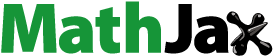
ABSTRACT
It is well known that lubricating oils reduce the friction coefficient between two surfaces in contact. Since petroleum lubricants are toxic and have a low biodegradability, they are typically not appropriate for the environment. As a result, as public worries about a pollution-free environment grow, so does the demand for lubricants that are acceptable to the environment. The primary rationale for utilizing vegetable oils in forthcoming bio-lubricant formulations is their high lubricating performance, low toxicity, sustainable, and biodegradability. Plant oils hold great potential as a foundational fluid for bio-lubricants, since their synthetic and vegetable oil-based esters provide the most environmentally friendly option for creating lubricants. In this study, Jatropha and Jojoba raw oil were chemically modified via epoxidation followed by transesterification to produce bio-lubricants. Thus, the aim of this work is to develop a bio-lubricant from jatropha and jojoba methyl ester, further adding nanoparticles multiwalled nanotubes and titanium dioxide for improvement of tribological properties. The chemical modification of the jatropha and jojoba oil results in a decrease in iodine value, resulting in the breaking of carbon bonds. Viscosity tests were performed using the Anton Par MCR 92 Rheometer, with temperature ranging from 30°C to 80°C. Thermal stability measurements of bio-lubricants were performed using PerkinElmer’s Thermogravimetric analyzer − 4000 instrument and thermal degradation temperature for Epoxidized Jatropha – sulfuric acid, Epoxidized Jatropha – hydrochloric acid, Epoxidized Jojoba – sulfuric acid and Epoxidized Jojoba – Hydrochloric acid samples are 238°C, 224°C, 230°C, and 244°C, respectively. The 94.68% and 79.85% reduction in wear was obtained for Epoxidized Jojoba with hydrochloric acid, titanium dioxide and epoxidized Jatropha with sulfuric acid, multiwalled carbon nanotubes in comparison with their epoxidized samples. The coefficient of friction of Epoxidized Jatropha with sulfuric acid, multiwalled carbon nanotubes and Epoxidized Jojoba with Hydrochloric acid, Titanium dioxide sample was found to be 0.061 and 0.059, respectively.
Introduction
A lubricant is a substance, usually liquid, which, when interposed between two solid surfaces, reduces friction, creating a very thin film between the two contact surfaces (Tesser et al. Citation2020). Petroleum-based lubricants make up more than 95% of those that leak into the environment and are bad for the environment (Schneider Citation2006). Given that most lubricants made of petroleum are hazardous and unsustainable, this is a worrying issue (Seela, Ravisankar, and Raju Citation2018). Unscientific disposal and continuous combustion of petroleum lubricants eventually lead to degradation of the environment (Mannekote et al. Citation2018). The worldwide community is becoming increasingly concerned about the steady depletion of petroleum resources and the escalating environmental concerns (Heikal et al. Citation2017a). Many steps have been taken to mitigate these immediate dangers, such as the creation of sustainable energy systems and the potential substitution of renewable resources for items derived from minerals (Bart, Gucciardi, and Cavallaro Citation2013). All lubricants made from bio-based raw materials, such as vegetable oils, animal fats, or any other hydrocarbon that is environmentally benign, are referred to as “bio-based lubricants” or “bio-lubricants” (Salimon, Salih, and Yousif Citation2010). Bio-based lubricants are reported to have superior lubricity, a higher flash point, a higher viscosity index, and better resistance to shear than mineral oils, in addition to being easily biodegradable (Soni and Agarwal Citation2014). Certain bio-lubricants, particularly unrefined vegetable oils, exhibit inadequate oxidative stability and low-temperature properties. However, these shortcomings can be addressed by adding the right additives, such as oils, and making the appropriate chemical adjustments (Walters Citation1986). The most frequent strategies for changing vegetable oils are transesterification and epoxidation processes (Salih, Salimon, and Yousif Citation2012).
Sulaiman, Chuah, and Fakhru’l-Razi (Citation2007) evaluated a palm oil derivative in which a transesterification reaction produces trimethylolpropane (TMP) under vacuum. The conversion rate was 61% for two hours at 110°C, based on the results. Koh, Ghazi, and Idris (Citation2014) assessed the research on the synthesis of bio-lubricants utilizing TMP, palm methyl ester, and sodium methoxide catalyst (NaOCH3). From 110°C to 150°C, the transesterification reaction was carried out. According to their findings, the ideal reaction temperature for a 25-minute reaction is 140°C. Aziz et al. (Citation2014) utilized sodium methoxide catalyst and palm oil methyl ester as the raw material for the transesterification study. It was discovered that 158°C, 1.56% catalyst concentration, 4:1 molar ratio, and 55 minutes of reaction time were the ideal parameters for the reaction. These ideal circumstances produced a yield of 37.56%. McNutt (Citation2016) conducted transesterification investigations. In comparison to pure oil, his experimental research revealed a decrease in the pour point and an increase in the thermo-oxidative stability of esters. Heikal et al. (Citation2017) employed a two-stage transesterification method to create a bio-lubricant using palm and jatropha oil. As a catalyst, methanol was combined with sodium methoxide and potassium hydroxide. The yield of TMP esters extracted from palm oil was 97.8%, according to the results, during a 4-hour reaction at 130°C. In a similar manner, TMP esters with a yield of 98.2% were extracted from Jatropha oil using comparable reaction conditions. TMP esters made from Jatropha oil satisfied industrial oil requirements with their high viscosity index, low pour point temperature (−3°C), and moderate thermal stability.
Epoxidation increases oxidative stability and it is necessary to convert neat vegetable oil into bio-lubricant. Heterogeneous and homogeneous catalysts, like sulfuric acid and ion exchange resin, are frequently used to speed up the epoxidation process in order to produce larger yields in less time (Aguilera et al. Citation2019). High yields of natural unsaturated triglycerides were obtained at the end of epoxidation by using tungsten, rhenium, and amorphous titanium or silicon dioxide (SiO2)-based catalysts (Goud, Patwardhan, and Pradhan Citation2007). Goud, Patwardhan, and Pradhan (Citation2006) examined the epoxidation of Mahua oil using active oxygen carriers such acetic acid, hydrogen peroxide as an oxygen donor, and mineral acids as catalysts. At the conclusion of the trials, a greater yield in less time was attained. Studies performed by Doll et al. (Citation2017) showed that the carbonated and epoxidized estolides were produced by epoxidizing them with hydrogen peroxide and formic acid, then ring-opening them with carbon dioxide using tetrabutylammonium bromide as a catalyst. According to their research, the viscosities of the epoxidized estolides were significantly higher than those of the original estolides. Sharma and Dalai (Citation2013) evaluated .5:1 acid-to-ethylenenic unsaturation molar ratio and an Amberlite IR-120 catalyst (20% of oil) was used in the investigations. The research findings demonstrated that innovative sulfated Ti-SBA-15(10) was the most active and highly selective catalyst when compared to other commercial catalysts. Unsaturated fatty acid-rich soybean (Adhvaryu and Erhan Citation2002) and rape seed oils (Wu et al. Citation2000), showed better oxidation stability and reduced friction properties after being epoxidized in comparison to their initial forms.
Even though several researchers have optimized the yield of bio-lubricant derived from different vegetable oils, studies related to the synthesis, preparation and characterization of bio-lubricants derived from jatropha and jojoba oils are limited. As per the research literature, elevating the temperature enhances the conversion of epoxidized vegetable oil, while modifying the molar ratio impacts the epoxidized oil output. The ideal temperature, catalyst, and alcohol molar ratios must be maintained in order to enhance the epoxy bio-lubricant’s quality and quantity stages.
Addition of solid and liquid additives to the base lubricant could improve the heat transfer and tribological properties. The addition of micro or nano-sized additives to the mineral oil or synthetic oil-based lubricants enables the lubricant to keep clean and free from debris, allows it to operate at elevated temperatures, and also gives them enhanced wear and frictional characteristics. Pure metals or metal oxides in the form of nanoparticles have been used in the recent years to enhance the lubricating characteristics. Nanoparticle additives were being added to mineral oil, synthetic oil and also to the vegetable oil bio-lubricants. Several researchers have tested the impact of nano additives lubrication related applications. Celik, Ay, and Goncu (Citation2013) examined the engine lubricating oil that had the coefficient of friction (COF) changed by adding hexagonal boron nitride (hBN) nanopowder. Jatti and Kumar (Citation2015) evaluated engine oil (mineral-based) by adding titanium dioxide nanoparticles. Tests were performed with .5 wt%, 1 wt%, 1.5 wt%, and 2 wt% of nanoparticles at loads of 40, 60, and 90 N, and sliding speeds of .5 m/s, 1.0 m/s, and 1.5 m/s. They observed that all the nano-lubricants had less wear and friction than basic oil. The anti-friction and anti-wear (AW) behavior was assumed to be caused by the deposition of soft TiO2 nanoparticles on the worn surface, which enhanced tribological characteristics by reducing shearing resistance. Asnida et al. (Citation2018) increased the longevity of the piston-liner contact by mixing copper oxide nanoparticles into regular engine oil. Copper oxide (CuO) nanoparticles were dispersed in SAE10W–30 to reduce wear and friction on the piston skirt. Raina and Anand (Citation2018) examined how the concentration of diamond nanoparticles affected the wear and friction characteristics of polyalphaolefin (PAO) oil. The nearly spherical form of the nanoparticles lessened wear loss and the coefficient of friction by smoothening sharp asperities and reducing sliding contact between interacting surfaces. The results of the investigation demonstrated that the lowest COF was found in PAO oil with .2 wt% diamond nanoparticles. Tang et al. (Citation2020) evaluated silver nanoparticles (Ag/BP) “black phosphorus (BP)” on PAO based oil by using ball-on-disc tribometer. Dispersing .075 wt% Ag/BP nanoadditives in PAO oil reduces friction by 73.4% and wear by 92.0% compared to using only PAO oil as the foundation.
Quite a good number of studies have been reported on the use of nano-sized metal and metal oxide nanoparticles on the conventional mineral and synthetic based lubricant. Studies concluded that optimum percentage of nanoparticles could lead to minimum wear and tear of rubbing surfaces. A few researchers have focused on the use of nanosized particles along with vegetable oil-based bio-lubricant that can be used in an IC engine lubrication and other machineries. Gulzar et al. (Citation2015) evaluated copper oxide (CuO) and molybdenum disulfide (MoS2) nanoparticles on modified palm oil. Their results showed that the anti-wear/extreme pressure characteristics of the MoS2 nanoparticles were superior to those of the CuO nanoparticles. Agglomerates could be reduced more easily when a surfactant with 1 wt% oleic acid content was added. Shafi and Charoo (Citation2020) investigated the rheological behavior of hazelnut oil mixed with nanoadditives of zirconium dioxide (ZrO2) at several concentrations (.5, 1.0, and 1.5 wt%). When 1.5 wt% of ZrO2 was added to hazelnut oil, the mixture’s viscosity reached its maximum (5.8%). Additionally, hazelnut oil had remarkable resistance to the rise in viscosity at lower temperatures, retaining its flowability even at very low temperatures. Singh, Chauhan, and Mamatha (Citation2020, Citation2020) examined the tribological characteristics of juliflora oil by the addition of TiO2 nanoparticles. The iodine value of juliflora oil dropped following chemical alteration, and the addition of nanoparticles assisted in raising the oil’s kinematic viscosity. The largest increase was seen at 1.2% nanoparticle addition. The addition of nanoparticles raised the flash point, with a concentration of .6% achieving the maximum. Tribological experiments demonstrated a substantial reduction in COF and pin wear with .6% of TiO2 nanoparticles. Razak et al. (2018) evaluated nano-clay (.02–.08 wt%) on palm oil (bio-lubricant) and mineral oil (20W–40) by using by four-ball tester using ASTM D4172–94. They found that the optimum concentration was .04 wt% nano-clay additive in palm oil, with a coefficient of friction of .081, which is 16% lower than mineral oil (20W–40). Roselina, Mohamad, and Kasolang (Citation2020) evaluated TiO2 nanoparticles (ranging from 0, .5, and 1.0 wt%.) on palm oil bio-lubricant and found that by using TiO2 as an additive, the viscosity index of the palm oil bio-lubricant was raised by 4.1%. Gulzar et al. (Citation2017) evaluated modified CuO nanoparticle suspensions on bio-based lubricant (Palm TMP ester). The test method used was fourball extreme pressure testing and sliding wear tests to assess wear protection. They found that the anti-wear/extreme pressure properties of the surface-modified nano-CuO enriched TMP ester were significantly better than those obtained without surfactant.
As per the literature survey, adding nanoparticles to mineral or synthetic oil improves its anti-friction properties while decreasing wear. There are few studies on adding nanoparticles to bio-lubricants originated from vegetable oil. Previous research has shown that adding nanoparticles at the right concentration increased the epoxidized oil’s dispersion and anti-wear properties. The kind of oil, the kind of nanoparticle, its concentration, and its size all affect the outcome. Therefore, extensive research is needed to fully establish the benefits of adding nanoparticles with surfactants to bio-lubricants in terms of their physiochemical and rheological characteristics, agglomeration, concentration, volume, and size change, etc. Further, on referring to the earlier published literature it is found that studies on influence of nanoparticle-based additives to the jojoba and jatropha based bio-lubricant are very limited. Further, studies related to the characterization of bio-lubricants derived from the above oils and the effect of nano additives on the tribological, heat transfer, wear, and friction characteristics are also inadequate.
Hence, to bridge the above gaps, jojoba and jatropha vegetable oil-based bio-lubricants are prepared by subjecting the raw oil to transesterification and epoxidation process. The multiwalled carbon nanotubes and titanium dioxide-based nanoparticles are added as functional additives to the synthesized bio-lubricant, and a series of tests have been conducted to examine the impact of the nanoparticles on thermal, tribological, and heat transport behavior. Results obtained with both types of nonadditive lubricants have been compared for optimum selection of the vegetable oil and nanoparticle combination for improved tribological performance in lubrication applications – is the novelty of the present study.
Methodology
Preparation of bio-lubricant
The raw non-edible oils such as Jatropha and Jojoba raw oils were purchased from vendors. Due to low thermal and oxidation stability, raw vegetable oils were not used directly as lubricants. These disadvantages were mitigated through chemical modifications such as: transesterification and epoxidation. H2SO4 and HCl were the two catalysts used for the study. The parameters such as reaction temperature, alcohol/methyl ester molar ratio and catalyst concentration are the most important factors influencing the epoxidation process. These factors were varied as shown in to analyze the effect of the above parameters on yield and thus, the optimal conditions for epoxidized jatropha and jojoba oil were determined. shows different parameters and their levels taken into consideration during chemical modification of the oils. Sixteen sets of experiments were conducted for each bio-lubricant (Jatropha and Jojoba with H2SO4 and HCl catalysts) for epoxidation process by varying the parameters independently.
Table 1. Main parameters and their levels considered for epoxidation reaction.
To prepare the epoxidized bio-lubricant, initially, the jatropha oil in a 3-neck bottom flask was heated and stirred using a magnetic stirrer at the rate of 600 rpm. The methanol and sodium hydroxide pellets were mixed in required quantities and stirred until pallets get dissolved. After dissolving the pellets, the mixture was poured into the 3-neck flat bottom flask and subjected to heating for half an hour. Afterwards, the mixture was poured in a separating funnel and glycerol and methyl ester separation was observed (). The transesterified oil was washed and taken in a beaker for epoxidation. The mixture of transesterified jatropha, peracetic acid and 30 ml/100 ml of catalyst concentration were agitated at room temperature for approximately 30 minutes. Hydrogen peroxide was added dropwise to the mixture and solution was heated for few hours. Diethyl ether was added to the solution and kept in a separating flask. Then an aqueous layer was formed at the bottom of the flask, which was drained as shown in . Then the solution was washed with cold water and warm water. Then two spatula of anhydrous sodium sulfate was added to the solution for removal of water content. Later, the epoxidized solution was filtered and stored in a beaker. Similar procedure was followed to modify raw jojoba oil.
The best optimized yield for jatropha bio-lubricant with H2SO4 and HCl catalysts was obtained with molar ratio .5:1.5, temperature 70°C and catalyst concentration at .48 ml/100 ml. The best optimized yield for jojoba bio-lubricant with H2SO4 & HCl catalysts was obtained with molar ratio .5:1.5, temperature 60°C and catalyst concentration at .48 ml/100 ml. The physico-chemical properties of the raw oils and the prepared bio-lubricants are shown in .
Table 2. Physicochemical properties of jatropha and jojoba bio-lubricants (Cecilia et al. Citation2020; Mushtaq and Hanief Citation2021) and (Hassan, Hassan, and Youssif Citation2019.).
In order to determine the property variations of the prepared samples, iodine test, TGA and wear study by using TRB3 Ball on Disk tribometer were performed and the results have been discussed in the following sections. shows the uncertainty involved in testing the parameters using each of the instruments.
Table 3. Uncertainty of the instruments used in the study.
Results and discussions
Iodine value
The amount of iodine needed to saturate 100 grams of oil or fat is known as the iodine value. The iodine value of fatty acids is correlated with the number of double bonds. This characteristic, which only depends on the source of the vegetable oil, describes the quantity of unsaturated fatty acids present. Due to the fact that a high concentration of unsaturated fatty acids leads to the polymerization of the glycerides, an unsaturated fatty acid restriction may be required. This may cause deposits to build or the lubricating oil to deteriorate. The number of double bonds in the fatty acid chain has an impact on this effect. Therefore, limiting the amount of more unsaturated fatty acids, such as linolenic acid, is preferable to limiting the level of unsaturation, which is determined by the iodine number. As a result, the value of iodine rises as the number of double bonds grows. Compared to saturated fatty acids, unsaturated fatty acids have more double bonds. The iodine value of raw jatropha and jojoba oil is reduced as a result of chemical processing. It is calculated as
Eq. wt. of iodine = 127 g/mol
Vol. of Sodium thiosulfate = V blank - V test
where Vblank = Volume of blank sample
Vtest = Volume of test sample
Normality of Sodium thiosulfate = 0.1
The above procedure is repeated and the iodine values for all the combinations of the bio-lubricant are shown in .
Table 4. Iodine values for different set of test samples.
The efficiency of epoxidation reaction was determined by iodine value, which is the number of grams of iodine absorbed per 100 grams of oil sample. Based on the above results, raw jatropha and jojoba oils have higher value of IV than epoxidized oils, showing that oil is unsaturated – which implies carbon–carbon double bonds are more in number. Through epoxidation, epoxidized oils have undergone a chemical reaction in which the double bonds in the fatty acid chains have been converted into epoxy group. Consequently, it leads to the replacement of unsaturated bonds by oxirane ring – resulting in the decrement of iodine value. From the above table, the chemical modified jatropha, i.e., epoxidized jatropha with H2SO4 and HCl catalyst showed Iodine value 5 and 8 gI2/100 g, respectively. Epoxidized jojoba showed IV 6 and 9 gI2/100 g for H2SO4 and HCl catalyst respectively which are far less as compared to that of neat vegetable oils. This confirms the suitability of the modified vegetable oils for lubrication purposes.
Thermogravimetric analysis
The temperature at which oil begins to break down is known as the onset temperature, which is used to measure thermal stability. To understand the decomposition temperature of the bio-lubricant within the operating temperature range of the experiments, thermogravimetric analysis (TGA) is very useful. A quick, dependable, and affordable method for analyzing the thermal stability of oil is the TGA, which measures changes in the material’s weight and enthalpy in relation to temperature and heating rate. Besides, TGA has the advantage of being a highly precise and sensitive technique requiring only a small amount of sample. The TGA-4000 apparatus from PerkinElmer was used to conduct the experiments, which had a nitrogen gas environment and a flow rate of 30 ml/min. The samples weighed between 30 and 40 mg when they were put inside quartz crucibles. All samples were heated at a rate of 20°C per minute when the measurements were first conducted at room temperature and continued to 900°C. TGA curves showed how the weight of vegetable oil lost (%) as a function of temperature. The temperature curve of a TGA is shown from left to right. A decrease in weight is indicated by the TGA thermal curve’s descent. When decomposition occurs in a nitrogen atmosphere, the onset temperature is utilized to quantify the degree of thermal degradation. Most TGA experiments use an inert gas. Thermal Stability data of different samples based on Onset of degradation is shown in .
Table 5. Thermal stability data of different samples based on onset of degradation.
During TGA, a sample is heated at a constant rate while its weight is continuously monitored. As the temperature increases, various chemical and physical processes can occur, leading to changes in the sample’s weight. In TGA, the first weight loss typically corresponds to the removal of physically absorbed or loosely bound water molecules from a sample as it is heated. This initial weight loss is often referred to as the “dehydration step” and can provide valuable information about the moisture content and thermal stability of a sample. The first weight loss was 61.40%, 62.00%, 60.56%, 57.98%, 55.52%, and 53.76% for JA, JO, EJA -H2SO4, EJA – HCl, EJO -H2SO4 and EJO – HCl, respectively.
The second weight loss in TGA typically corresponds to more significant chemical processes occurring within the sample as it continues to be heated. This weight loss indicates the decomposition, degradation, or other chemical transformations of the sample. Just like the first weight loss, the nature of the second weight loss depends on the type of sample being analyzed. The temperature range at which the first weight loss and second weight loss occurs can also provide insights into the thermal stability of the sample and any potential decomposition or degradation processes that might be taking place. The second weight loss was found to be 32.41%, 37.99%, 39.43%, 34.18%, 44.47%, and 46.23% for JA, JO, EJA -H2SO4, EJA – HCl, EJO -H2SO4 and EJO – HCl, respectively.
In TGA of oils, the third weight loss, if present, could be indicative of more complex processes occurring within the oil sample as it is subjected to increasing temperatures. Oil samples are complex mixtures of various hydrocarbons and other compounds, and their behavior during TGA can depend on factors such as the specific type of oil, impurities, additives, and environmental conditions. The third weight loss could represent the decomposition of these more complex components that start breaking down at higher temperatures. In some cases, especially with heavier oils, catalytic cracking reactions can occur at elevated temperatures. These reactions involve the breaking of larger hydrocarbon molecules into smaller ones and can lead to weight loss.
The absence of ash content in TGA is indeed a common scenario, especially when analyzing oil samples. In TGA, the absence of ash content is typically indicated by the absence of a significant weight loss or residue at higher temperatures. Ash content refers to the inorganic residue left behind after complete combustion of a material. In TGA, absence of ash content means there is not a significant weight loss at higher temperatures while analyzing the oil samples, which generally indicates that there’s minimal or no inorganic ash content in the sample. If there is any residue left behind at higher temperatures, it suggests the presence of inorganic components that are not easily volatilized or decomposed under the conditions of the TGA analysis indicating presence of ash content. From our experimental results, no ash content was observed for all samples subjected under TGA. show the TGA for neat JA and JO respectively, show the TGA for EJA-H2SO4 and EJO-HCl, respectively.
From the , the decomposition temperature of jatropha and jojoba raw oil are 168°C and 178°C, respectively. The corresponding ring opening products of samples 3, 4, 5, and 6 are thermally stable below the temperature of 238°C, 224°C, 230°C, and 244°C respectively as seen from . So, the best combination for thermal degradation for jatropha is obtained for EJA-H2SO4 and for jojoba its EJO-HCl as shown in , respectively. This shows the improved thermal stability of epoxidized oils over their neat forms. Epoxide was expected to have higher heat stability than unmodified oils because during epoxidation, every double bond was changed into an oxirane ring. The presence of a significant number of saturated fatty acids is what significantly contributes to the improved thermal stability of the biodegradable oil, which results in the higher degradation temperature of the epoxidized methyl esters. This recommends use of the epoxidized jojoba and jatropha bio-lubricants to high-temperature applications such as IC engines. The major factors influencing oil breakdown are its chemical composition and the free fatty acid compositions. Meanwhile, the fatty acid’s chain length, branching pattern, and level of unsaturation all play a significant role in the oil’s thermal stability. In contrast to raw oils, epoxidized methyl esters demonstrated greater heat stability. Out of the various combination of epoxidized oils tested, jatropha oil with sulfuric acid catalyst and jojoba oil with hydrochloric acid catalyst combination are superior as compared to the others, due to their high values of onset degradation temperature and also due to their minimum first weight loss values.
Characterization of the bio-lubricant sample with nanoparticle additive
Jatropha and Jojoba bio-lubricant samples were prepared with H2SO4 and HCl catalyst. Multiwalled carbon nanotubes (MWCNT) and TiO2 nanoparticles were used for the study. The MWCNT nanoparticles of 1 wt% and TiO2 nanoparticles of 1 wt% were added to prepared jatropha and jojoba bio-lubricants and sonicated using Triton X-100 surfactant. In a variety of applications, ultrasonic probe sonicators are frequently employed to stop agglomeration or agglomerate dispersion. In the current study, an ultrasonic probe sonicator was employed to prevent MWCNT and TiO2 nanoparticles from clumping together in produced jatropha and jojoba bio-lubricant samples. After subjecting the samples for the sonication process under ultrasonic probe sonicator equipment for a period of 30 minutes, good dispersion of nanoparticles was observed for both types of bio-lubricants. The experimental conditions and the level of dispersion after addition of nanoparticles to sample is shown in .
Table 6. Dispersion analysis of nanoparticle and TritonX-100 surfactant combinations.
UV-visible spectroscopy
Spectrophotometry, another name for UV-Visible Spectroscopy, is a quantitative technique for determining the amount of light that a single molecule absorbs. To do this, the amount of light passing through a sample is compared to the amount of light passing through a blank or reference sample. The concept that chemical substances have the ability to absorb ultraviolet or visible light and produce distinctive spectra as a result is the basis of UV-Visible Spectroscopy. In current study, the dispersion properties of the nanoparticles were studied using UV–Visible spectrometer Shimadzu−1800 model. The MWCNT and TiO2 nanoparticles dispersion in the methyl ester of epoxidized jatropha and jojoba was examined using a UV spectrometer. The Dispersibility of MWCNT and TiO2 nanoparticles in Epoxidized Jatropha Methyl Ester is shown in . The Dispersibility of MWCNT and TiO2 nanoparticles in Epoxidized Jojoba Methyl Ester is shown in . The spectra exhibited between 350 and 800 nm range were studied. Wavelengths of Jatropha and jojoba methyl ester with MWCNT and TiO2 nanoparticles are shown in , respectively.
Table 7. Wavelengths of jatropha methyl ester with MWCNT and TiO2 nanoparticles.
Table 8. Wavelengths of jojoba methyl ester with MWCNT and TiO2 nanoparticles.
Bio-lubricants, which are environmentally friendly alternatives to conventional lubricants, often contain various organic compounds that can absorb UV light. Absorbance units (AU) are a unit of measurement used in UV spectroscopy to indicate how much light a sample absorbs at a given wavelength. More light is absorbed by the sample at a given wavelength when the absorbance is higher. Using visible spectroscopy, the absorbance level of visible light was measured over time. This absorbance level is proportional to the dispersion of nano particles in the lubricant sample. shows the spectra of MWCNT nano-lubricants, showing a peak at wavelength of 368 nm (λmax) and absorbance of 2.76. A greater distribution of the nanoparticles within the epoxidized methyl esters is suggested by the higher peak of absorbance level. The reason for high absorbance values with MWCNT nanoparticles in samples are mainly due to overlapping absorption bands. Sometimes, multiple absorbing species in the sample can have absorption bands that overlap, leading to combined effects that result in high absorbance values. From , a peak at wavelength of 352 nm (λmax) was observed in the spectrum of TiO2 nano-lubricants with absorbance being 3.51. With TiO2 nanoparticles, there is possibility of interference in the sample that might absorb light at the selected wavelength. These interferences could contribute to the high absorbance values. Therefore, from current study, Epoxidized Jatropha with H2SO4 catalyst – MWCNT nanoparticles and Jojoba with HCl catalyst – TiO2 nanoparticles showed a better dispersion with time than the rest of the samples which may result in better physicochemical properties compared with the rest of the samples.
Viscosity
A rheometer is a scientific instrument used to measure the rheological properties of materials, particularly their flow and deformation behavior. Rheology is the study of how materials respond to applied forces or stresses and how they flow or deform under different conditions. The relationship between shear stress (τ) and viscosity (η) can be described by Newton’s law of viscosity as:
where: τ = Shear stress (measured in Pa or N/m2)
η = Viscosity (measured in Pa·s or N·s/m2)
γ= Shear rate (measured in s− 1 or 1/s)
The nanoparticles are homogeneously distributed in the fluid through the ultrasonication process for 30 minutes. The rheology of all samples is studied using Anton Par MCR 92 Rheometer as shown in at temperatures ranging from −10°C to 80°C. represents jatropha raw oil sample kept for testing.
The lubricant’s intermolecular forces causes viscosity. To put it another way, raising the temperature of the lubricant results in increased molecular potential energy and correspondingly lower intermolecular forces. In this study, the temperature-dependent viscosity of lubricants has been studied for the various prepared bio-lubricant samples. Most bio-lubricants exhibit high viscosity, and in ester bio-lubricants, the hydrocarbon chain length of the carboxylic acid or alcohol directly correlates with the viscosity. Through chemical modifications, hydroxyl groups are added to fatty acids, which increases the viscosity by increasing hydrogen bond interactions. shows temperature-dependent viscosity of the four variants of jatropha oil, i.e., JA, EJA-H2SO4, EJA-H2SO4-MWCNT, and EJA-H2SO4-TiO2 which indicates that the EJA-H2SO4-MWCNT bio-lubricant has higher viscosity in comparison with rest of the jatropha oils. In particular, the EJA-H2SO4-MWCNT in low-temperature field exhibits very high viscosity, which is the starting viscosity of the engine. In other words, more beginning torque will be needed when using the EJA-H2SO4-MWCNT for lubrication. show that the epoxidation increases the viscosity of the EJA-H2SO4 and EJA-HCl catalysts in comparison to the raw JA. Viscosity is increased as a result of the chemical conversion of unsaturated fatty acids into saturated fatty acids. The formation of the long-chain molecular structure increases viscosity. The modified oil’s viscosity is further increased by the inclusion of MWCNT and TiO2 nanoparticles. The rise in nanoparticle density encouraged their aggregation on surfaces, which encouraged molecular collision and increased viscosity. MWCNT showed slightly higher value than TiO2 based lubricant. This gentle rising trend may be due to the tubular form of MWCNT against to spherical shape of TiO2 nanoparticles. It is also clear from , that the viscosity reduces for MWCNT and TiO2 nanoparticles for both H2SO4 and HCl catalysts with increasing temperature from 30°C to 80°C. This is an expected result and is caused by weakening of the van der Waals forces between the molecules. As compared to their raw forms and epoxidized forms viscosity of the nanoparticle added lubricant was found to be more stable with huge range of temperatures, which is one of the important considerations for a good lubricant. Moreover, thermal stability of surfactant solution (Triton X-100) also plays an important role in dispersion of MWCNT and TiO2 nanoparticles. Usually, the addition of MWCNT nanoparticle creates poor solubility and agglomeration in the prepared bio-lubricant samples. So, to overcome this drawback, Triton X-100 was added to the MWCNT bio-lubricant sample. Regardless of addition of the surfactant, from it was seen that MWCNT showed slightly higher viscosity than the TiO2 samples because in agglomerated condition, the MWCNT nano-sized particles accumulate to form irregular shape micron size particle which results in more viscosity, hence further affecting the tribological properties of the bio-lubricant samples.
Figure 11. Temperature-dependent viscosity for JA, EJA-H2SO4,EJA - H2SO4- TiO2and EJA - H2SO4- MWCNT.
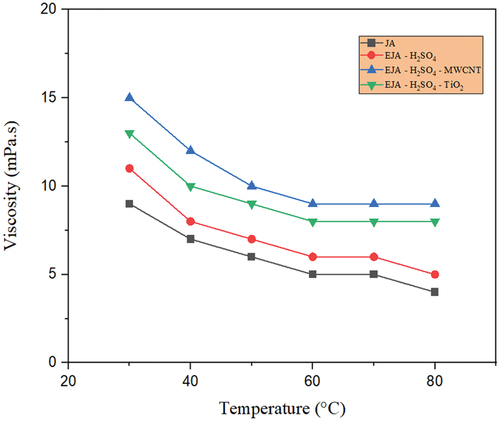
Similarly, from , it is revealed that the viscosity of the modified jojoba oil with both H2SO4 and HCl catalyst in comparison to the raw jojoba oil increases due to epoxidation. From , as MWCNT nanoparticles have a high surface area-to-volume ratio, leading to increased interparticle interactions. These interactions can create networks or clusters of particles within the fluid, resulting in higher resistance to flow and an increase in viscosity in jojoba bio-lubricant. These MWCNT nanoparticles could agglomerate or cluster together due to attractive forces between them. These agglomerates can act as larger particles, increasing the effective particle size and resulting in a higher viscosity in both EJO-H2SO4-MWCNT and EJO-HCl-MWCNT bio-lubricant samples. The addition of TiO2 nanoparticles to the epoxidized jojoba with H2SO4 and HCl catalyst was the better combination because TiO2 nanoparticles experience significant Brownian motion by preventing particles from settling down, leading to the stability of colloidal nanoparticle solutions.
Wear and friction tests
The anti-wear and friction-reduction performance were evaluated on Nanoeva’s ST400 non-contact profilometer and Anton Paar’s TRB3 ball on disk. Anton Paar’s TRB3 ball on disk is shown in . Nanoeva’s ST400 non-contact profilometer uses mountains map surface analysis software for calculating wear rate, wear depth, and worn track section. Anton Paar’s TRB3 ball on disk uses Tribometer – InstrumX 9..16 version software for calculation COF. The measuring principle used for calculation of friction in Anton Paar’s TRB3 ball on disk is that the friction force is proportional to normal load and is independent of sliding velocity. The tangential force required to overcome friction is directly proportional to the normal force and the coefficient of friction. The COF is calculated by using the following equation:
Ft = Tangential force (force that acts in a direction that is tangent to a surface)
Fn= Normal force (acts perpendicular to the surface)
The tests were conducted on Al4032 alloy (procured from Jicon steels, Mumbai) at room temperature (298 K) under an applied load of 20N. The test conducted complies with the ASTM G99 and ASTM G133 standards. The wear test samples of Al4032 alloy were cut using wire EDM machine. To get the mirror finish for the Al4032 samples, grit papers of 400,1000,1500 and 2000 microns were used and the surfaces were polished. The steel ball, with a diameter of 12.7 mm with an HRC of 60–65 was used for wear studies. The principle of working is shown in , wherein a spherical ball (typically made of a hard material like steel) is pressed against a flat, rotating disk under controlled conditions. The ball rolls on the disk surface, and the frictional forces and wear rates are measured. The prepared bio-lubricant sample is poured into a cup holder which has an Al4032 sample in it. The operating conditions considered for each sample during the operating period of the machine are given in . The comparative analysis has been made for different lubricants (jatropha and jojoba) in terms of wear and COF. Various prepared lubricant samples EJA-H2SO4, EJA-HCl, EJO-H2SO4, EJO-HCl, EJA-H2SO4-MWCNT, EJA-HCl-MWCNT, EJA-H2SO4-TiO2, EJA-HCl-TiO2, EJO-H2SO4-MWCNT, EJO-HCl-MWCNT, EJO-H2SO4- TiO2 and EJO-HCl- TiO2 are tested under standard operating conditions as shown in . COF and wear Rate of the samples are shown in .
Table 9. Parameters considered for COF and wear rate analysis.
Table 10. COF and wear rate of the samples.
Of the different samples tested, neat epoxidized oils showed maximum wear rate as shown in . With the addition of TiO2 nanoparticles, the wear rate was considerably reduced for all tested samples. EJO-HCl-TiO2 combination showed the lowermost wear rate which is 94.68% lower than the neat oil combination (without the addition of nanoparticles). A similar trend has been observed for the MWCNT nanoparticle-added samples. The maximum wear rate was obtained for EJO-H2SO4, and the minimum was obtained for EJA- H2SO4 -MWCNT sample, which was 79.85% lesser than its neat form. EJO-HCl-TiO2 and EJA-H2SO4-MWCNT, had the less wear rate and COF among all the samples. The percentage reduction in wear for TiO2 nanoparticle samples in comparison with epoxidized samples are 82.26%, 83.71%, 71.16%, and 94.68% for EJA-H2SO4-TiO2, EJA-HCl-TiO2, EJO-H2SO4-TiO2, and EJO-HCl-TiO2, respectively. With the addition of MWCNT nanoparticles − 79.85%, 57.29%, 71.78%, and 41.96% reductions in wear were observed for EJA-H2SO4-MWCNT, EJA-HCl-MWCNT, EJO-H2SO4-MWCNT and EJO-HCl-MWCNT samples, respectively.
3-D view of the surface and worn track section (to know wear depth) of the samples have been plotted for all the bio-lubricant samples. The development of the protective layer between the surfaces in contact determines the friction coefficient and wear during oil use. Nevertheless, as compared to lubricants with nanoparticle addition, the epoxidized esters exhibit poor wear properties and a low auto-ignition temperature. Compared to samples that have nanoparticles added, epoxidized samples often have a higher wear rate and COF. The epoxidized esters were combined with a nanoparticle addition to increase these qualities, which resulted in an improvement in the wear property and COF. This could be explained by the nanoparticles adhering to the ball’s surface and creating a robust shield between the oil, the nanoparticles, and the surfaces. From , it is also evident that COF decreased with nanoparticle additives as compared to epoxidized samples. The COF of EJA-H2SO4-MWCNT and EJO-HCl-TiO2 sample was found to be .061 and .059, respectively. show the 3-D view of the EJA-H2SO4-MWCNT and EJO-HCl-TiO2 samples, respectively. The wear rate was found to be 9.552 × 10−7 and 1.587 × 10−7 mm3/Nm for EJA-H2SO4-MWCNT and EJO-HCl-TiO2, respectively. From the , cross-section of the sample is plotted to know the wear depth of the sample (worn out area). Among all the samples, EJO-HCl – TiO2 and EJA-H2SO4 – MWCNT have the least wear depth, i.e., 18.11 and 3.907 µm, respectively.
Figure 19. (a) 3Dview of the EJA-H2SO4-MWCNTsample. (b) Cross-section of the EJA-H2SO4-MWCNT sample.
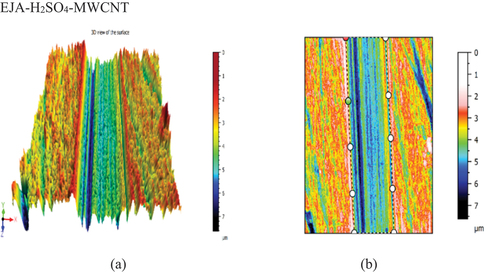
TiO2 nanoparticles enhance the thermal conductivity of the lubricant. This helps to dissipate heat more efficiently from the contact region, reducing the risk of thermal degradation and hence wear. TiO2 nanoparticles can modify the viscosity of the lubricant, making it more stable and effective at various temperatures and pressures compared to epoxidized samples prepared. This ensures consistent lubrication and protection. TiO2 nanoparticles can help the lubricant to form a stronger and more durable oil film between surfaces by preventing metal-to-metal contact and reducing wear. Hence, minimum wear rate and COF have been observed as compared with all the samples. MWCNT nanoparticles can enhance the load-bearing capacity of the lubricant. This means that these nanoparticles can withstand and distribute the forces between moving components, reducing direct metal-to-metal contact and wear. The prepared epoxidized samples, after treatment with MWCNT nanoparticles, helped in reducing friction between contacting surfaces through mechanisms like rolling or sliding by preventing direct rubbing against each other. MWCNT nanoparticles can fill in surface imperfections and irregularities, effectively creating a smoother surface for Al4032 alloy sample to glide upon, making surface roughness minimal. The tubular structure of the MWCNT nanoparticles resulted in a slightly higher wear rate and COF as against the TiO2 particles, which are usually spherical in nature. Further, the best combination also reveals that chemical modification of the base vegetable oil is also a deterministic factor in reducing wear and friction.
Conclusions
Characterization of the bio-lubricant samples have been done with nanoparticle as an additive. Jatropha and Jojoba are the two types of non-edible oils, made to react with catalysts H2SO4 and HCl. Triton X-100 surfactant, Multiwalled carbon nanotubes (MWCNT) and TiO2 nanoparticles were used for the study. The following conclusions are drawn from the study.
The iodine value was found to be 110 and 85 gI2/100 g for raw jatropha and jojoba oil respectively. The chemical modification of the jatropha and jojoba oil results in a decrease of iodine value resulting in breaking of carbon bonds. The IV values for EJA-H2SO4, EJA-HCl, EJO-H2SO4 and EJO-HCl samples were 5,8,9 and 6 gI2/100 g respectively.
TGA measurements showed that the epoxidized methyl esters have higher degradation temperatures, which causes the increased thermal stability of the biodegradable oil. Thermal degradation temperatures for EJA-H2SO4, EJA-HCl, EJO-H2SO4, and EJO-HCl samples are 238°C, 224°C, 230°C, and 244°C, respectively.
The nanoparticle-added bio-lubricant samples were tested for dispersion properties using UV the visible spectrometer Shimadzu − 1800 model. EJA-H2SO4-MWCNT and EJO-HCl-TiO2 samples absorbed maximum light resulting in better dispersion stability.
The viscosity values after the addition of the nanoparticles were found to be consistent with minimum variation in the temperature range studied. However, MWCNT samples showed slightly higher viscosity than the TiO2 based samples. Among all the samples, EJO – H2SO4 - MWCNT sample showed maximum viscosity at all temperature ranges.
The percentage reduction in wear for TiO2 nanoparticle samples in comparison with epoxidized samples are 82.26%, 83.71%, 71.16%, and 94.68% for EJA-H2SO4-TiO2, EJA-HCl-TiO2, EJO-H2SO4-TiO2, and EJO-HCl-TiO2 respectively.
With addition of MWCNT nanoparticle − 79.85%, 57.29%, 71.78%, and 41.96% reduction in wear were observed for EJA-H2SO4-MWCNT, EJA-HCl-MWCNT, EJO-H2SO4-MWCNT, and EJO-HCl-MWCNT samples, respectively.
The COF of EJA-H2SO4-MWCNT and EJO-HCl-TiO2 sample was found to be .061 and .059, respectively. Among all the samples, EJO-HCl-TiO2 and EJA-H2SO4-MWCNT have the least wear depth, i.e., 18.11 and 3.907 µm, respectively.
Future research work includes bio-lubricants derived from different types of nonedible oils could be compared, and the corresponding yield could be optimized. Investigation of various ways for modifying the stability of nanoparticles in base oils to produce lubricants that exhibit both chemical and physical stability could be undertaken. Different types of nanoparticles, their volume fractions, and size could be investigated for optimum tribological performance. Investigation of effect of nano additive toxicity on the human health needs to be addressed.
Limitations of the study include vegetable oils have ester functionality groups, which make them prone to hydrolytic breakdown, it is imperative to avoid water contamination at all stages. Additionally, vegetable oils have poor corrosion prevention, particularly in the presence of moisture. The price of the bio-lubricants presents another significant obstacle. The cost of bio-lubricant is approximately 30–40% higher than that of conventional lubricant. Authors have considered the single uniform size of the nanoparticles during the study. Influence of size of the nanoparticles on the tribological behavior needs to be investigated.
Nomenclature
JA | = | Jatropha raw oil |
JO | = | Jojoba raw oil |
H2SO4 | = | Sulfuric acid |
HCl | = | Hydrochloric acid |
MWCNT | = | Multi-walled carbon nanotubes |
TiO2 | = | Titanium dioxide |
NaOCH3 | = | Sodium methoxide |
TMP | = | Trimethylolpropane |
hBN | = | Hexagonal boron nitride |
CuO | = | Copper oxide |
PAO | = | Polyalphaolefin |
MoS2 | = | Molybdenum disulfide |
SiO2 | = | Silicon dioxide |
TGA | = | Thermogravimetric analysis |
EJA-H2SO4 | = | Epoxidized jatropha methyl ester with H2SO4 catalyst |
EJA-HCl | = | Epoxidized jatropha methyl ester with HCl catalyst |
EJO-H2SO4 | = | Epoxidized jojoba methyl ester with H2SO4 catalyst |
EJO-HCl | = | Epoxidized jojoba methyl ester with HCl catalyst |
EJA-H2SO4-MWCNT | = | Epoxidized jatropha methyl ester with H2SO4 catalyst and MWCNT nanoparticle |
EJA-HCl-MWCNT | = | Epoxidized jatropha methyl ester with HCl catalyst and MWCNT nanoparticle |
EJA-H2SO4-TiO2 | = | Epoxidized jatropha methyl ester with H2SO4 catalyst and TiO2 nanoparticle |
EJA-HCl-TiO2 | = | Epoxidized jatropha methyl ester with HCl catalyst and TiO2 nanoparticle |
EJO-H2SO4-MWCNT | = | Epoxidized jojoba methyl ester with H2SO4 catalyst and MWCNT nanoparticle |
EJO-HCl-MWCNT | = | Epoxidized jojoba methyl ester with HCl catalyst and MWCNT nanoparticle |
EJO-H2SO4-TiO2 | = | Epoxidized jojoba methyl ester with H2SO4 catalyst and TiO2 nanoparticle |
EJO-HCl-TiO2 | = | Epoxidized jojoba methyl ester with HCl catalyst and TiO2 nanoparticle |
Acknowledgements
The authors would like to acknowledge Manipal Academy of Higher Education, Manipal for providing financial assistance through Intra Mural fund (Ref: MAHE/CDS/PHD/IMF/2019) for the Ph.D. work.
Disclosure statement
No potential conflict of interest was reported by the authors.
Additional information
Funding
Notes on contributors
Rajendra Uppar
Rajendra Uppar is a full-time research scholar in the Department of Mechanical and Industrial Engineering at Manipal Institute of Technology Manipal. Manipal Academy of Higher Education, Manipal, India.
P. Dinesha
P. Dinesha is a Professor in the Department of Mechanical and Manufacturing Engineering, at Manipal Institute of Technology, Manipal Academy of Higher Education, Manipal, India. He obtained a doctoral degree from the National Institute of Technology Karnataka, Surathkal, India in the area of alternative fuels. His research interests include engine combustion, alternative fuels, pollution, and biomass waste management, biolubricants etc.
Shiva Kumar
Shiva Kumar is a Professor in the Department of Mechanical and Manufacturing Engineering, at Manipal Institute of Technology, Manipal Academy of Higher Education, Manipal, India. He obtained a doctoral degree in the area of internal combustion engines from Visvesvaraya Technological University, Belagavi, India. His research interests include engine combustion, alternative fuels, pollution, biolubricants,heat transfer, CFD studies, and nanofluids.
References
- Adhvaryu, A., and S. Z. Erhan. 2002. Epoxidized soybean oil as a potential source of high-temperature lubricants. Industrial Crops and Products 15 (3):247–54. doi:10.1016/S0926-6690(01)00120-0.
- Aguilera, A. F., P. Tolvanen, K. Eränen, J. Wärnå, S. Leveneur, T. Marchant, and T. Salmi. 2019. Kinetic modelling of prileschajew epoxidation of oleic acid under conventional heating and microwave irradiation. Chemical Engineering Science 199:426–38. doi:10.1016/j.ces.2019.01.035.
- Asnida, M., S. Hisham, N. W. Awang, A. K. Amirruddin, M. M. Noor, K. Kadirgama, D. Ramasamy, G. Najafi, and F. Tarlochan. 2018. Copper (II) oxide nanoparticles as additive in engine oil to increase the durability of piston-liner contact. Fuel 212:656–67. doi:10.1016/j.fuel.2017.10.002.
- Aziz, N. A. M., R. Yunus, U. Rashid, and A. M. Syam. 2014. Application of response surface methodology (RSM) for optimizing the palm-based pentaerythritol ester synthesis. Industrial Crops and Products 62:305–12. doi:10.1016/j.indcrop.2014.08.040.
- Bart, J. C. J., E. Gucciardi, and S. Cavallaro. 2013. 1 - renewable lubricants, Biolubricants, 1–9. UK: Woodhead Publishing.
- Cecilia, J. A., D. Ballesteros Plata, R. M. Alves Saboya, F. M. Tavares de Luna, C. L. Cavalcante Jr, and E. Rodríguez-Castellón. 2020. An overview of the biolubricant production process: Challenges and future perspectives. Processes 8 (3):257. doi:10.3390/pr8030257.
- Celik, O. N., N. Ay, and Y. Goncu. 2013. Effect of nano hexagonal boron nitride lubricant additives on the friction and wear properties of AISI 4140 steel. Particulate Science and Technology 31 (5):501–06. doi:10.1080/02726351.2013.779336.
- Doll, K. M., S. C. Cermak, J. A. Kenar, E. L. Walter, and T. A. Isbell. 2017. Derivatization of castor oil based estolide esters: Preparation of epoxides and cyclic carbonates. Industrial Crops and Products 104:269–77. doi:10.1016/j.indcrop.2017.04.061.
- Goud, V. V., A. V. Patwardhan, and N. C. Pradhan. 2006. Studies on the epoxidation of mahua oil (Madhumica Indica) by hydrogen peroxide. Bioresource Technology 97 (12):1365–71. doi:10.1016/j.biortech.2005.07.004.
- Goud, V. V., A. V. Patwardhan, and N. C. Pradhan. 2007. Kinetics of in situ epoxidation of natural unsaturated triglycerides catalysed by acidic ion exchange resin. Industrial & Engineering Chemistry Research 46 (10):3078–85. doi:10.1021/ie060146s.
- Gulzar, M., H. H. Masjuki, M. A. Kalam, M. Varman, and N. W. M. Zulkifli. 2017. Antiwear behavior of CuO nanoparticles as additive in bio-based lubricant. Key Engineering Materials 748:166–170. doi:10.4028/www.scientific.net/KEM.748.166.
- Gulzar, M., H. H. Masjuki, M. Varman, M. A. Kalam, R. A. Mufti, N. W. M. Zulkifli, R. Yunus, and R. Zahid. 2015. Improving the AW/EP ability of chemically modified palm oil by adding CuO and MoS2 nanoparticles. Tribology International 88:271–79. doi:10.1016/j.triboint.2015.03.035.
- Hassan, A., M. T. Hassan, and A. Youssif. 2019. Production of bio lubricant from jojoba oil. International Journal of Engineering Innovative Research 8 (4):146–53.
- Heikal, E. K., M. S. Elmelawy, S. A. Khalil, and N. M. Elbasuny. 2017a. Manufacturing of environment friendly biolubricants from vegetable oils. Egyptian Journal of Petroleum 26 (1):53–59. doi:10.1016/j.ejpe.2016.03.003.
- Heikal, E. K., M. S. Elmelawy, S. A. Khalil, and N. M. Elbasuny. 2017b. Manufacturing of environment friendly biolubricants from vegetable oils. Egyptian Journal of Petroleum 26 (1):53–59. doi:10.1016/j.ejpe.2016.03.003.
- Jatti, V. K. S., and V. Kumar. 2015. Titanium oxide nanoparticles as anti-wear and friction-reduction additives in lubricating oil. Journal of Chemical and Pharmaceutical Research 7 (4):1049–55.
- Koh, M. Y., T. I. M. Ghazi, and A. Idris. 2014. Synthesis of palm based biolubricant in an oscillatory flow reactor (OFR). Industrial Crops and Products 52:567–74. doi:10.1016/j.indcrop.2013.10.042.
- Mannekote, J. K., S. V. Kailas, K. Venkatesh, and N. Kathyayini. 2018. Environmentally friendly functional fluids from renewable and sustainable sources-A review. Renewable and Sustainable Energy Reviews 81:1787–1801. doi:10.1016/j.rser.2017.05.274.
- McNutt, J. 2016. Development of biolubricants from vegetable oils via chemical modification. Journal of Industrial and Engineering Chemistry 36:1–12. doi:10.1016/j.jiec.2016.02.008.
- Mushtaq, Z., and M. Hanief. 2021. Evaluation of tribological performance of jatropha oil modified with molybdenum disulphide micro-particles for steel–steel contacts. Journal of Tribology 143 (2):129–43. doi:10.1115/1.4047752.
- Raina, A., and A. Anand. 2018. Lubrication performance of synthetic oil mixed with diamond nanoparticles: Effect of concentration. Materials Today: Proceedings 5 (9):2058–94. doi:10.1016/j.matpr.2018.06.438.
- Roselina, N. R., N. S. Mohamad, and S. Kasolang. 2020, April. Evaluation of TiO2 nanoparticles as viscosity modifier in palm oil bio-lubricant. IOP Conference Series: Materials Science & Engineering 834 (1):012032. ( IOP Publishing). doi: 10.1088/1757-899X/834/1/012032.
- Salih, N., J. Salimon, and E. Yousif. 2012. Synthetic biolubricant basestocks based on environmentally friendly raw materials. Journal of King Saud University-Science 24 (3):221–26. doi:10.1016/j.jksus.2011.02.003.
- Salimon, J., N. Salih, and E. Yousif. 2010. Biolubricants: Raw materials, chemical modifications, and environmental benefits. European Journal of Lipid Science and Technology 112 (5):519–30. doi:10.1002/ejlt.200900205.
- Schneider, M. P. 2006. Plant‐oil‐based lubricants and hydraulic fluids. Journal of the Science of Food and Agriculture 86 (12):1769–1780. doi:10.1002/jsfa.2559.
- Seela, C. R., B. Ravisankar, and B. M. V. A. Raju. 2018. A GRNN based frame work to test the influence of nano zinc additive biodiesel blends on CI engine performance and emissions. Egyptian Journal of Petroleum 27 (4):641–647. doi:10.1016/j.ejpe.2017.09.006.
- Shafi, W. K., and M. S. Charoo (2020). Rheological properties of hazelnut oil mixed with zirconium dioxide nanoparticles. Materials Today Proceedings, 26, 745–49.
- Sharma, R. V., and A. K. Dalai. 2013. Synthesis of bio-lubricant from epoxy canola oil using sulfated Ti-SBA-15 catalyst. Applied Catalysis B Environmental 142:604–14. doi:10.1016/j.apcatb.2013.06.001.
- Singh, A., P. Chauhan, and T. G. Mamatha (2020). A review on tribological performance of lubricants with nanoparticles additives. Materials Today Proceedings, 25, 586–91.
- Singh, Y., D. Singh, A. Singla, A. Sharma, and N. K. Singh. 2020. Chemical modification of juliflora oil with trimethylolpropane (TMP) and effect of TiO2 nanoparticles concentration during tribological investigation. Fuel 280:118704. doi:10.1016/j.fuel.2020.118704.
- Soni, S., and M. Agarwal. 2014. Lubricants from renewable energy sources–a review. Green Chemistry Letters and Reviews 7 (4):359–382. doi:10.1080/17518253.2014.959565.
- Sulaiman, S. Z., A. L. Chuah, and A. Fakhru’l-Razi. 2007. Batch production of trimetylolpropane ester from palm oil as lubricant base stock. Journal of Applied Sciences 7 (15):2002–05. doi:10.3923/jas.2007.2002.2005.
- Tang, G., F. Su, X. Xu, and P. K. Chu. 2020. 2D black phosphorus dotted with silver nanoparticles: An excellent lubricant additive for tribological applications. Chemical Engineering Journal 392:123631. doi:10.1016/j.cej.2019.123631.
- Tesser, R., V. Russo, R. Turco, R. Vitiello, and M. Di Serio. 2020. Bio-lubricants synthesis from the epoxidized oil promoted by clays: Kinetic modelling. Chemical Engineering Science 214:115445. doi:10.1016/j.ces.2019.115445.
- Walters, C. J. 1986. Adaptive management of renewable resources. California: Macmillan Publishers Ltd.
- Wu, X., X. Zhang, S. Yang, H. Chen, and D. Wang. 2000. The study of epoxidized rapeseed oil used as a potential biodegradable lubricant. Journal of the American Oil Chemists’ Society 77 (5):561–63. doi:10.1007/s11746-000-0089-2.