https://doi.org/10.1016/j.rser.2009.07.035.
- Gutierrez, A., Laia Miró, Antoni Gil, Javier Rodríguez-Aseguinolaza, Camila Barreneche, Nicolas Calvet, Xavier Py, et al. 2016. “Industrial Waste Materials and By-products as Thermal Energy Storage (TES) Materials: A Review.” AIP Conference Proceedings. 1734 (1): 050019-1–050019-9. https://doi.org/10.1063/1.4949117.
- Haller, M. Y., C. A. Cruickshank, W. Streicher, S. J. Harrison, E. Andersen, and S. Furbo. 2009. “Methods to Determine Stratification Efficiency of Thermal Energy Storage Processes – Review and Theoretical Comparison.” Solar Energy 83 (10): 1847–1860. https://doi.org/10.1016/j.solener.2009.06.019.
- Hänchen, M., S. Brückner, and A. Steinfeld. 2011. “High-temperature Thermal Storage Using a Packed bed of Rocks – Heat Transfer Analysis and Experimental Validation.” Applied Thermal Engineering 31 (10): 1798–1806. https://doi.org/10.1016/j.applthermaleng.2010.10.034.
- Hasan, F. M., and M. A. Theeb. 2021. “Effect of Diffuser Height on Thermocline in Stratified Chilled Water Storage Tank.” Journal of Applied Fluid Mechanics 14 (2): 429–438. https://doi.org/10.47176/jafm.14.02.31450.
- Hernandez, A. B., I. Uriz, I. Ortega-Fernández, J. Rodriguez-Aseguinolaza, A. Ortuondo, and A. Faik. 2018. “Solid Packed Bed Thermal Energy Storage for ORC Electric Generation in Fresnel Type CSP Plants.” AIP Conference Proceeding 2033 (November): 090013-1–090013-8. https://doi.org/10.1063/1.5067107.
- Hoffmann, J. 2015. “Stockage thermique pour centrale solaire thermodynamique à concentration mettant en oeuvre des matériaux naturels ou recyclés.” (Thèse de Doctorat de Physique). Université de Perpignan Via Domitia.
- Hoffmann, J. F., et al. 2018. “Temperature Dependence of Thermophysical and Rheological Properties of Seven Vegetable Oils in View of their Use as Heat Transfer Fluids in Concentrated Solar Plants.” Solar Energy Materials and Solar Cells 178 (December 2018): 129–138. https://doi.org/10.1016/j.solmat.2017.12.037.
- Hoffmann, J. F., T. Fasquelle, V. Goetz, and X. Py. 2016. “A Thermocline Thermal Energy Storage System with Filler Materials for Concentrated Solar Power Plants: Experimental Data and Numerical Model Sensitivity to Different Experimental Tank Scales.” Applied Thermal Engineering 100: 753–761. https://doi.org/10.1016/j.applthermaleng.2016.01.110.
- Hoffmann, J. F., T. Fasquelle, V. Goetz, and X. Py. 2017. “Experimental and Numerical Investigation of a Thermocline Thermal Energy Storage Tank.” Applied Thermal Engineering 114: 896–904. https://doi.org/10.1016/j.applthermaleng.2016.12.053.
- Ibrahim, D., and R. Marc. 2011. A Thermal Energy Storage Systems and Applications.
- Ismail, K. A. R., and R. Stuginsky. 1999. “A Parametric Study on Possible Fixed bed Models for PCM and Sensible Heat Storage.” Applied Thermal Engineering 19 (7): 757–788. https://doi.org/10.1016/S1359-4311(98)00081-7.
- Jemmal, Y., N. Zari, and M. Maaroufi. 2016. “Thermophysical and Chemical Analysis of Gneiss Rock as low Cost Candidate Material for Thermal Energy Storage in Concentrated Solar Power Plants.” Solar Energy Materials and Solar Cells 157: 377–382. https://doi.org/10.1016/j.solmat.2016.06.002.
- Karim, M. A. 2011. “Experimental Investigation of a Stratified Chilled-Water Thermal Storage System.” Applied Thermal Engineering 31 (11-12): 1853–1860. https://doi.org/10.1016/j.applthermaleng.2010.12.019.
- Kocak, B., and H. Paksoy. 2020. “Performance of Laboratory Scale Packed-bed Thermal Energy Storage Using new Demolition Waste Based Sensible Heat Materials for Industrial Solar Applications.” Solar Energy 211 (November): 1335–1346. https://doi.org/10.1016/j.solener.2020.10.070.
- Kuravi, S., J. Trahan, D. Y. Goswami, M. M. Rahman, and E. K. Stefanakos. 2013. “Thermal Energy Storage Technologies and Systems for Concentrating Solar Power Plants.” Progress in Energy and Combustion Science 39 (4): 285–319. https://doi.org/10.1016/j.pecs.2013.02.001.
- Laube, T., L. Marocco, K. Niedermeier, J. Pacio, and T. Wetzel. 2020. “Thermodynamic Analysis of High-Temperature Energy Storage Concepts Based on Liquid Metal Technology.” Energy Technology 8 (3): 1900908-1–1900908-10. https://doi.org/10.1002/ente.201900908.
- Li, S., Y. Li, X. Zhang, and C. Wen. 2013. “Experimental Study on the Discharging Performance of Solar Storage Tanks with Different Inlet Structures.” International Journal of Low-Carbon Technologies 8 (3): 203–209. https://doi.org/10.1093/ijlct/cts023.
- Li, S. H., Y. X. Zhang, Y. Li, and X. S. Zhang. 2014. “Experimental Study of Inlet Structure on the Discharging Performance of a Solar Water Storage Tank.” Energy and Buildings 70: 490–496. https://doi.org/10.1016/j.enbuild.2013.11.086.
- Liu, M., M. Belusko, N. H. Steven Tay, and F. Bruno. 2014. “Impact of the Heat Transfer Fluid in a Flat Plate Phase Change Thermal Storage Unit for Concentrated Solar Tower Plants.” Solar Energy 101: 220–231. https://doi.org/10.1016/j.solener.2013.12.030.
- Lou, W., Y. Fan, and L. Luo. 2020. “Single-tank Thermal Energy Storage Systems for Concentrated Solar Power: Flow Distribution Optimization for Thermocline Evolution Management.” Journal of Energy Storage 32 (August): 101749. https://doi.org/10.1016/j.est.2020.101749.
- Mawire, A., and M. McPherson. 2009. “Experimental and Simulated Temperature Distribution of an Oil-pebble Bed Thermal Energy Storage System with a Variable Heat Source.” Applied Thermal Engineering 29 (5-6): 1086–1095. https://doi.org/10.1016/j.applthermaleng.2008.05.028.
- Medrano, M., A. Gil, I. Martorell, X. Potau, and L. F. Cabeza. 2010. “State of the art on High-Temperature Thermal Energy Storage for Power Generation. Part 2—Case Studies.” Renewable and Sustainable Energy Reviews 14 (1): 56–72. https://doi.org/10.1016/j.rser.2009.07.036.
- Melanson, M. M., and A. G. Dixon. 1985. “Solid Conduction in low dt/dp Beds of Spheres, Pellets and Rings.” International Journal of Heat and Mass Transfer 28 (2): 383–394. https://doi.org/10.1016/0017-9310(85)90071-7.
- Mira-Hernández, C., S. M. Flueckiger, and S. V. Garimella. 2014. “Numerical Simulation of Single- and Dual-Media Thermocline Tanks for Energy Storage in Concentrating Solar Power Plants.” Energy Procedia 49: 916–926. https://doi.org/10.1016/j.egypro.2014.03.099.
- Modi, A., and F. Haglind. 2014. “Performance Analysis of a Kalina Cycle for a Central Receiver Solar Thermal Power Plant with Direct Steam Generation.” Applied Thermal Engineering 65 (1-2): 201–208. https://doi.org/10.1016/j.applthermaleng.2014.01.010.
- Motte, F., S. L. Bugler-Lamb, and Q. Falcoz. 2015. “Thermocline Storage Filled with Structured Ceramics. Numerical Consistency of the Developed Numerical Model and First Observations.” High Temperature Materials and Processes 34 (4): 353–365. https://doi.org/10.1515/htmp-2014-0057.
- Motte, F., S. L. Bugler-lamb, Q. Falcoz, and X. Py. 2014. “Numerical Study of a Structured Thermocline Storage Tank Using Vitrified Waste as Filler Material.” Energy Procedia 49: 935–944. https://doi.org/10.1016/j.egypro.2014.03.101.
- Nandi, B. R., S. Bandyopadhyay, and R. Banerjee. 2018. “Numerical Modeling and Analysis of Dual Medium Thermocline Thermal Energy Storage.” Journal of Energy Storage 16: 218–230. https://doi.org/10.1016/j.est.2018.01.020.
- Nellis, G., and S. Klein. 1977. Heat Transfer.
- Nicolas, L. F., et al. 2019. “Flexibility and Robustness of a High-Temperature Air/Ceramic Thermocline Heat Storage Pilot.” Journal of Energy Storage 21 (August 2018): 393–404. https://doi.org/10.1016/j.est.2018.11.034.
- Odenthal, C., W. Steinmann, S. Zunft, G. Aerospace, and L. Höhe. 2020a. “Analysis of a Horizontal Flow Closed Loop Thermal Energy Storage System in Pilot Scale for High Temperature Applications – Part I: Experimental Investigation of the Plant.” Applied Energy 263 (January): 114573. https://doi.org/10.1016/j.apenergy.2020.114573.
- Odenthal, C., W. Steinmann, S. Zunft, G. Aerospace, and L. Höhe. 2020b. “Analysis of a Horizontal Flow Closed Loop Thermal Energy Storage System in Pilot Scale for High Temperature Applications – Part II: Numerical Investigation.” Applied Energy 263 (January): 114576. https://doi.org/10.1016/j.apenergy.2020.114576.
- Ortega-fernández, I., I. Loroño, A. Faik, I. Uriz, J. Rodríguez-Aseguinolaza, and B. D’Aguanno 2017. “Parametric Analysis of a Packed Bed Thermal Energy Storage System.” AIP Conference Proceedings 1850 (1): 080021-1–080021-8. https://doi.org/10.1063/1.4984442.
- Pacheco, J. E., S. K. Showalter, and W. J. Kolb. 2001. “Development of a Molten-Salt Thermocline Thermal Storage System for Parabolic Trough Plants.” Solar Energy Conference 124 (2002): 453–460. https://doi.org/10.1115/sed2001-158.
- Py, X., N. Calvet, A. Meffre, P. Echegut, C. Bessada, and S. Ory. 2011. “Recycled Material for Sensible Heat Based Thermal Energy Storage to be Used in Concentrated Solar Thermal Power Plants.” Journal of Solar Energy Engineering 133 (3): 031008-1–031008-8. https://doi.org/10.1115/1.4004267.
- Py, Y. L. X., and A. M. R. Olives. 2016. “Comparative LCA Between Current and Alternative Waste-Based TES for CSP.” Waste Biomass Valor 7 (2016): 1509–1519. https://doi.org/10.1007/s12649-016-9549-6.
- Qin, F. G. F., et al. 2012. “Thermocline Stability Criterions in Single-Tanks of Molten Salt Thermal Energy Storage.” Applied Energy 97: 816–821. https://doi.org/10.1016/j.apenergy.2012.02.048.
- Raymon, H., and G. Robert. 1997. “Solaar Thermal Power Systeme Phase I, CRDL ITEM2 Pilot Plant Preliminary Design Report.” 1, 10.
- Reddy, K. S., and N. Pradeep. 2021. “Stability Analysis of the Thermocline Thermal Energy Storage System During High Flow Rates for Solar Process Heating Applications.” Solar Energy 226 (August 2021): 40–53. https://doi.org/10.1016/j.solener.2021.08.026.
- Rodrigues, F. A., and M. J. S. De Lemos. 2020. “Effect of porous material properties on thermal efficiencies of a thermocline storage tank.” Applied Thermal Engineering 173 (February): 115194. https://doi.org/10.1016/j.applthermaleng.2020.115194.
- Rosen, M. A. 1999. “Second-law Analysis of Aquifer Thermal Energy Storage Systems.” Energy 24 (2): 167–182. https://doi.org/10.1016/S0360-5442(98)00080-2.
- Rosen, M. A. 2001. “The Exergy of Stratified Thermal Energy Storages.” Solar Energy 71 (3): 173–185. https://doi.org/10.1016/S0038-092X(01)00036-6.
- Rosen, M. A. 2012. “Appropriate Thermodynamic Efficiency Measures for Closed Systems for Energy Storage.” Energy Storage 114 (May 1992): 47–74.
- Rosen, M. A., and I. Dincer. 1997. “ON Exergy and Environmental Impact.” International Journal of Energy Research 21 (7): 643–654. https://doi.org/10.1002/(SICI)1099-114X(19970610)21:7<643::AID-ER284>3.0.CO;2-I.
- Rosen, M. A., R. Tang, and I. Dincer. 2004. “Effect of Stratification on Energy and Exergy Capacities in Thermal Storage Systems.” International Journal of Energy Research 28 (2): 177–193. https://doi.org/10.1002/er.960.
- Sassine, N., F. Donzé, and A. Bruch. 2017. “Rock-Bed Thermocline Storage : A Numerical Analysis of Granular Bed Behavior and Interaction with Storage Tank.” AIP Conference Proceedings 1850 (1): 080023-1–080023-7. https://doi.org/10.1063/1.4984444.
- Sassine, N., F. V. Donzé, B. Harthong, and A. Bruch. 2018. “Thermal Stress Numerical Study in Granular Packed bed Storage Tank.” Granular Matter 20 (3): 1–15. https://doi.org/10.1007/s10035-018-0817-y.
- Schlipf, D., P. Schicktanz, H. Maier, and G. Schneider. 2015. “Using Sand and Other Small Grained Materials as Heat Storage Medium in a Packed Bed HTTESS.” Energy Procedia 69: 1029–1038. https://doi.org/10.1016/j.egypro.2015.03.202.
- Shah, L. J., and S. Furbo. 2003. “Entrance Effects in Solar Storage Tanks.” Solar Energy 75 (4): 337–348. https://doi.org/10.1016/j.solener.2003.04.002.
- Tian, Y., and C. Y. Zhao. 2013. “A Review of Solar Collectors and Thermal Energy Storage in Solar Thermal Applications.” Applied Energy 104: 538–553. https://doi.org/10.1016/j.apenergy.2012.11.051.
- Tiskatine, R., et al. 2017. “Suitability and Characteristics of Rocks for Sensible Heat Storage in CSP Plants.” Solar Energy Materials and Solar Cells 169 (December 2017): 245–257. https://doi.org/10.1016/j.solmat.2017.05.033.
- Touzo, A., et al. 2020. “Experimental and Numerical Analysis of a Packed-bed Thermal Energy Storage System Designed to Recover High Temperature Waste Heat: An Industrial Scale up.” Journal of Energy Storage 32 (May): 101894. https://doi.org/10.1016/j.est.2020.101894.
- Touzo, A.. 2021. Intégration d’un stockage de chaleur de type thermocline à des procédés énergétiques. 2021PERP0016 . tel-03353418.
- Van Lew, J. T., P. Li, C. L. Chan, W. Karaki, and J. Stephens. 2011. “Analysis of Heat Storage and Delivery of a Thermocline Tank Having Solid Filler Material.” Journal of Solar Energy Engineering 133 (2): 021003-1–021003-10. https://doi.org/10.1115/1.4003685.
- Vannerem, S. 2022. “Étude numérique et expérimentale de l ‘ influence des conditions opératoires sur un stockage de type thermocline intégré à une centrale solaire thermodynamique Segolène Vannerem To cite this version : HAL Id : tel-03574574 Spécialité : Énergétique et géni”.
- Vannerem, S., P. Neveu, and Q. Falcoz. 2021. “Experimental and Numerical Investigation of the Impact of Operating Conditions on Thermocline Storage Performance.” Renewable Energy 168: 234–246. https://doi.org/10.1016/j.renene.2020.12.061.
- Vignarooban, K., X. Xu, A. Arvay, K. Hsu, and A. M. Kannan. 2015. “Heat Transfer Fluids for Concentrating Solar Power Systems – A Review.” Applied Energy 146: 383–396. https://doi.org/10.1016/j.apenergy.2015.01.125.
- Wang, L., Z. Yang, and Y. Duan. 2015. “Influence of Flow Distribution on the Thermal Performance of Dual-Media Thermocline Energy Storage Systems.” Applied Energy 142: 283–292. https://doi.org/10.1016/j.apenergy.2014.12.024.
- Wang, G., S. Yu, S. Niu, Z. Chen, and P. Hu. 2020. “A Comprehensive Parametric Study on Integrated Thermal and Mechanical Performances of Molten-Salt-Based Thermocline Tank.” Applied Thermal Engineering 170 (September 2019): 115010. https://doi.org/10.1016/j.applthermaleng.2020.115010.
- Wildin, M. W. 1996. “Experimental Results from Single-Pipe Diffusers for Stratified Thermal Energy Storage.” ASHRAE Transactions. 102 (2): 123–132.
- Xie, B. 2022. Thermocline Study of Packed-bed Thermal Energy Storage System.
- Xie, B., and B. Xie. 2022. “Thermocline Study of Packed-bed Thermal Energy Storage System, [En ligne].” Disponible sur. https://hal.archives-ouvertes.fr/tel-03798303.
- Xu, C., X. Li, Z. Wang, Y. He, and F. Bai. 2013. “Effects of Solid Particle Properties on the Thermal Performance of a Packed-bed Molten-Salt Thermocline Thermal Storage System.” Applied Thermal Engineering 57 (1-2): 69–80. https://doi.org/10.1016/j.applthermaleng.2013.03.052.
- Xu, C., Z. Wang, Y. He, X. Li, and F. Bai. 2012a. “Parametric Study and Standby Behavior of a Packed-bed Molten Salt Thermocline Thermal Storage System.” Renewable Energy 48: 1–9. https://doi.org/10.1016/j.renene.2012.04.017.
- Xu, C., Z. Wang, Y. He, X. Li, and F. Bai. 2012b. “Sensitivity Analysis of the Numerical Study on the Thermal Performance of a Packed-bed Molten Salt Thermocline Thermal Storage System.” Applied Energy 92: 65–75. https://doi.org/10.1016/j.apenergy.2011.11.002.
- Yang, Z., and S. V. Garimella. 2010. “Thermal Analysis of Solar Thermal Energy Storage in a Molten-Salt Thermocline.” Solar Energy 84 (6): 974–985. https://doi.org/10.1016/j.solener.2010.03.007.
- Yang, Z., and S. V. Garimella. 2013. “Cyclic Operation of Molten-Salt Thermal Energy Storage in Thermoclines for Solar Power Plants.” Applied Energy 103: 256–265. https://doi.org/10.1016/j.apenergy.2012.09.043.
- Yang, X., X. Yang, F. G. F. Qin, and R. Jiang. 2016. “Experimental Investigation of a Molten Salt Thermocline Storage Tank.” International Journal of Sustainable Emergy 35: 606–614. https://doi.org/10.1080/14786451.2014.930465.
- Yin, H., J. Ding, R. Jiang, and X. Yang. 2017. “Thermocline Characteristics of Molten-Salt Thermal Energy Storage in Porous Packed-bed Tank.” Applied Thermal Engineering 110: 855–863. https://doi.org/10.1016/j.applthermaleng.2016.08.214.
- Zanganeh, G., A. Pedretti, A. Haselbacher, and A. Steinfeld. 2015. “Design of Packed bed Thermal Energy Storage Systems for High-Temperature Industrial Process Heat.” Applied Energy 137: 812–822. https://doi.org/10.1016/j.apenergy.2014.07.110.
- Zanganeh, G., A. Pedretti, S. Zavattoni, M. Barbato, and A. Steinfeld. 2012. “Packed-bed Thermal Storage for Concentrated Solar Power – Pilot-Scale Demonstration and Industrial-Scale Design.” Solar Energy 86 (10): 3084–3098. https://doi.org/10.1016/j.solener.2012.07.019.
- Zhao, B. C., M. S. Cheng, C. Liu, and Z. M. Dai. 2018. “System-level Performance Optimization of Molten-Salt Packed-bed Thermal Energy Storage for Concentrating Solar Power.” Applied Energy 226 (May): 225–239. https://doi.org/10.1016/j.apenergy.2018.05.081.
- Zunft, S., M. Hänel, M. Krüger, V. Dreißigacker, F. Göhring, and E. Wahl. 2011. “Jülich Solar Power Tower—Experimental Evaluation of the Storage Subsystem and Performance Calculation.” Journal of Solar Energy Engineering 133 (3): 1–5. https://doi.org/10.1115/1.4004358.