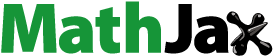
Abstract
The dramatic changes the world and the expectations of potential users have pushed researchers working in flexible foam pressure sensor (FFPS) fabrication to develop more affordable and high-performance materials. Among various materials, polymer foamed-based nanocomposites are a preferred choice due to their excellent mechanical properties, good chemical properties, and easy control. Moreover, the use of nanofillers such as carbon nanotubes (CNTs), carbon black (CB), and graphene in the polymer matrix has greatly improved the properties of the sensors. Therefore, this review focuses on the recent advances in FFPS by using different types of nanofillers in shape and structure. Accordingly, developments in the fabrication of FFPS, including dip coating, spray coating, sputtering, and in situ polymerization are also discussed. Special attention has been paid to identifying the underlying mechanism to maximize pressure sensing and improve the performance of FFPS. Suggestions for future developments in the area of sensing devices applied in health monitoring are also presented.
1. Introduction
Interest in pressure sensors, especially in FFPS, has gained enormous attention in recent years due to their high sensitivity, wide applicability, and high flexibility.[Citation1–5] Thanks to these outstanding properties of FFPS, they can be used as an electronic skin (e-skin) for biomimetic robots and prostheses, as well as to monitor bio-signals for humans.[Citation6] The operation concept of FPFS is based on the change of the electrical signal in response to the applied pressure.[Citation7–10] Their sensing mechanisms can be divided into several categories: capacitive,[Citation11–14] piezoelectric,[Citation15,Citation16] and piezoresistive.[Citation17–19] The capacitive FFPS measures the change in capacitance under pressure by inserting a flexible dielectric material between two flexible conductive plates.[Citation20,Citation21] The piezoelectric and piezoresistive FFPS are based on a change in resistance or voltage when pressure is applied to them.[Citation22]
Various processes are developed and applied to prepare FFPS, such as spray coating,[Citation23,Citation24] dip coating,[Citation19] sputtering,[Citation25] and in situ polymerization.[Citation26] These processes include the use of a conductive nanofiller combined with a foamed structure to create the sensor. The main differences between these methods are the dispersion quality of the nanofiller and the scalability, simplicity, and cost of the method. The nanofiller acts as a conductive layer that senses the change in electrical signal when pressure is applied. The foam structure serves as a supporting substrate for the nanofiller which compresses elastically under pressure. The flexible pressure sensors are classified according to their substrate structure: one-dimensional (1D), two-dimensional (2D), and three-dimensional (3D).[Citation27] 1D pressure sensor typically consists of a substrate made of a yarn of elastic rubber or elastic fibers to which conductive fillers are attached by coating or immersion. For example, Gong et al.[Citation28] prepared a pressure sensor by coating spandex yarn with MXene and polydopamine (PDA)/Ni2+. The result showed that the sensor has good pressure sensitivity and is suitable for health monitoring applications. 2D sensors are usually made from thin-film substrates coated with a conductive filler. Tao et al. prepared a paper-graphene pressure sensor, and it was found that the sensor can detect in a range of up to 20 kPa and good pressure sensitivity of 0-2 kPa−1 was also achieved. However, 1D and 2D sensors usually have a low-pressure detection limit and cannot sense very low-pressure signals.[Citation27] On the other hand, 3D foam substrates such as polydimethylsiloxane (PDMS), melamine foam (MF), polyimide foam (PF), and polyurethane (PU) have shown better sensing performance. This is due to the unique 3D microstructure and superior elasticity of the substrate.[Citation29] Moreover, the highly conductive foam with a porous structure is more easily deformable and sensitive to pressure.
Currently, nanofillers such as metal nanoparticles,[Citation30,Citation31] metal nanowires,[Citation18,Citation32] CNT,[Citation33,Citation34] graphene,[Citation35,Citation36] and carbon black(CB) [Citation37,Citation38] are used to create FFPS due to their excellent sensing properties,[Citation39–46] good mechanical properties,[Citation47–51] chemical stability,[Citation6,Citation52–56] and large surface area.[Citation57–61] The main objective of this review is to provide a comprehensive overview of flexible foam-based pressure sensors. The sensing mechanisms of the pressure sensors will be presented and a brief introduction to the foam structure used as a supporting substrate will also be included. Nanofillers commonly used as conductive layers will be discussed and the preparation of FFPS systems will be summarized. The evaluation of the pressure sensor and the factors affecting its performance will be described, and strategies to improve the performance of sensors are also presented. Furthermore, applications of FFPS, such as monitoring bio-signals on electronic skin and in humans will also be discussed.
2. Pressure sensing mechanisms
As mentioned earlier, the sensing mechanism of FFPS is divided into piezoresistive,[Citation4,Citation62] capacitive,[Citation12,Citation63,Citation64] and piezoelectric,[Citation15] types. The response of the sensing mechanism differs depending on the type of materials that make up the sensors’ structure. The operating principle of the piezoresistive sensor is based on the change in electrical resistance in response to the application of pressure (). When an external force presses on a piezoresistive sensor and causes it to change shape, the distance between the conductive fillers decreases. This creates more conductive paths, resulting in a reduction of the electrical resistance in the sensor (). The pressure sensitivity of the piezoresistive pressure (Spr) sensor is calculated by measuring the slope of the curve of the relative resistance changes versus the applied pressure:
(1)
(1)
where the relative resistance change is determined as (ΔR/Ro), ΔR = R − Ro, Ro is the initial resistance in Ω, R is the resistance while the sensor is under pressure, and ΔP is the varying pressure in Pa.
Figure 1. General sensing mechanism of foamed pressure sensor types: (a) piezoresistive, (b) capacitive, and (c) piezoelectric.

On the other hand, the operating principle of a capacitive pressure sensor is to detect the change in capacitance when pressure is applied to it.[Citation65] A capacitive pressure sensor is made up of a dielectric layer sandwiched between two parallel electrodes. The capacitance is calculated as follows:
(2)
(2)
where ε0 is the permittivity of vacuum, or is the relative permittivity, A is the area between the electrodes, and d is the distance between the electrodes. Under pressure, the thickness of the dielectric layer decreases, and the capacitance changes with the change in thickness ().[Citation66–68] The pressure sensitivity of such capacitive type of pressure sensors (Sc) can be calculated according to the following equation:
(3)
(3)
where co is the initial capacitance c is the capacitance when pressure is applied.
Piezoelectricity means that materials generate electrical voltage when pressure is applied to them. The FFPS piezoelectricity type takes advantage of this effect by measuring the change in voltage as a function of the applied pressure (). When pressure is applied, the piezoelectric sensor deforms, and polarization occurs. At the same time, negative and positive charges accumulate on their opposing surfaces and convert the mechanical pressure into an electrical signal.[Citation69] The pressure sensitivity of such systems can be determined by using the following equation:
(4)
(4)
where ΔI is the change of current.
3. Materials
3.1. Supporting substrate
Several types of foam structures have been successfully applied to create FFPS, such as PDMS,[Citation46,Citation62,Citation70–72] MF,[Citation2,Citation18,Citation19,Citation73,Citation74] PF,[Citation75] and PU.[Citation37,Citation76–78] PDMS is prepared from dimethyldichlorosilane which reacts with water. It can also be prepared by a reaction between hexamethylcyclotrisiloxane and octamethylcyclotetrasiloxane in the presence of acidic or basic catalysts.[Citation79] PDMS is a flexible polymer and offers many advantages, such as mechanical and chemical robustness, affordability, non-toxicity, and ease of shaping.[Citation80–82] However, two drawbacks limit its use in FFPS. First, the production of PDMS foam requires additional processes to insert pores into the structure of the material. This can be achieved by various methods, such as direct templating,[Citation83,Citation84] emulsion templating,[Citation85,Citation86] gas forming,[Citation87,Citation88] and 3D printing.[Citation89,Citation90] Second, in the production of polydimethylsiloxane, it’s difficult to control the structure because it’s difficult to manipulate the ratio of reactants. MF is a flexible polymer with low density and high porosity that can be prepared from a formaldehyde-melamine-sodium bisulfite copolymer.[Citation91] MF has good mechanical properties when pressure is applied to it; it compresses and returns to its original shape when pressure is released.[Citation92] However, MF has poor thermal properties and can decompose at low temperatures.[Citation93] PF has good thermal properties such as thermostability and flame retardancy. It also has excellent mechanical properties such as flexibility and tensile strength.[Citation94,Citation95] The powdered forming technique is the most widely used and typical process for the preparation of polyimide foam.[Citation96] This includes the following two steps: (1) the polymerization of benzophenone tetracarboxylic dianhydride and oxydianiline with a mixed solution of tetrahydrofuran and methanol; and (2) the imidization process and thermal forming. The chemical and physical properties of PF depend on many factors, such as foam structure, chemical composition, and density.[Citation97,Citation98] Nonetheless, compared to conventional substrates, the fabrication of PF-based sensors may require additional processing steps or challenges, which can increase the complexity and cost of manufacturing.[Citation95] PU is a highly flexible material with a high pore structure, low modulus of elasticity, and high resilience.[Citation39,Citation99–101] Therefore, PU could be compressed up to 85% without any plastic deformation.[Citation102] PU foam is manufactured easily by the reaction of polyol, isocyanate, and blowing agents in the presence of a catalyst.[Citation103] Foaming of PU can be initiated by the reaction of isocyanate and water (blowing agent), which leads to CO2 formation and turns the polymer into foam. One of the most interesting features of PU is that its properties can be controlled by controlling the ratio of reactants. The isocyanate is the so-called hard segment, while the polyol is the soft segment of polyurethane. Thus, by changing their ratio, the structures and properties of PU can be controlled.[Citation104] The degree of phase separation of the hard and soft segments and the content of the hard segment in turn affect the mechanical and physical properties.[Citation105,Citation106] However, like other types of foam, PU foam is typically porous and allows liquid or gas penetration. This permeability can affect the performance of nanofillers, compromising sensor reliability.[Citation107]
The physical and chemical properties of the above-discussed supporting substrates were compared (). It can be seen that PU is the best candidate for pressure sensors compared to other polymer foam structures because the polyurethane foam structure can be easily controlled by controlling the ratio of reactants during the synthesis. In addition, the ability to produce PU with a wide range of Young’s modulus and high compressive strength allows it to be used in a wide range of pressure levels and applications. In addition, PU can be made both hydrophilic and hydrophobic by selecting appropriate polyols and isocyanates.[Citation141] The PU is produced with a higher polyol content tends to be more hydrophilic. Isocyanates are more reactive; they are responsible for polymerization process and the stiffness of the PU. Hence, an excessive amount of isocyanate leads to a stiffer and more hydrophobic PU.[Citation141] Therefore, the properties of PU can be easily finetuned according to the properties (e.g. hydrophilicity) of the applied nanofiller.
Table 1. Physical and chemical properties of various substrates, such as polyurethane (PU), polydimethylsiloxane (PDMS), melamine foam (MF), and polyimide foam (PF), applied in flexible foam pressure sensor (FFPS) development.
3.2. Nanofillers
Various nanofiller types are used for FFPS preparation and these can be classified into four groups: zero-dimension (0D),[Citation31,Citation142,Citation143] one-dimension (1D),[Citation144,Citation145] two-dimension (2D),[Citation60,Citation146] and hybrid systems ().[Citation147–149] The 0D group includes the materials that have all dimensions at the nanoscale, such as silver nanoparticles (AgNP), and CB.[Citation150,Citation151] These have good electrical conductivity and are easy to synthesize.[Citation152–154] The 1D group represents those nanomaterials that are on the nanoscale in two dimensions, such as silver nanowires (AgNW) and CNTs. They have remarkably high aspect ratios[Citation155] and are most commonly used in FFPS, because of their excellent electrical properties and unique fibrous structure.[Citation6,Citation156] The 2D group includes structures that have two dimensions outside of the nanometric size range and the atomic-scale thickness. Graphene (GNP) and MXene are two great examples of 2D materials characterized by excellent mechanical properties, a large surface area, and remarkable electrical conductivity.[Citation157–160] MXene-based FFPS showed high sensitivity, wide pressure range detection, and good strain factor.
Among the aforementioned nanofillers, graphene is an attractive candidate for FFPS fabrication due to its excellent electrical conductivity, large surface area, and exceptional mechanical properties (). It has an electrical conductivity 99.94% higher than that of silver and Young’s modulus 75% higher than that of CNT. In addition, graphene has a 94% bigger surface area than CB. These properties enable graphene to reach the electrical conductivity percolation threshold of FFPS at lower loading.[Citation178] However, the large surface area[Citation179–181] and the strong Van der Waals force[Citation182,Citation183] lead to the agglomeration of graphene in the polymer matrix. Therefore, the improvement of FFPS performance by the addition of GNP is only possible if it is uniformly dispersed in the polymer matrix. One of the most effective strategies to overcome agglomeration and improve the dispersion of graphene is by combining different morphologies, shapes, and structures of nanofillers.[Citation184,Citation185] CNTs are the most suitable candidates to use with graphene as a hybrid nanofiller because the 1D CNTs can form a bridge with the 2D GNPs and thus, prevent the aggregation of GNPs, and improve the dispersion state of the nanofiller in the polymer matrix.[Citation186] The synergistic enhancement of hybrid graphene-CNTs can be described as a 3D hybrid structure. Such phenomena improve the dispersion and prevent graphene platelets from agglomerating. Simultaneously, a large surface area is created between graphene sheets and nanotubes which improves the electrical conductivity. In addition, the CNTs can act as extended tentacles for the 3D hybrid structure and interlock with the polymer matrix chain, leading to better interaction between nanotubes and graphene.[Citation179,Citation187]
Table 2. Nano-conductor properties of applied nanofillers.
4. Preparation of FFPS
Conductive foam can be made by combining an insulator foam and coating it with conductive filler via methods such as spray coating, in-situ polymerization, sputtering, and dip coating, as shown in () and (). Spray coating is a process in which materials are sprayed through a nozzle to form a fine aerosol ().[Citation196] This method has been used to fabricate FFPS by depositing conductive aerosol layer-by-layer on foamed PDMS.[Citation197] Spray coating involves applying a thin layer of a material to a substrate by spraying a suspension liquid or solution of the material onto the surface. This approach is often used to produce FFPS as it is a relatively simple and scalable process. The coating liquid is sprayed onto the supporting substrate such as PDMS using a spray gun or other spray device. The spray parameters, such as the spray rate and distance, are precisely controlled to ensure that an even coating is applied.[Citation197,Citation198] During in-situ nanocomposite polymerization, nanoparticles are dispersed in a monomeric solution and then, polymerization is carried out in the presence of the dispersed nanoparticles.[Citation199] Hu et al.[Citation200] describe the preparation of a polyurethane-based conductive sponge by coating it with silver nanoparticles after in-situ synthesis of poly(3,4-ethylenedioxythiophene) (PEDOT) on the backbone of a PU foam. Silver nitride is then dissolved in deionized water and the PU sponge is immersed in the silver nitride solution for 5 min, followed by drying at 60 °C for 6 h to obtain a PU/PEDOT-Ag pressure sensor (). Sputtering describes the ejection of atoms from a target by using high energy. Subsequently, the ejected atoms are deposited on the substrate material.[Citation201] This technique was used to fabricate FFPS by sputtering gold nanoparticles (AuNP) onto PU foam ().[Citation25] In dip coating, a conductive nanofiller is dispersed in a suitable solvent and then, the foamed structure is immersed into the solution. Finally, the solvent is removed in an oven to obtain a dispersed nanofiller on the foamed structure ().[Citation202] By comparing the methods, it can be seen that the dip coating is the most suitable for the fabrication of FFPS because it is the most cost-effective, the final sensor can be used directly, no special equipment or post-processing is required, and the substrates can be fully covered with the applied nanofiller (). In addition, a wide range of nanofiller types and concentrations can be used, which is a very efficient method for mass production.
Figure 3. FFPS preparation methods: (a) spray coating, (b) in-situ polymerization, (c) sputtering, and (d) dip coating.[Citation197][Citation200][Citation25][Citation202].
![Figure 3. FFPS preparation methods: (a) spray coating, (b) in-situ polymerization, (c) sputtering, and (d) dip coating.[Citation197][Citation200][Citation25][Citation202].](/cms/asset/0374ae42-ac4e-4b6c-9a1d-97cd45b0ea43/lmsc_a_2262558_f0003_c.jpg)
Table 3. Advantages and limitations of commonly used flexible foam pressure sensor (FFPS) preparation processes.
5. Evaluation of the performance of the pressure sensor
The piezoresistive pressure sensor detects the variation in electrical resistance of the sensor in response to deformations such as bending, compression, and torsion. To verify the performance of the sensor, various parameters such as resistance change (ΔR/Ro), strain, gauge factor (GF), and pressure sensitivity (S) are measured ().
Three mechanisms are behind the sensing of FFPS: connection nano-gap, micro-gap, and fracture surface of the conductive layers (). The nano- and microcrack mechanism occurred when a low and medium range was applied (),[Citation203] while in the case of high-pressure range, the contact area between the pores of the substrate and the fracture surface is a decisive factor in the measuring of S and GF ().
Figure 5. Sensing mechanisms of flexible foam pressure sensors (FFPS) is associated with structural changes at (a) low, (b) medium, and (c) high-pressure ranges.[Citation203]
![Figure 5. Sensing mechanisms of flexible foam pressure sensors (FFPS) is associated with structural changes at (a) low, (b) medium, and (c) high-pressure ranges.[Citation203]](/cms/asset/2713aa6e-1f8b-4e12-809d-a836bcfe4667/lmsc_a_2262558_f0005_c.jpg)
S and GF are important factors in testing the performance of FFPS. Various strategies have been employed to increase the sensitivity like using a substrate with a low elastic modulus. Such a system has a high deformation capacity, which results in an increased ability to detect low pressure/strain values. For example, PU has low elastic modulus, high pore structures, and high resilience which make it a good candidate for a high-performance FFPS substrate.[Citation204–206] Another method to improve S and GF is to create a rough surface in the conductive layers via the pre-strain method. Yang et al.[Citation76] introduced a microcrack on PU foam structure by applying the pre-strain technique (). Since they compressed the PU foam which is followed by a dip coating with graphene oxide (GO). After that, the compression load was released to create rough structures like micro-wrinkles and micro cracks on the conductive layers of the GO. The microcracks and micro-wrinkles act as microswitches that increase S and GF when pressure/strain is applied.
Figure 6. Flexible foam pressure sensors (FFPS): (a) manufacturing process, (b–c) morphology of FFPS without pre-strain, (d–e) morphology of pre-strained FFPS sample, (f) relation between change in relative current vs pressure, and (g) the relation between the change in relative current vs strain.[Citation76]
![Figure 6. Flexible foam pressure sensors (FFPS): (a) manufacturing process, (b–c) morphology of FFPS without pre-strain, (d–e) morphology of pre-strained FFPS sample, (f) relation between change in relative current vs pressure, and (g) the relation between the change in relative current vs strain.[Citation76]](/cms/asset/6db8daae-5fad-4834-a035-401208912843/lmsc_a_2262558_f0006_c.jpg)
A new technique was used by Ma et al. to improve S and GF by increasing the number of dip cycles (1, 3, 5) in the dip coating process (). The results showed that as the number of dip coating cycles increased, the GF increased due to decreased resistance (Ro), and the relative resistance change (ΔR/R0) also increased (). Thus, more dip-coating cycles caused a significant increase in electrical conductivity (). This phenomenon could be related to the formation of more conductive nanofiller networks. Unlikely, the pressure sensitivity of the sensor with 3 dip coating cycles is higher than that with 1 dip coating cycle (). However, the sensor with 5 dip coating cycles showed lower pressure sensitivity compared to the sensor with 3 dip coating cycles. This could be related to the fact that the sensor with 5 dip coating cycles has a higher Young’s modulus which results in less deformation of the sensor and consequently less change in resistance at the same compression pressure.[Citation203,Citation208]
Figure 7. The effect of the number of dip coating cycles on the (a) electrical conductivity, (b) sensitivity (S), and (c) gauge factor (GF).[Citation207]
![Figure 7. The effect of the number of dip coating cycles on the (a) electrical conductivity, (b) sensitivity (S), and (c) gauge factor (GF).[Citation207]](/cms/asset/e8f5f60e-7eb4-4ece-a3ac-ea8eeeca0935/lmsc_a_2262558_f0007_c.jpg)
Wang et al.[Citation209] used a different approach to improve the sensitivity of their CNT/PU pressure sensor by using different CNT loadings (2-10) wt.% (). They achieved the highest S value at 4 wt.% of CNT because the sensor with lower CNT content has high initial resistance, which leads to lower sensitivity. In turn, sensors with more than 4 wt.% had high stiffness, which led to less deformation of the sensor and consequently less change in resistance with pressure application.
Figure 8. The effect of carbon nanotube (CNT) loading wt.% on the properties of CNT/PU pressure sensor: (a) gauge factor (GF), (b) electrical conductivity, and (c) sensitivity (S).[Citation209]
![Figure 8. The effect of carbon nanotube (CNT) loading wt.% on the properties of CNT/PU pressure sensor: (a) gauge factor (GF), (b) electrical conductivity, and (c) sensitivity (S).[Citation209]](/cms/asset/9cb43917-909c-4360-a21d-2ee4de2eb519/lmsc_a_2262558_f0008_c.jpg)
Additionally, Nabeel et al.[Citation210] used another method to enhance S and GF by increasing the pore volume of the substrate (). A PU/CNT system was developed including PU with three different pore volumes. The results showed that a sample with a larger pore volume had a lower initial electrical resistance. This phenomenon could be related to the fact that the larger the pore volume, the smaller the entire PU scaffold. Consequently, more CNTs are interconnected, resulting in more conducting paths and a larger effective conducting area throughout the PU scaffold. In addition, the low initial resistance of PU leads to a big change in electrical resistance in response to pressure/strain, resulting in a high value of S and GF.[Citation210] The higher the total pore volume, the more wrinkles are formed and thus, a lower total PU and density are achieved (). Therefore, more interconnected CNTs lead to an increase in wrinkles and burrs. The burrs and wrinkles work as “microswitches” which can modulate the electrical resistance.[Citation204] In addition, the surface of the sample with a higher pore volume is richer and covered with more carbon nanotubes (). Therefore, it has lower electrical resistance and higher electrical conductivity than the other samples.
Figure 9. The relation between pore volume and (a) S, (b) GF, and (c) initial resistance. Morphology images of the prepared CNT/PU sensors, sample 1 (d-e), sample 2 (f-g), and sample 3 (h-i).[Citation210]
![Figure 9. The relation between pore volume and (a) S, (b) GF, and (c) initial resistance. Morphology images of the prepared CNT/PU sensors, sample 1 (d-e), sample 2 (f-g), and sample 3 (h-i).[Citation210]](/cms/asset/8dbebfa3-4583-410b-bbed-633050651c5c/lmsc_a_2262558_f0009_c.jpg)
The cyclic load test is applied to determine the durability and repeatability of FFPS which will also indicate the lifetime of the system.[Citation211] In the cyclic test, the electrical resistance rate is measured in response to repeated loading and unloading of the pressure which is performed to check the adhesion and bond strength between the nanofiller and substrate. Zhu et al.[Citation48] synthesized an AgNW/PU pressure sensor and investigated the stability of the sensor under 2300 cycles in the pressure range of 2 kPa (). The results showed that the amplitude of the peaks and the waveform were almost identical, indicating that the fabricated sensor has good stability and durability. Zhai et al.[Citation212] prepared a sensor and subjected it to various cyclic compressive loads (15, 30, 60, 80%) (). It was found that the sensor had excellent repeatability due to the good electrical properties of the applied CB and the excellent flexibility of the PU substrate. However, the relative change in resistance at 80% strain has a small drift in the first few cycles but soon becomes identical again.
Figure 10. Cyclic load vs. time (a); typical cyclic load vs. time at (b) 15%, (c) 30%, (d) 60%, and (e) 80% strain.[Citation212]
![Figure 10. Cyclic load vs. time (a); typical cyclic load vs. time at (b) 15%, (c) 30%, (d) 60%, and (e) 80% strain.[Citation212]](/cms/asset/fb51f1ee-49c3-46d0-a5c3-d05c0c3b4157/lmsc_a_2262558_f0010_c.jpg)
In the case of the ideal FFPS, the peak amplitude and the waveform of the resistance signal remained unchanged during the cyclic test.[Citation205] However, slight fluctuations in resistance rate peaks amplitude as a result of various reasons are possible. Due to the viscoelastic properties of the foam polymer matrix, which change its structure to withstand the applied pressure, it takes a long time to recover.[Citation39] In addition, the fracture of less stable conductive nanofiller layers during cyclic pressure could also cause such fluctuations.[Citation76] Moreover, the slight difference in the contact area of the conductive layers, even if the pressure is the same could also lead to fluctuations.
The desirable FFPS should have high sensitivity, linearity, and monotonicity. According to previous works,[Citation99,Citation213,Citation214] the cyclic load behavior was not monotonic manner i.e., at maximum pressure, an additional peak occurs which is called a peak shoulder. This is caused by the destruction, reconstruction, and reformation of the conductive network during the cyclic load.[Citation215] To achieve high sensor reliability, it is necessary to have a sharp main peak and eliminate the shoulder peak. The shoulder peak for 15-30% strain is much clearer than that for 5% strain. The low strain leads to weak destruction of the nanofiller network, so it can easily recover after the strain is removed.[Citation99] There are five models of peak shoulder phenomena presented in the literature ().[Citation215]
Figure 11. (a) Peak shoulder phenomena during cyclic loading and (b) visualization of bending as a cause of shoulder peak.[Citation215]
![Figure 11. (a) Peak shoulder phenomena during cyclic loading and (b) visualization of bending as a cause of shoulder peak.[Citation215]](/cms/asset/11cdaeb8-5f22-471e-90a4-ab91f24e05ff/lmsc_a_2262558_f0011_c.jpg)
Several factors can be behind the increase in the height and shape of the peak shoulder, including the viscoelastic properties of the polymer matrix, unstable conductive network, and the agglomeration of the nanoparticles.[Citation216] To achieve well-dispersed nanoparticles, the conductive layers need to connect easily. Thus, the sensor remains stable during the pressure application. On the other hand, agglomeration of the nanoparticles creates a high peak shoulder due to the separation of their layers. In addition, when the electrical conductivity exceeds the percolation threshold, the conductive layers are more stable, and then the chance is less to form a high peak shoulder.
6. Improving the performance of pressure sensor
One of the biggest challenges associated with FFPS is the unintentional detachment of the nanofiller from the substrate at the time of application pressure. This phenomenon could result from weak bonding between the nanofiller and the substrate like only van der Waals forces hold the nanofiller and the substrate together. Many solutions have been proposed to overcome such issues and improve the performance of the sensor.[Citation141,Citation208,Citation217] Nabeel et al.[Citation218] designed a pressure sensor by coating PU with CNT and then and the coated PU was impregnated with silicone rubber (SR) to fill the pores of PU and improve the stability of the final pressure sensor (). Lv et al.[Citation217] created the GO/polypyrrole@PU pressure sensor by immersing the PU into a hydrochloric acid solution to apply a positive charge, which exerted an electrostatic attraction on the negatively charged graphene oxide layer (). Then, the GO/PU was dipped into a pyrrole monomer (Py) ethanol solution to absorb the Py monomer through the GO layers. Finally, the Py monomer was polymerized by immersing it in FeCl3 (). In addition, Li et al.[Citation208] prepared an MXene-PU pressure sensor and improved the stability of the sensor by dispersing MXene in the presence of chitosan (CS) via alternately immersing positively charged chitosan and negatively charged MXene on the PU foam. This method improves the electrostatic interaction between MXene and PU and leads to an improvement in the stability of the pressure sensor (). Another strategy was used to improve the workability of the sensor by using different mixing ratios of isocyanate and polyol to prepare PU and then, dispersing CNT on the PU skeleton. The results showed that the samples with higher polyol ratios had lower electrical resistance and higher-pressure sensitivity. This is because PU with an excess of polyols tends to be more hydrophilic.[Citation141] Isocyanates are more reactive and responsible for the polymerization process and stiffness of the PU. Hence, an excessive amount of isocyanate leads to a stiffer and more hydrophobic PU. Therefore, the sample prepared with a higher polyol content is more hydrophilic and stable when functional CNT is dispersed on it ().[Citation141] Wang et al. manufactured polymer composite foam with superhydrophobic was synthesized by adsorption of an Ag precursor in tetrahydrofuran (THF) on a rubber sponge and subsequent reduction of Ag+ ions to Ag nanoparticles (). During the process of Ag+ reduction in a hydrazine solution, the rubber sponge swells and partially precipitates when treated with THF, a phenomenon known as nonsolvent-induced phase separation (NIPS). The formation of a porous structure on the surface of the sponge from NIPS leads to increased surface roughness, which in turn improves the superhydrophobic properties of the material. This approach improves sensor performance by fabricating superhydrophobic foamed pressure/strain sensors through non-solvent-induced phase separation. In addition, the interaction between individual AgNPs is improved by coating them with a precipitated polymer. The results show good reliability and durability of the foamed pressure/strain sensors. Multifunctional pressure or strain sensors have high water repellency and heating effects and can be used in harsh environments such as low temperatures and high humidity.[Citation207] Similarly, Qiang et al. present the successful synthesis of graphene oxide nanoribbons (GONR) and subsequent functionalization with silane molecules on a PU foam surface. The result of this process is the fabrication of porous composites with reduced graphene oxide nanoribbons (rGONR). These sensors exhibit remarkable properties such as superhydrophobicity, electrical conductivity, and mechanical flexibility. This study shows that the surface and physical properties of PU foam can be modified by grafting silane molecules onto GONR. The resulting changes range from insulating and hydrophobic properties to conductive and superhydrophobic properties. This approach aims to develop porous composites based on rGONRs with exceptional properties such as superhydrophobicity, electrical conductivity, and mechanical flexibility that can be used as strain sensors under harsh environmental conditions.[Citation220] Zhai et al. fabricated CB/PDMS FFPS with the three-dimensional conductive network by using CB to decorate the porous PDMS foams by ultrasonic treatment. The CB is both inside and outside the PDMS foam structure based on strong ultrasound, which improves the stability of electrical properties and response. The excellent linear response is due to the excellent conductive channels formed on the surfaces of the high compression modulus PDMS. In addition, the response of the sensor is well maintained in water due to its excellent hydrophobic properties (water contact angle of up to 149).[Citation46] Furthermore, Zhao et al. use a simple approach to manufacture high-performance FFPS. The approach involves the construction of a conductive/insulating/conductive sandwich-like porous foam structure (SPS) (). The SPS comprises three layers: The bottom and top layers are electrically conductive rGO-coated foam nanocomposites fabricated by dip coating and chemical reduction and a middle layer of electrically insulating PU foam. The SPS sensors exhibit extreme resistance switching performance, fast response and recovery time, high sensitivity, and outstanding mechanical properties. The impregnation of the conductive graphene network in the porous middle layer results in a highly efficient transition from an insulating to a conductive state. The sensor features high sensitivity, fast response, and good mechanical stability, offering a new concept for portable electronic applications.[Citation219]
Figure 12. Improving the performance of the sensor during preparation: (a) CNT/PU-SR, (b) GO/polypyrrole-PU, (c) MXene-CS@PU sensor by using LBL method, (d) CNT/PU sensor by using hydrophilic PU and functionalized CNT. (e) Schematic representation of the preparation of self-derived superhydrophobic and conductive foam nanocomposite. (f) Schematic demonstration of the manufacturing procedure of sandwich-like porous structure sensor based on graphene coated foams.[Citation207,Citation210,Citation217–219]
![Figure 12. Improving the performance of the sensor during preparation: (a) CNT/PU-SR, (b) GO/polypyrrole-PU, (c) MXene-CS@PU sensor by using LBL method, (d) CNT/PU sensor by using hydrophilic PU and functionalized CNT. (e) Schematic representation of the preparation of self-derived superhydrophobic and conductive foam nanocomposite. (f) Schematic demonstration of the manufacturing procedure of sandwich-like porous structure sensor based on graphene coated foams.[Citation207,Citation210,Citation217–219]](/cms/asset/740eb7af-e588-4548-93e8-525935d54db3/lmsc_a_2262558_f0012_c.jpg)
7. Morphology of flexible foam pressure sensors
The study of the morphology of FFPS is important as it provides important insights into the structure of the sensor and its effects on its performance. This is key to improving the durability, sensitivity, and overall functionality of sensors and paves the way for advanced applications in various fields. The surface of the pure PDMS foam was smooth (). Nonetheless, the wrinkly surface of the PDMS can also be seen (), demonstrating that Ag-CNTs-rGO nanocomposites are coated on the PDMS foam substrate. The Ag-CNTs-rGO nanocomposites overlapped each other and tightly connected with the adjacent CNTs (inset of ), which is valuable to improving the conductivity stability of the Ag-CNTs-rGO/PDMS foam.[Citation221]
Figure 13. The morphologies of Ag-CNTs-rGO/PDMS sponge. (a-b) SEM images of the PDMS foam and (e-f) Ag-CNTs-rGO/PDMS foam.[Citation221]
![Figure 13. The morphologies of Ag-CNTs-rGO/PDMS sponge. (a-b) SEM images of the PDMS foam and (e-f) Ag-CNTs-rGO/PDMS foam.[Citation221]](/cms/asset/df3cfe1e-aaf1-470d-a74e-e12e29a70ec8/lmsc_a_2262558_f0013_c.jpg)
Wang et al. investigated the effects of CNT loading on the electrical conductivity of CNTs/PU foam nanocomposites. The morphologies of PU foam and CNTs/PU foam nanocomposites with different CNT loadings were characterized (). The dispersion of CNTs with concentrations of (2, 4, 6, 8, and 10) wt.%on the PU foam was different (). Compared to pure PU foam, the conductive CNT fillers are all bound to the foam scaffold. At a CNT concentration of 2%, the CNTs are evenly arranged on the surface of the foam without tangling with each other. As the CNT concentration increases, the CNTs on the surface of the foam become entangled with each other, increasing the conductive network in space. However, when the CNT concentration is increased to 8 and 10 wt.% respectively, too many carbon nanotubes stack on top of each other, and gullies form.[Citation209] Such phenomena are related to the percolation threshold, when the CNT loading is very low, a continuous conductive network may not form PU. However, as the concentration of CNT content increases, a crucial point is eventually reached, the so-called percolation threshold, at which an electrically conductive network begins to develop. Consequently, the electrical conductivity increases significantly. With higher CNT proportions, the probability of the CNTs aggregating or clustering increases. The presence of these clusters can hinder the development of a highly effective network for conducting electricity, leading to a reduction in electrical conductivity.
Figure 14. SEM Images of dip-coated CNTs with different loading, (a) uncoated, (b) 2% MWCNTs; (c) 4% CNTs, (d) 6% CNTs, (e) 8% CNTs, (f) 10% CNTs.[Citation209]
![Figure 14. SEM Images of dip-coated CNTs with different loading, (a) uncoated, (b) 2% MWCNTs; (c) 4% CNTs, (d) 6% CNTs, (e) 8% CNTs, (f) 10% CNTs.[Citation209]](/cms/asset/06397491-b3a1-49e4-a822-6389e31aa41a/lmsc_a_2262558_f0014_c.jpg)
Wu et al. fabricated CB/PU pressure sensors and investigated the electrical properties under pressure as shown in . When the CB @ PU foam was pressurized, the bending of the PU foam caused stress in the CB conductive layer. Therefore, mechanical microcracks are easily formed in the CB conductive layers (). When the compression pressure is released, the microcracks close and form a crack joint. The 3D network foam CB @ PU before compression is intact, while it is deformed under 60% compression (). It can be seen that some CB @PU backbones touched each other, increasing the contact area between the conductive layers. By varying the contact area between the CB @PU backbones, the CB @PU foam was able to detect large compressive loads. As the pressure on CB @PU sponges increases, the micro-crack connection in the CB layer loosens first (). This causes interruptions in the local conduction paths to foam. When the pressure load steadily increases, the crack spacing and crack density increase accordingly, leading to a further decrease in the conductivity of CB layers ( middle). When the applied strain reaches a certain value, some CB @PU touch each other. This promotes the formation of more conductive paths ( right), leading to an increase in the electrical conductivity of the CB @PU foam. These two mechanisms act simultaneously and explain the pressure-sensitive behavior of the CB @PU foam, which endows CB @PU foam with the versatility to detect a wide range of deformations and high sensitivity.[Citation222]
Figure 15. Morphology of CB @PU foam. (a) SEM images of the microcrack joint on a CB @PU foam after compressive pretreatment, magnification: (c) 2000× and (b) 4000×. c) SEM images of an uncompressed and (d) compressed CB @PU foam, magnification: 100×. (e) Schematic evolution of conduction paths in a CB @PU foam during continuous compression deformation. The disruption of microcrack connections in the CB layer occurred at small deformations and disrupted local conductive pathways (Middle). CB @PU backbones touched each other at large deformations, leading to the formation of further conductive pathways in the CB layer (right).[Citation222]
![Figure 15. Morphology of CB @PU foam. (a) SEM images of the microcrack joint on a CB @PU foam after compressive pretreatment, magnification: (c) 2000× and (b) 4000×. c) SEM images of an uncompressed and (d) compressed CB @PU foam, magnification: 100×. (e) Schematic evolution of conduction paths in a CB @PU foam during continuous compression deformation. The disruption of microcrack connections in the CB layer occurred at small deformations and disrupted local conductive pathways (Middle). CB @PU backbones touched each other at large deformations, leading to the formation of further conductive pathways in the CB layer (right).[Citation222]](/cms/asset/4ee514af-3794-4f04-a2c2-a74f0add3133/lmsc_a_2262558_f0015_c.jpg)
8. Application of flexible foam pressure sensors (FFPS)
FFPS with a wide pressure range, high flexibility, and excellent pressure sensitivity meet the requirements for use in e-skin and wearable applications.[Citation223] Generally, an FFPS is attached to the human body or placed on wearable textiles to detect human activity. FFPS can be used both in the low-pressure range, such as breath and speech recognition, and in the high-pressure range, such as motion detection. Chen et al.[Citation17] prepared MXene/PU foam and used it for e-skin and wearable device applications. Due to the wide range of pressure detection, the sensor can recognize voices, facial movements, and hand and foot movements ().[Citation217]
Figure 16. Application of flexible foam pressure sensors (FFPS): (a) pressure range, (b) speech, (c) cheek, (d) finger, and (e) knee motion detection.[Citation17]
![Figure 16. Application of flexible foam pressure sensors (FFPS): (a) pressure range, (b) speech, (c) cheek, (d) finger, and (e) knee motion detection.[Citation17]](/cms/asset/6367db5b-7805-4772-8393-3a4068f9f883/lmsc_a_2262558_f0016_c.jpg)
Lue et al.[Citation213] developed rGO/PU and used it as a wearable pressure sensor to detect human activity. The sensor was placed on the neck, index finger joint, wrist, elbow, shoe sole, and face (). The results revealed that the current intensity changes proportionally to the pressure applied during neck bending, finger movement, wrist bending, arm bending, walking movement, and facial expression. When human motion compresses the sensor, the current of the sensor increases as it responds to the deformation of the sensor and returns to its initial value when the pressure is removed. These are encouraging results that offer the possibility of use foam-based sensors in intelligent robot applications. Dai et al.[Citation70] created a CNT/PDMS foam pressure sensor which was fitted in various locations on the human body to detect different practical body movements and physiological signs (). The sensor is attached to the wrist to detect the peaks of the heart pulses () and it can distinguish the peaks of T (tidal), P (percussive), and D (diastolic) in each heart pulse from the steady waveforms of the radial artery pulse at a heart rate of 68 beats per minute. A FFPS was also able to detect breathing by placing it on the subject’s chest to monitor respiration, which is an important physiological signal to prevent sleep apnea. Periodic breathing produces repeatable variations of ΔR/R0 (). In addition, the ΔR/R0 of the sensor shows different and repetitive patterns when the tester pronounces “Silicon,” “Hi,” and “Sensor” indicating a possible application in speech recognition devices ().
Figure 17. Monitoring human motion using rGO/PU foam sensor: a) finger pressing, b) finger movement, c) wrist bending, d) arm bending, e) nodding, f) knee bending, g) facial expression of cheek-bulging, and h) walking.[Citation213]
![Figure 17. Monitoring human motion using rGO/PU foam sensor: a) finger pressing, b) finger movement, c) wrist bending, d) arm bending, e) nodding, f) knee bending, g) facial expression of cheek-bulging, and h) walking.[Citation213]](/cms/asset/59efe76e-f85f-41a6-a504-d6856ac8013e/lmsc_a_2262558_f0017_c.jpg)
Figure 18. Applications of the CNT/PDMS sensor for detecting various physiological signals and monitoring human body movements: a) wrist pulse monitoring. The inset shows the pulse waveform of one cycle (right), where the P-wave, T-wave, and D-wave are visible. b) Monitoring respiration under normal conditions and after running. Inset: photo of the sensors attached to the chest. c) Detection of various acoustic stimuli when the wearer spoke “hi”, “sensor”, “silicon”.[Citation70]
![Figure 18. Applications of the CNT/PDMS sensor for detecting various physiological signals and monitoring human body movements: a) wrist pulse monitoring. The inset shows the pulse waveform of one cycle (right), where the P-wave, T-wave, and D-wave are visible. b) Monitoring respiration under normal conditions and after running. Inset: photo of the sensors attached to the chest. c) Detection of various acoustic stimuli when the wearer spoke “hi”, “sensor”, “silicon”.[Citation70]](/cms/asset/458979fe-7603-497d-982b-29264f7488fb/lmsc_a_2262558_f0018_c.jpg)
A simple and efficient ultrasound-assisted dip coating method for PU foam with carbonaceous nanofiller to produce conductive foam. The resulting conductive sponges exhibited various excellent properties, such as good compressibility, fast response time, and high sensitivity, which are the desired characteristics of piezoresistive sensing materials. The hybrid CNT-CB/PU foam with a ratio of CNT: CB = 1:20 exhibits optimal comprehensive performance in both low- and high-pressure areas. This hybrid CNT-CB/PU foam can be developed into a piezoresistive strain sensor that can detect various human motions over a wide range of strains, such as blowing, swallowing, deep breathing, bending fingers, elbows and knees as shown in .[Citation147] Beccatelli et al. investigated the functionalization of PU foam with the conductive polymer poly(3,4-ethylenedioxythiophene) polystyrene sulfonate (PEDOT: PSS). The result is a modified polymer foam whose internal pore network is uniformly coated with a layer of PEDOT: PSS, which acts as a mechanical transducer. The main objective of this study is to investigate the potential use of the research results for the development of flexible pressure sensors. In particular, these sensors are based on the use of PEDOT: PSS-modified PU foam. These sensors can be used in many applications, including biomedical, sports, and robotics. The fully realized prototype polymer insole with integrated monitoring provides medical professionals with important data, such as instantaneous body weight distribution and a comprehensive representation of walking dynamics. The use of PEDOT: PSS for PU foam functionalization is a suitable approach for pressure sensor fabrication. This technology offers several advantages, including lower sensitivity to pressure variations, cost efficiency, long-term stability, and ease of fabrication. The proposed micromechanical model can be used to predict the conversion capacities of the device and adjust the parameters to achieve devices with sensitivity in the approximate range of 1 to 1000 Pa−1.[Citation206]
Figure 19. Response of resistance signals of the CNT-CB/PU pressure sensor for various body motions, including (a) blowing, (b) swallowing, (c) deep breath, (d) finger bending, (e) elbow bending, and (f) knee bending.[Citation147]
![Figure 19. Response of resistance signals of the CNT-CB/PU pressure sensor for various body motions, including (a) blowing, (b) swallowing, (c) deep breath, (d) finger bending, (e) elbow bending, and (f) knee bending.[Citation147]](/cms/asset/3b5e930b-52e1-414e-a586-79d70a83db3a/lmsc_a_2262558_f0019_c.jpg)
Chen et al. have fabricated dual-mode strain sensors based on a porous GNP foam nanocomposite for strain measurement. The dual-mode waterproof sensors can detect large, small, and even subtle movements and temperature changes both in dry conditions and underwater (). Furthermore, the dual-mode sensor can be used in a variety of environments, such as detecting pulse waveforms beyond common locations such as the carotid artery, wrist artery, and ankle, to much more difficult locations further away from the heart (). The sensor detects pulse waveforms at the eyebrow bone (green), fingertip (purple), and toe (orange) (). In addition, the sensor shows a rapid response to multiple voluntary contractions and relaxation of the biceps and flexor carpi radialis and correlates the response with the surface electromyogram (sEMG) in terms of contraction times and ranges (). Contraction of the biceps muscle produces a substantial sensor response, totaling 300 units, with variations between 250 and 350 units. The observed local peaks within the sensor response envelope are consistent with surface electromyography (sEMG) data (). In addition, the sensor attached to the forearm of the human subject can also provide a fast response and is very sensitive to the simulated resting tremor in Parkinson’s disease ().[Citation224] On the other hand, Sencadas et al. fabricated FFPS and used it in robotic systems by synthesizing the foam structure of poly (glycerol secabate) (PGS) and then incorporating it with CNT. Three sensors were attached to the fingers of the gripper (). The status of each sensor was visually displayed using a simulated light-emitting diode (LED) and the corresponding change in resistance was plotted, as shown in . The resistance of the individual sensors decreased as the gripper grasped the objects because the sensors were mechanically pressed together and the resistance of the individual sensors. The gripper can effectively grasp various objects with different shapes and textures and also can detect any form of contact between the tip of its flexible fingers and the objects to be grasped. The above results demonstrate the potential use of these sensors in soft adaptive grippers and other soft robot applications that require elastic touch sensors.[Citation225]
Figure 20. Realistic and schematic representation of (a) prototype orthopedic insole with embedded pressure sensors mapping the spatial distribution of body weight, (b) representation of pressure distribution when a person stands still (c) current-time diagram of each sensor monitoring the dynamics of footstep during walking.[Citation206]
![Figure 20. Realistic and schematic representation of (a) prototype orthopedic insole with embedded pressure sensors mapping the spatial distribution of body weight, (b) representation of pressure distribution when a person stands still (c) current-time diagram of each sensor monitoring the dynamics of footstep during walking.[Citation206]](/cms/asset/585a405e-fc19-4184-8843-87b19503b501/lmsc_a_2262558_f0020_c.jpg)
Figure 21. (a, b) the Utilization of dual-mode waterproof sensors for the detection of small, and large strain ranges. (c) Schematic diagram of the network of human arteries showing the subtlest movements at the eyebrow bone, fingertip, and toe. (d) Identification and measurement of the pulse waveform on the eyebrow bone (green), fingertip (purple), and toe (orange). (e) the comparison between the sensory response (blue) and the muscle action (red) identified by the surface electromyogram (sEMG) in response to different voluntary relaxation/contraction cycles of the biceps muscle group, with a magnified view of the sensory response during muscle relaxation and contraction. (f) The comparison of the sensor response (blue) and the sEMG signals (red) processed with an integrated data processing system within a period of 1 s. (g) Attaching the sensor to the biceps measures the signal of muscle tension. (h) The response of the sensor to the simulated static parkinson’s tremor at a frequency of 5.5 Hz with an illustration showing the placement of the sensor.[Citation224]
![Figure 21. (a, b) the Utilization of dual-mode waterproof sensors for the detection of small, and large strain ranges. (c) Schematic diagram of the network of human arteries showing the subtlest movements at the eyebrow bone, fingertip, and toe. (d) Identification and measurement of the pulse waveform on the eyebrow bone (green), fingertip (purple), and toe (orange). (e) the comparison between the sensory response (blue) and the muscle action (red) identified by the surface electromyogram (sEMG) in response to different voluntary relaxation/contraction cycles of the biceps muscle group, with a magnified view of the sensory response during muscle relaxation and contraction. (f) The comparison of the sensor response (blue) and the sEMG signals (red) processed with an integrated data processing system within a period of 1 s. (g) Attaching the sensor to the biceps measures the signal of muscle tension. (h) The response of the sensor to the simulated static parkinson’s tremor at a frequency of 5.5 Hz with an illustration showing the placement of the sensor.[Citation224]](/cms/asset/9e6771e9-82a2-40e7-9744-db01a84d75d2/lmsc_a_2262558_f0021_c.jpg)
Figure 22. Application of the foamed pressure sensor in soft robotic systems: (a) soft gripper with the sensors attached, (b) change in electrical resistance before, (c) during, and (d) after releasing a tennis ball.[Citation225]
![Figure 22. Application of the foamed pressure sensor in soft robotic systems: (a) soft gripper with the sensors attached, (b) change in electrical resistance before, (c) during, and (d) after releasing a tennis ball.[Citation225]](/cms/asset/166ba4dc-65d4-44d6-b56d-92e471318f56/lmsc_a_2262558_f0022_c.jpg)
There are many applications of FFPS to highlight their versatility in various fields and to provide a comprehensive overview, is presented below, detailing the pressure sensor types, their properties, and corresponding applications.
Table 4. Summary of the application and performance of FFPS.
9. Conclusion
A detailed comparison of flexible foam pressure sensors based on a variety of compounds, manufacturing processes, performance, and applications is presented. To design FFPS with high performance, PU could be a desirable substrate due to its ability to produce pressure sensors with variable levels of stiffness and compression strength. The hydrophilicity of PU can be controlled to match the selected polarity of the nanofiller. Moreover, it can also be successfully applied with hybrid nanofillers to create FFPS systems. By assessing the various preparation methods, dip coating is the easiest and most effective way to manufacture FFPS directly without the necessity of advanced equipment. The performance of FFPS can be enhanced in terms of sensitivity and gauge factor by controlling the number of dip cycles of the substrate in nanofiller suspension, nanofiller loading, and pore size of the substrate. In addition, the durability of FFPS can also be improved by adding silicone rubber to fill the substrate pores as well as improving the bonding between the nanofiller and substrate. FFPS applicability has a huge potential in bio-signal monitoring and human activity detection. Furthermore, the use of FFPS in smart robots and human-machine interfaces is directions for future perspectives. All in all, a comprehensive review of recent advances in flexible foam pressure sensors is prepared.
Disclosure statement
The authors report there are no competing interests to declare.
Additional information
Funding
References
- Su, Y.; Ma, K.; Mao, X.; Liu, M.; Zhang, X. Highly Compressible and Sensitive Flexible Piezoresistive Pressure Sensor Based on MWCNTs/Ti3C2Tx MXene @ Melamine Foam for Human Gesture Monitoring and Recognition. Nanomaterials (Basel) 2022, 12, 2225. DOI: 10.3390/nano12132225.
- Li, Z.-X.; Gao, X.-Y.; Huang, P.; Li, Y.-Q.; Fu, S.-Y. A Flexible Carbonized Melamine Foam/Silicone/Epoxy Composite Pressure Sensor with Temperature and Voltage-Adjusted Piezoresistivity for Ultrawide Pressure Detection. J. Mater. Chem. A 2022, 10, 9114–9120. DOI: 10.1039/D1TA10965K.
- Zhu, W.-B.; Luo, H.-S.; Tang, Z.-H.; Zhang, H.; Fan, T.; Wang, Y.-Y.; Huang, P.; Li, Y.-Q.; Fu, S.-Y. Ti3C2T x MXene/Bamboo Fiber/PDMS Pressure Sensor with Simultaneous Ultrawide Linear Sensing Range, Superb Environmental Stability, and Excellent Biocompatibility. ACS Sustainable Chem. Eng. 2022, 10, 3546–3556. DOI: 10.1021/acssuschemeng.1c07994.
- Liu, M.-Y.; Zhao, X.-F.; Hang, C.-Z.; Zhu, L.-Y.; Wu, X.-Y.; Wen, X.-H.; Wang, J.-C.; Lu, H.-L. A Stretchable Pressure Sensor with Interlinked Interfaces Prepared by a Template-Free Process. Compos Part A Appl. Sci. Manuf. 2022, 162, 107144. DOI: 10.1016/j.compositesa.2022.107144.
- Kurup, L. A.; Arthur, J. N.; Yambem, S. D. Highly Sensitive Capacitive Low-Pressure Graphene Porous Foam Sensors. ACS Appl. Electron. Mater. 2022, 4, 3962–3972. DOI: 10.1021/acsaelm.2c00616.
- He, J.; Zhang, Y.; Zhou, R.; Meng, L.; Chen, T.; Mai, W.; Pan, C. Recent Advances of Wearable and Flexible Piezoresistivity Pressure Sensor Devices and Its Future Prospects. J. Materiomics 2020, 6, 86–101. DOI: 10.1016/j.jmat.2020.01.009.
- Yu, Z.; Cai, G.; Liu, X.; Tang, D. Pressure-Based Biosensor Integrated with a Flexible Pressure Sensor and an Electrochromic Device for Visual Detection. Anal. Chem. 2021, 93, 2916–2925. DOI: 10.1021/acs.analchem.0c04501.
- Huang, C.-B.; Witomska, S.; Aliprandi, A.; Stoeckel, M.-A.; Bonini, M.; Ciesielski, A.; Samorì, P. Molecule-Graphene Hybrid Materials with Tunable Mechanoresponse: Highly Sensitive Pressure Sensors for Health Monitoring. Adv. Mater. 2019, 31, e1804600. DOI: 10.1002/adma.201804600.
- Garcia, C.; Trendafilova, I.; Guzman de Villoria, R.; Sanchez del Rio, J. Self-Powered Pressure Sensor Based on the Triboelectric Effect and Its Analysis Using Dynamic Mechanical Analysis. Nano Energy 2018, 50, 401–409. DOI: 10.1016/j.nanoen.2018.05.046.
- Kou, H.; Zhang, L.; Tan, Q.; Liu, G.; Lv, W.; Lu, F.; Dong, H.; Xiong, J. Wireless Flexible Pressure Sensor Based on Micro-Patterned Graphene/PDMS Composite. Sens. Actuators A Phys. 2018, 277, 150–156. DOI: 10.1016/j.sna.2018.05.015.
- Ha, K.-H.; Huh, H.; Li, Z.; Lu, N. Soft Capacitive Pressure Sensors: Trends, Challenges, and Perspectives. ACS Nano. 2022, 16, 3442–3448. DOI: 10.1021/acsnano.2c00308.
- Ma, Z.; Zhang, K.; Yang, S.; Zhang, Y.; Chen, X.; Fu, Q.; Deng, H. High-Performance Capacitive Pressure Sensors Fabricated by Introducing Dielectric Filler and Conductive Filler into a Porous Dielectric Layer through a Biomimic Strategy. Compos. Sci. Technol. 2022, 227, 109595. DOI: 10.1016/j.compscitech.2022.109595.
- Kurup, L. A.; Cole, C. M.; Arthur, J. N.; Yambem, S. D. Graphene Porous Foams for Capacitive Pressure Sensing. ACS Appl. Nano Mater. 2022, 5, 2973–2983. DOI: 10.1021/acsanm.2c00247.
- Yu, H.; Guo, C.; Ye, X.; Pan, Y.; Tu, J.; Wu, Z.; Chen, Z.; Liu, X.; Huang, J.; Ren, Q.; Li, Y. Wide-Range Flexible Capacitive Pressure Sensors Based on Dielectrics with Various Porosity. Micromachines (Basel) 2022, 13, 1588. DOI: 10.3390/mi13101588.
- Han, C.; Zhang, H.; Chen, Q.; Li, T.; Kong, L.; Zhao, H.; He, L. A Directional Piezoelectric Sensor Based on Anisotropic PVDF/MXene Hybrid Foam Enabled by Unidirectional Freezing. Chem. Eng. J. 2022, 450, 138280. DOI: 10.1016/j.cej.2022.138280.
- Zhang, X.; Li, J.; Lin, J.; Li, W.; Chu, W.; Wang, X. Facile Fabrication of Carbon Ink-Decorated Melamine Foams for Efficient Piezoelectric Pressure Sensors. J. Mater. Sci. Mater. Electron. 2022, 33, 13731–13742. DOI: 10.1007/s10854-022-08306-6.
- Chen, Q.; Gao, Q.; Wang, X.; Schubert, D. W.; Liu, X. Flexible, Conductive, and Anisotropic Thermoplastic Polyurethane/Polydopamine/MXene Foam for Piezoresistive Sensors and Motion Monitoring. Compos. Part A Appl. Sci. Manuf. 2022, 155, 106838. DOI: 10.1016/j.compositesa.2022.106838.
- Cai, B.; Wang, L.; Yu, F.; Jia, j.; Li, J.; Li, X.; Yang, X.; Jiang, Y.; Lü, W. Compressible Piezoresistive Pressure Sensor Based on Ag Nanowires Wrapped Conductive Carbonized Melamine Foam. Appl. Phys. A 2022, 128, 6. DOI: 10.1007/s00339-021-05143-y.
- Bhatt, B. B.; Kumar, L.; Kushwaha, A.; Gupta, D. An Ultra-Compressible Piezoresistive Strain and Pressure Sensor Based on RGO-CNT-Melamine Foam Composite for Biomedical Sensing. Sens. Actuators A Phys. 2021, 331, 112875. DOI: 10.1016/j.sna.2021.112875.
- Mu, C.; Li, J.; Song, Y.; Huang, W.; Ran, A.; Deng, K.; Huang, J.; Xie, W.; Sun, R.; Zhang, H. Enhanced Piezocapacitive Effect in CaCu3Ti4O12–Polydimethylsiloxane Composited Sponge for Ultrasensitive Flexible Capacitive Sensor. ACS Appl. Nano Mater. 2018, 1, 274–283. DOI: 10.1021/acsanm.7b00144.
- Kang, M.; Kim, J.; Jang, B.; Chae, Y.; Kim, J.-H.; Ahn, J.-H. Graphene-Based Three-Dimensional Capacitive Touch Sensor for Wearable Electronics. ACS Nano. 2017, 11, 7950–7957. DOI: 10.1021/acsnano.7b02474.
- Shehata, N.; Nair, R.; Boualayan, R.; Kandas, I.; Masrani, A.; Elnabawy, E.; Omran, N.; Gamal, M.; Hassanin, A. H. Stretchable Nanofibers of Polyvinylidenefluoride (PVDF)/Thermoplastic Polyurethane (TPU) Nanocomposite to Support Piezoelectric Response via Mechanical Elasticity. Sci. Rep. 2022, 12, 8335. DOI: 10.1038/s41598-022-11465-5.
- Tran, M. T.; Tung, T. T.; Sachan, A.; Losic, D.; Castro, M.; Feller, J. F. 3D Sprayed Polyurethane Functionalized Graphene/Carbon Nanotubes Hybrid Architectures to Enhance the Piezo-Resistive Response of Quantum Resistive Pressure Sensors. Carbon N Y 2020, 168, 564–579. DOI: 10.1016/j.carbon.2020.05.086.
- Offenzeller, C.; Knoll, M.; Jakoby, B.; Hilber, W. Embedded, Fully Spray-Coated Pressure Sensor Using a Capacitive Transducing Mechanism. Polymers (Basel) 2018, 10, 852. DOI: 10.3390/polym10080852.
- Wu, Y.; Liu, H.; Chen, S.; Dong, X.; Wang, P.; Liu, S.; Lin, Y.; Wei, Y.; Liu, L. Channel Crack-Designed Gold@PU Sponge for Highly Elastic Piezoresistive Sensor with Excellent Detectability. ACS Appl. Mater. Interfaces. 2017, 9, 20098–20105. DOI: 10.1021/acsami.7b04605.
- Du, C.; Zhang, Y.; Zhang, D.; Zhang, B.; Zhao, W. An in Situ Polymerized Polypyrrole/Halloysite Nanotube–Silver Nanoflower Based Flexible Wearable Pressure Sensor with a Large Measurement Range and High Sensitivity. J. Mater. Chem. C 2021, 9, 13172–13181. DOI: 10.1039/D1TC03135J.
- Wang, Y.; Yue, Y.; Cheng, F.; Cheng, Y.; Ge, B.; Liu, N.; Gao, Y. Ti3C2Tx MXene-Based Flexible Piezoresistive Physical Sensors. ACS Nano. 2022, 16, 1734–1758. DOI: 10.1021/acsnano.1c09925.
- Gong, M.; Yue, L.; Kong, J.; Lin, X.; Zhang, L.; Wang, J.; Wang, D. Knittable and Sewable Spandex Yarn with Nacre-Mimetic Composite Coating for Wearable Health Monitoring and Thermo- and Antibacterial Therapies. ACS Appl. Mater. Interfaces. 2021, 13, 9053–9063. DOI: 10.1021/acsami.1c00864.
- Kang, F.; Zhang, W.; Liu, M.; Liu, F.; Jia, Z.; Jia, D. Highly Flexible and Sensitive Ti3C2 MXene@Polyurethane Composites for Piezoresistive Pressure Sensor. J. Mater. Sci. 2022, 57, 12894–12902. DOI: 10.1007/s10853-022-07387-2.
- Yan, Q.; Xie, W.; Zhou, M.; Fu, H. An Ultrasensitive and Highly Compressive Piezoresistive Sensor Based on a Biopolyol-Reinforced Polyurethane Sponge Coated with Silver Nanoparticles and Carbon Nanotubes/Cellulose Nanocrystals. J. Mater. Chem. C 2020, 8, 16603–16614. DOI: 10.1039/D0TC04141F.
- Paghi, A.; Corsi, M.; Corso, S.; Mariani, S.; Barillaro, G. In Situ Controlled and Conformal Coating of Polydimethylsiloxane Foams with Silver Nanoparticle Networks with Tunable Piezo-Resistive Properties. Nanoscale Horiz. 2022, 7, 425–436. DOI: 10.1039/D1NH00648G.
- Hu, T.; Xuan, S.; Ding, L.; Gong, X. Stretchable and Magneto-Sensitive Strain Sensor Based on Silver Nanowire-Polyurethane Sponge Enhanced Magnetorheological Elastomer. Mater. Des. 2018, 156, 528–537. DOI: 10.1016/j.matdes.2018.07.024.
- Fei, Y.; Jiang, R.; Fang, W.; Liu, T.; Saeb, M. R.; Hejna, A.; Ehsani, M.; Barczewski, M.; Sajadi, S. M.; Chen, F.; Kuang, T. Highly Sensitive Large Strain Cellulose/Multiwalled Carbon Nanotubes (MWCNTs)/Thermoplastic Polyurethane (TPU) Nanocomposite Foams: From Design to Performance Evaluation. J. Supercrit. Fluids 2022, 188, 105653. DOI: 10.1016/j.supflu.2022.105653.
- Zhang, T.; Zhang, W.; Li, Y.; Hu, X.; Yuan, H.; Jiang, T. High-Efficient Flexible Pressure Sensor Based on Nanofibers and Carbon Nanotubes for Artificial Electronic Skin and Human Motion Monitoring. J. Porous Mater. 2023. DOI: 10.1007/s10934-023-01465-9.
- Sengupta, D.; Kamat, A. M.; Smit, Q.; Jayawardhana, B.; Kottapalli, A. G. P. Piezoresistive 3D Graphene–PDMS Spongy Pressure Sensors for IoT Enabled Wearables and Smart Products. Flex. Print. Electron. 2022, 7, 015004. DOI: 10.1088/2058-8585/ac4d0e.
- Luo, R.; Cui, Y.; Li, H.; Wu, Y.; Du, b.; Zhou, S. A Sponge-Like High Sensitivity Wearable Piezoresistive Pressure Sensor Based on Fragmented Graphene Aerogel/Polydimethylsiloxane. SSRN J. 2022, 26, 2779–2787. DOI: 10.2139/ssrn.4138054.
- Zhu, H.; Dai, S.; Cao, J.; Bai, H.; Zhong, Y.; Zhang, Z.; Cheng, G.; Yuan, N.; Ding, J. A High-Performance Textile Pressure Sensor Based on Carbon Black/Carbon Nanotube-Polyurethane Coated Fabrics with Porous Structure for Monitoring Human Motion. Mater. Today Commun. 2022, 33, 104541. DOI: 10.1016/j.mtcomm.2022.104541.
- Nabeel, M.; Kuzsella, L.; Viskolcz, B.; Kollar, M.; Fiser, B.; Vanyorek, L. Synergistic Effect of Carbon Nanotubes and Carbon Black as Nanofillers of Silicone Rubber Pressure Sensors. Arabian J. Chem. 2023, 16, 104594. DOI: 10.1016/j.arabjc.2023.104594.
- Huang, W.; Dai, K.; Zhai, Y.; Liu, H.; Zhan, P.; Gao, J.; Zheng, G.; Liu, C.; Shen, C. Flexible and Lightweight Pressure Sensor Based on Carbon Nanotube/Thermoplastic Polyurethane-Aligned Conductive Foam with Superior Compressibility and Stability. ACS Appl. Mater. Interfaces. 2017, 9, 42266–42277. DOI: 10.1021/acsami.7b16975.
- Lee, D.; Kim, J.; Kim, H.; Heo, H.; Park, K.; Lee, Y. High-Performance Transparent Pressure Sensors Based on Sea-Urchin Shaped Metal Nanoparticles and Polyurethane Microdome Arrays for Real-Time Monitoring. Nanoscale 2018, 10, 18812–18820. DOI: 10.1039/C8NR05843A.
- Cheng, Y.; Wang, R.; Zhai, H.; Sun, J. Stretchable Electronic Skin Based on Silver Nanowire Composite Fiber Electrodes for Sensing Pressure, Proximity, and Multidirectional Strain. Nanoscale 2017, 9, 3834–3842. DOI: 10.1039/C7NR00121E.
- Chen, X.; Liu, H.; Zheng, Y.; Zhai, Y.; Liu, X.; Liu, C.; Mi, L.; Guo, Z.; Shen, C. Highly Compressible and Robust Polyimide/Carbon Nanotube Composite Aerogel for High-Performance Wearable Pressure Sensor. ACS Appl. Mater. Interfaces. 2019, 11, 42594–42606. DOI: 10.1021/acsami.9b14688.
- He, Y.; Zhou, M.; Mahmoud, M. H. H.; Lu, X.; He, G.; Zhang, L.; Huang, M.; Elnaggar, A. Y.; Lei, Q.; Liu, H.; et al. Multifunctional Wearable Strain/Pressure Sensor Based on Conductive Carbon Nanotubes/Silk Nonwoven Fabric with High Durability and Low Detection Limit. Adv. Compos. Hybrid Mater. 2022, 5, 1939–1950. DOI: 10.1007/s42114-022-00525-z.
- Song, Z.; Li, W.; Bao, Y.; Wang, W.; Liu, Z.; Han, F.; Han, D.; Niu, L. Bioinspired Microstructured Pressure Sensor Based on a Janus Graphene Film for Monitoring Vital Signs and Cardiovascular Assessment. Adv. Elect. Materials 2018, 4, 1800252. DOI: 10.1002/aelm.201800252.
- Lu, Y.; Tian, M.; Sun, X.; Pan, N.; Chen, F.; Zhu, S.; Zhang, X.; Chen, S. Highly Sensitive Wearable 3D Piezoresistive Pressure Sensors Based on Graphene Coated Isotropic Non-Woven Substrate. Compos Part A Appl. Sci. Manuf. 2019, 117, 202–210. DOI: 10.1016/j.compositesa.2018.11.023.
- Zhai, W.; Xia, Q.; Zhou, K.; Yue, X.; Ren, M.; Zheng, G.; Dai, K.; Liu, C.; Shen, C. Multifunctional Flexible Carbon Black/Polydimethylsiloxane Piezoresistive Sensor with Ultrahigh Linear Range, Excellent Durability and Oil/Water Separation Capability. Chem. Eng. J. 2019, 372, 373–382. DOI: 10.1016/j.cej.2019.04.142.
- Shen, X.; Zheng, L.; Tang, R.; Nie, K.; Wang, Z.; Jin, C.; Sun, Q. Double-Network Hierarchical-Porous Piezoresistive Nanocomposite Hydrogel Sensors Based on Compressive Cellulosic Hydrogels Deposited with Silver Nanoparticles. ACS Sustainable Chem. Eng. 2020, 8, 7480–7488. DOI: 10.1021/acssuschemeng.0c02035.
- Zhu, G. J.; Ren, P. G.; Wang, J.; Duan, Q.; Ren, F.; Xia, W. M.; Yan, D. X. A Highly Sensitive and Broad-Range Pressure Sensor Based on Polyurethane Mesodome Arrays Embedded with Silver Nanowires. ACS Appl. Mater. Interfaces. 2020, 12, 19988–19999. DOI: 10.1021/acsami.0c03697.
- Luo, Q.; Zheng, H.; Hu, Y.; Zhuo, H.; Chen, Z.; Peng, X.; Zhong, L. Carbon Nanotube/Chitosan-Based Elastic Carbon Aerogel for Pressure Sensing. Ind. Eng. Chem. Res. 2019, 58, 17768–17775. DOI: 10.1021/acs.iecr.9b02847.
- Zhao, L.; Jiang, B.; Huang, Y. Self-Healable Polysiloxane/Graphene Nanocomposite and Its Application in Pressure Sensor. J. Mater. Sci. 2019, 54, 5472–5483. DOI: 10.1007/s10853-018-03233-6.
- Huang, J.; Zhao, M.; Cai, Y.; Zimniewska, M.; Li, D.; Wei, Q. A Dual‐Mode Wearable Sensor Based on Bacterial Cellulose Reinforced Hydrogels for Highly Sensitive Strain/Pressure Sensing. Adv. Elect. Materials 2020, 6, 1900934. DOI: 10.1002/aelm.201900934.
- Tan, P.; Li, H.; Wang, J.; Gopinath, S. C. B. Silver Nanoparticle in Biosensor and Bioimaging: Clinical Perspectives. Biotechnol. Appl. Biochem. 2020, 68, bab.2045. DOI: 10.1002/bab.2045.
- Fu, D.; Wang, R.; Wang, Y.; Sun, Q.; Cheng, C.; Guo, X.; Yang, R. An Easily Processable Silver Nanowires-Dual-Cellulose Conductive Paper for Versatile Flexible Pressure Sensors. Carbohydr. Polym. 2022, 283, 119135. DOI: 10.1016/j.carbpol.2022.119135.
- Venkataraman, A.; Amadi, E. V.; Chen, Y.; Papadopoulos, C. Carbon Nanotube Assembly and Integration for Applications. Nanoscale Res. Lett. 2019, 14, 220. DOI: 10.1186/s11671-019-3046-3.
- He, J.; Xiao, P.; Lu, W.; Shi, J.; Zhang, L.; Liang, Y.; Pan, C.; Kuo, S.-W.; Chen, T. A Universal High Accuracy Wearable Pulse Monitoring System via High Sensitivity and Large Linearity Graphene Pressure Sensor. Nano Energy 2019, 59, 422–433. DOI: 10.1016/j.nanoen.2019.02.036.
- Gupta, S.; Tai, N.-H. Carbon Materials and Their Composites for Electromagnetic Interference Shielding Effectiveness in X-Band. Carbon N Y 2019, 152, 159–187. DOI: 10.1016/j.carbon.2019.06.002.
- Han, X.; Lv, Z.; Ran, F.; Dai, L.; Li, C.; Si, C. Green and Stable Piezoresistive Pressure Sensor Based on Lignin-Silver Hybrid Nanoparticles/Polyvinyl Alcohol Hydrogel. Int. J. Biol. Macromol. 2021, 176, 78–86. DOI: 10.1016/j.ijbiomac.2021.02.055.
- Xia, J.; Wang, X.; Zhang, J.; Kong, C.; Huang, W.; Zhang, X. Flexible Dual-Mechanism Pressure Sensor Based on Ag Nanowire Electrodes for Nondestructive Grading and Quality Monitoring of Fruits. ACS Appl. Nano Mater. 2022, 5, 10652–10662. DOI: 10.1021/acsanm.2c01968.
- Nela, L.; Tang, J.; Cao, Q.; Tulevski, G.; Han, S.-J. Large-Area High-Performance Flexible Pressure Sensor with Carbon Nanotube Active Matrix for Electronic Skin. Nano Lett. 2018, 18, 2054–2059. DOI: 10.1021/acs.nanolett.8b00063.
- Feng, C.; Yi, Z.; Jin, X.; Seraji, S. M.; Dong, Y.; Kong, L.; Salim, N. Solvent Crystallization-Induced Porous Polyurethane/Graphene Composite Foams for Pressure Sensing. Compos B Eng. 2020, 194, 108065. DOI: 10.1016/j.compositesb.2020.108065.
- Liu, Y.; Zheng, H.; Liu, M. High Performance Strain Sensors Based on Chitosan/Carbon Black Composite Sponges. Mater. Des. 2018, 141, 276–285. DOI: 10.1016/j.matdes.2017.12.046.
- Lei, X.; Ma, L.; Li, Y.; Cheng, Y.; Cheng, G. J.; Liu, F. Highly Sensitive and Wide-Range Flexible Pressure Sensor Based on Carbon Nanotubes-Coated Polydimethylsiloxane Foam. Mater. Lett. 2022, 308, 131151. DOI: 10.1016/j.matlet.2021.131151.
- Hwang, J.; Kim, Y.; Yang, H.; Oh, J. H. Fabrication of Hierarchically Porous Structured PDMS Composites and Their Application as a Flexible Capacitive Pressure Sensor. Compos B Eng. 2021, 211, 108607. DOI: 10.1016/j.compositesb.2021.108607.
- Chhetry, A.; Sharma, S.; Yoon, H.; Ko, S.; Park, J. Y. Enhanced Sensitivity of Capacitive Pressure and Strain Based on CaCu3Ti4O12 Wrapped Hybrid Sponge for Wearable Applications. Adv. Funct. Mater. 2020, 30, 1910020. DOI: 10.1002/adfm.201910020.
- Li, R.; Zhou, Q.; Bi, Y.; Cao, S.; Xia, X.; Yang, A.; Li, S.; Xiao, X. Research Progress of Flexible Capacitive Pressure Sensor for Sensitivity Enhancement Approaches. Sens. Actuators A Phys. 2021, 321, 112425. DOI: 10.1016/j.sna.2020.112425.
- Yoon, J. I.; Choi, K. S.; Chang, S. P. A Novel Means of Fabricating Microporous Structures for the Dielectric Layers of Capacitive Pressure Sensor. Microelectron. Eng. 2017, 179, 60–66. DOI: 10.1016/j.mee.2017.04.028.
- Wan, S.; Bi, H.; Zhou, Y.; Xie, X.; Su, S.; Yin, K.; Sun, L. Graphene Oxide as High-Performance Dielectric Materials for Capacitive Pressure Sensors. Carbon N. Y. 2017, 114, 209–216. DOI: 10.1016/j.carbon.2016.12.023.
- Kwon, D.; Lee, T.-I.; Shim, J.; Ryu, S.; Kim, M. S.; Kim, S.; Kim, T.-S.; Park, I. Highly Sensitive, Flexible, and Wearable Pressure Sensor Based on a Giant Piezocapacitive Effect of Three-Dimensional Microporous Elastomeric Dielectric Layer. ACS Appl. Mater. Interfaces. 2016, 8, 16922–16931. DOI: 10.1021/acsami.6b04225.
- Xu, D.; Zhang, H.; Pu, L.; Li, L. Fabrication of Poly(Vinylidene Fluoride)/Multiwalled Carbon Nanotube Nanocomposite Foam via Supercritical Fluid Carbon Dioxide: Synergistic Enhancement of Piezoelectric and Mechanical Properties. Compos Sci. Technol. 2020, 192, 108108. DOI: 10.1016/j.compscitech.2020.108108.
- Dai, S.-W.; Gu, Y.-L.; Zhao, L.; Zhang, W.; Gao, C.-H.; Wu, Y.-X.; Shen, S.-C.; Zhang, C.; Kong, T.-T.; Li, Y.-T.; et al. Bamboo-Inspired Mechanically Flexible and Electrically Conductive Polydimethylsiloxane Foam Materials with Designed Hierarchical Pore Structures for Ultra-Sensitive and Reliable Piezoresistive Pressure Sensor. Compos B Eng. 2021, 225, 109243. DOI: 10.1016/j.compositesb.2021.109243.
- Kim, D. H.; Jung, Y.; Jung, K.; Kwak, D. H.; Park, D. M.; Shin, M. G.; Tak, H. J.; Ko, J. S. Hollow Polydimethylsiloxane (PDMS) Foam with a 3D Interconnected Network for Highly Sensitive Capacitive Pressure Sensors. Micro Nano Syst. Lett. 2020, 8, 24. DOI: 10.1186/s40486-020-00127-8.
- Xu, M.; Gao, Y.; Yu, G.; Lu, C.; Tan, J.; Xuan, F. Flexible Pressure Sensor Using Carbon Nanotube-Wrapped Polydimethylsiloxane Microspheres for Tactile Sensing. Sens. Actuators A Phys. 2018, 284, 260–265. DOI: 10.1016/j.sna.2018.10.040.
- Xiong, Y.; Zhu, Y.; Liu, X.; Zhu, P.; Hu, Y.; Sun, R.; Wong, C.-P. A Flexible Pressure Sensor Based on Melamine Foam Capped by Copper Nanowires and Reduced Graphene Oxide. Mater. Today Commun. 2020, 24, 100970. DOI: 10.1016/j.mtcomm.2020.100970.
- Veeralingam, S.; Praveen, S.; Vemula, M.; Badhulika, S. One-Step Synthesis of Carbon-Doped PPy Nanoparticles Interspersed in 3D Porous Melamine Foam as a High-Performance Piezoresistive Pressure, Strain, and Breath Sensor. Mater. Chem. Front. 2022, 6, 570–579. DOI: 10.1039/D1QM01427G.
- Zhong, A.; Li, J.; Zhang, Y.; Zhang, F.; Wang, T.; Zhang, G.; Sun, R.; Wong, C.-P. Low Temperature Microwave Fabrication of Three-Dimensional Graphene/Polyimide Foams with Flexibility Strain Responsivity. Compos Part A Appl. Sci. Manuf. 2020, 137, 105995. DOI: 10.1016/j.compositesa.2020.105995.
- Yang, H.; Shang, J.-C.; Wang, W.-F.; Yang, Y.-F.; Yuan, Y.-N.; Lei, H.-S.; Fang, D.-N. Polyurethane Sponges-Based Ultrasensitive Pressure Sensor via Bioinspired Microstructure Generated by Pre-Strain Strategy. Compos Sci. Technol. 2022, 221, 109308. DOI: 10.1016/j.compscitech.2022.109308.
- Zhu, G.; Li, H.; Peng, M.; Zhao, G.; Chen, J.; Zhu, Y. Highly-Stretchable Porous Thermoplastic Polyurethane/Carbon Nanotubes Composites as a Multimodal Sensor. Carbon N. Y. 2022, 195, 364–371. DOI: 10.1016/j.carbon.2022.04.033.
- Wang, T.; Li, J.; Zhang, Y.; Liu, F.; Zhang, B.; Wang, Y.; Jiang, R.; Zhang, G.; Sun, R.; Wong, C. Highly Ordered 3D Porous Graphene Sponge for Wearable Piezoresistive Pressure Sensor Applications. Chemistry 2019, 25, 6378–6384. DOI: 10.1002/chem.201900014.
- Jothi, L.; Nageswaran, G. Plasma Modified Polymeric Materials for Biosensors/Biodevice Applications. In Non-Thermal Plasma Technology for Polymeric Materials; Elsevier: Oxford, UK, 2019; pp 409–437. DOI: 10.1016/B978-0-12-813152-7.00015-9.
- Bélanger, M.-C.; Marois, Y. Hemocompatibility, Biocompatibility, Inflammatory and in Vivo Studies of Primary Reference Materials Low-Density Polyethylene and Polydimethylsiloxane: A Review. J. Biomed. Mater. Res. 2001, 58, 467–477. DOI: 10.1002/jbm.1043.
- Lötters, J. C.; Olthuis, W.; Veltink, P. H.; Bergveld, P. The Mechanical Properties of the Rubber Elastic Polymer Polydimethylsiloxane for Sensor Applications. J. Micromech. Microeng. 1997, 7, 145–147. DOI: 10.1088/0960-1317/7/3/017.
- Mata, A.; Fleischman, A. J.; Roy, S. Characterization of Polydimethylsiloxane (PDMS) Properties for Biomedical Micro/Nanosystems. Biomed. Microdevices. 2005, 7, 281–293. DOI: 10.1007/s10544-005-6070-2.
- Wang, Q.; Chen, A.; Gu, H.; Qin, G.; Zhang, J.; Xu, J.; Jiang, G.; Liu, W.; Zhang, Z.; Huang, H. Highly Interconnected Porous PDMS/CNTs Sandwich Sponges with anti-Icing/Deicing Microstructured Surfaces. J. Mater. Sci. 2021, 56, 11723–11735. DOI: 10.1007/s10853-021-06052-4.
- Zhou, L.; Rada, J.; Zhang, H.; Song, H.; Mirniaharikandi, S.; Ooi, B. S.; Gan, Q. Sustainable and Inexpensive Polydimethylsiloxane Sponges for Daytime Radiative Cooling. Adv. Sci. (Weinh) 2021, 8, e2102502. DOI: 10.1002/advs.202102502.
- Pharino, U.; Sinsanong, Y.; Pongampai, S.; Charoonsuk, T.; Pakawanit, P.; Sriphan, S.; Vittayakorn, N.; Vittayakorn, W. Influence of Pore Morphologies on the Mechanical and Tribo-Electrical Performance of Polydimethylsiloxane Sponge Fabricated via Commercial Seasoning Templates. Radiat. Phys. Chem. 2021, 189, 109720. DOI: 10.1016/j.radphyschem.2021.109720.
- Pruvost, M.; Smit, W. J.; Monteux, C.; Poulin, P.; Colin, A. Polymeric Foams for Flexible and Highly Sensitive Low-Pressure Capacitive Sensors. Npj Flex. Electron. 2019, 3, 7. DOI: 10.1038/s41528-019-0052-6.
- Wang, H.; Zhang, R.; Yuan, D.; Xu, S.; Wang, L. Gas Foaming Guided Fabrication of 3D Porous Plasmonic Nanoplatform with Broadband Absorption, Tunable Shape, Excellent Stability, and High Photothermal Efficiency for Solar Water Purification. Adv. Funct. Mater. 2020, 30, 2003995. DOI: 10.1002/adfm.202003995.
- Drozdov, A. D.; Claville Christiansen, J. The Effect of Porosity on Elastic Moduli of Polymer Foams. J. Appl. Polymer Sci. 2020, 137, 48449. DOI: 10.1002/app.48449.
- Liu, C.; Le, L.; Zhang, M.; Ding, J. Tunable Large‐Scale Compressive Strain Sensor Based on Carbon Nanotube/Polydimethylsiloxane Foam Composites by Additive Manufacturing. Adv. Eng. Mater. 2022, 24, 2101337. DOI: 10.1002/adem.202101337.
- Timusk, M.; Nigol, I. A.; Vlassov, S.; Oras, S.; Kangur, T.; Linarts, A.; Šutka, A. Low-Density PDMS Foams by Controlled Destabilization of Thixotropic Emulsions. J. Colloid Interface Sci. 2022, 626, 265–275. DOI: 10.1016/j.jcis.2022.06.150.
- García-Valverde, M.; Chatzimitakos, T.; Lucena, R.; Cárdenas, S.; Stalikas, C. Melamine Sponge Functionalized with Urea-Formaldehyde Co-Oligomers as a Sorbent for the Solid-Phase Extraction of Hydrophobic Analytes. Molecules 2018, 23, 2595. DOI: 10.3390/molecules23102595.
- Khan, M. R. R.; An, T. K.; Lee, H. S. A Battery-Free, Chipless, Highly Sensitive LC Pressure Sensor Tag Using PEDOT: PSS and Melamine Foam. IEEE Sensors J. 2021, 21, 2184–2193. DOI: 10.1109/JSEN.2020.3021076.
- Cui, W.; Li, X.; Li, X.; Si, T.; Lu, L.; Ma, T.; Wang, Q. Thermal Performance of Modified Melamine Foam/Graphene/Paraffin Wax Composite Phase Change Materials for Solar-Thermal Energy Conversion and Storage. J Clean Prod 2022, 367, 133031. DOI: 10.1016/j.jclepro.2022.133031.
- Yan, L.; Fu, L.; Chen, Y.; Tian, H.; Xiang, A.; Rajulu, A. V. Improved Thermal Stability and Flame Resistance of Flexible Polyimide Foams by Vermiculite Reinforcement. J. Appl. Polym. Sci. 2017, 134, 44828. DOI: 10.1002/app.44828.
- Gu, W.; Wang, G.; Zhou, M.; Zhang, T.; Ji, G. Polyimide-Based Foams: Fabrication and Multifunctional Applications. ACS Appl. Mater. Interfaces. 2020, 12, 48246–48258. DOI: 10.1021/acsami.0c15771.
- Zhu, Z.; Yao, H.; Dong, J.; Qian, Z.; Dong, W.; Long, D. High-Mechanical-Strength Polyimide Aerogels Crosslinked with 4, 4′-Oxydianiline-Functionalized Carbon Nanotubes. Carbon N Y 2019, 144, 24–31. DOI: 10.1016/j.carbon.2018.11.057.
- Pan, L.-Y.; Shen, Y.-X.; Zhan, M.-S.; Wang, K.; Gao, D.-L. Visualization Study of Foaming Process for Polyimide Foams and Its Reinforced Foams. Polym. Compos. 2008, 31, NA–NA. DOI: 10.1002/pc.20764.
- Li, Y.; Liu, X.-Y.; Zhan, M.-S.; Wang, K. Effects of 3,4′-Oxydianiline on the Structures and Properties of a Novel Aromatic Polyimide Foam. J. Appl. Polym. Sci. 2012, 125, 4128–4134. DOI: 10.1002/app.35683.
- Liu, H.; Gao, J.; Huang, W.; Dai, K.; Zheng, G.; Liu, C.; Shen, C.; Yan, X.; Guo, J.; Guo, Z. Electrically Conductive Strain Sensing Polyurethane Nanocomposites with Synergistic Carbon Nanotubes and Graphene Bifillers. Nanoscale 2016, 8, 12977–12989. DOI: 10.1039/C6NR02216B.
- Liu, H.; Li, Y.; Dai, K.; Zheng, G.; Liu, C.; Shen, C.; Yan, X.; Guo, J.; Guo, Z. Electrically Conductive Thermoplastic Elastomer Nanocomposites at Ultralow Graphene Loading Levels for Strain Sensor Applications. J. Mater. Chem. C 2016, 4, 157–166. DOI: 10.1039/C5TC02751A.
- Lan, Y.; Liu, H.; Cao, X.; Zhao, S.; Dai, K.; Yan, X.; Zheng, G.; Liu, C.; Shen, C.; Guo, Z. Electrically Conductive Thermoplastic Polyurethane/Polypropylene Nanocomposites with Selectively Distributed Graphene. Polymer (Guildf) 2016, 97, 11–19. DOI: 10.1016/j.polymer.2016.05.017.
- Yilgör, I.; Yilgör, E.; Wilkes, G. L. Critical Parameters in Designing Segmented Polyurethanes and Their Effect on Morphology and Properties: A Comprehensive Review. Polymer (Guildf) 2015, 58, A1–A36. DOI: 10.1016/j.polymer.2014.12.014.
- Soto, M.; Sebastián, R. M.; Marquet, J. Photochemical Activation of Extremely Weak Nucleophiles: Highly Fluorinated Urethanes and Polyurethanes from Polyfluoro Alcohols. J. Org. Chem. 2014, 79, 5019–5027. DOI: 10.1021/jo5005789.
- Oppon, C.; Hackney, P. M.; Shyha, I.; Birkett, M. Effect of Varying Mixing Ratios and Pre-Heat Temperature on the Mechanical Properties of Polyurethane (PU) Foam. Proc. Eng. 2015, 132, 701–708. DOI: 10.1016/j.proeng.2015.12.550.
- Chen, K.-S.; Leon Yu, T.; Chen, Y.-S.; Lin, T.-L.; Liu, W.-J. Soft-and Hard-Segment Phase Segregation of Polyester-Based Polyurethane. J. Polym. Res. 2001, 8, 99–109. DOI: 10.1007/s10965-006-0139-3.
- Tang, Y. W.; Labow, R. S.; Santerre, J. P. Enzyme-Induced Biodegradation of Polycarbonate Polyurethanes: Dependence on Hard-Segment Concentration. J. Biomed. Mater. Res. 2001, 56, 516–528. DOI: 10.1002/1097-4636.
- Verdolotti, L.; Di Caprio, M. R.; Lavorgna, M.; Buonocore, G. G. Polyurethane Nanocomposite Foams. In Polyurethane Polymers; Jackson, D., Ed.; Elsevier: Oxford, UK, 2017; pp 277–310. DOI: 10.1016/B978-0-12-804065-2.00009-7.
- Ng, Z. C.; Roslan, R. A.; Lau, W. J.; Gürsoy, M.; Karaman, M.; Jullok, N.; Ismail, A. F. A Green Approach to Modify Surface Properties of Polyurethane Foam for Enhanced Oil Absorption. Polymers (Basel) 2020, 12, 1883. DOI: 10.3390/polym12091883.
- Tay, R. Y.; Li, H.; Lin, J.; Wang, H.; Lim, J. S. K.; Chen, S.; Leong, W. L.; Tsang, S. H.; Teo, E. H. T. Lightweight, Superelastic Boron Nitride/Polydimethylsiloxane Foam as Air Dielectric Substitute for Multifunctional Capacitive Sensor Applications. Adv. Funct. Materials 2020, 30, 1909604. DOI: 10.1002/adfm.201909604.
- Grande, J. B.; Fawcett, A. S.; McLaughlin, A. J.; Gonzaga, F.; Bender, T. P.; Brook, M. A. Anhydrous Formation of Foamed Silicone Elastomers Using the Piers–Rubinsztajn Reaction. Polymer (Guildf) 2012, 53, 3135–3142. DOI: 10.1016/j.polymer.2012.05.033.
- Mu, L.; Yue, X.; Hao, B.; Wang, R.; Ma, P.-C. Facile Preparation of Melamine Foam with Superhydrophobic Performance and Its System Integration with Prototype Equipment for the Clean-up of Oil Spills on Water Surface. Sci. Total Environ. 2022, 833, 155184. DOI: 10.1016/j.scitotenv.2022.155184.
- Patel, P. S.; Shepherd, D. E.; Hukins, D. W. Compressive Properties of Commercially Available Polyurethane Foams as Mechanical Models for Osteoporotic Human Cancellous Bone. BMC Musculoskelet. Disord. 2008, 9, 137. DOI: 10.1186/1471-2474-9-137.
- Cuenca, J.; Van der Kelen, C.; Göransson, P. A General Methodology for Inverse Estimation of the Elastic and Anelastic Properties of Anisotropic Open-Cell Porous Materials—with Application to a Melamine Foam. J. Appl. Phys. 2014, 115, 84904. DOI: 10.1063/1.4865789.
- Silcox, R.; Bolton, J. S.; Kim, N.; Cano, R.; Howerton, B. Development of Polyimide Foam for Aircraft Sidewall Applications. In 51st AIAA Aerospace Sciences Meeting Including the New Horizons Forum and Aerospace Exposition; 213. 2013. DOI: 10.2514/6.2013-213.
- Zhang, H.; Fan, X.; Chen, W.; Wang, Y.; Liu, C.; Cui, B.; Li, G.; Song, J.; Zhao, D.; Wang, D.; et al. A Simple and Green Strategy for Preparing Flexible Thermoplastic Polyimide Foams with Exceptional Mechanical, Thermal-Insulating Properties, and Temperature Resistance for High-Temperature Lightweight Composite Sandwich Structures. Compos B Eng. 2022, 228, 109405. DOI: 10.1016/j.compositesb.2021.109405.
- Teixeira, I.; Castro, I.; Carvalho, V.; Rodrigues, C.; Souza, A.; Lima, R.; Teixeira, S.; Ribeiro, J; University of Minho, Mechanical Engineering Department, University of Minho, Campus de Azurém, Guimarães, Portugal. Polydimethylsiloxane Mechanical Properties: A Systematic Review. AIMSMATES 2021, 8, 952–973. DOI: 10.3934/matersci.2021058.
- Jia, H.; Kong, Q.-Q.; Liu, Z.; Wei, X.-X.; Li, X.-M.; Chen, J.-P.; Li, F.; Yang, X.; Sun, G.-H.; Chen, C.-M. 3D Graphene/Carbon Nanotubes/Polydimethylsiloxane Composites as High-Performance Electromagnetic Shielding Material in X-Band. Compos Part A Appl. Sci. Manuf. 2020, 129, 105712. DOI: 10.1016/j.compositesa.2019.105712.
- Cao, X.; Liu, H.; Cai, J.; Chen, L.; Yang, X.; Liu, M. Chinese Ink Coated Melamine Foam with Joule Heating and Photothermal Effect for Strain Sensor and Seawater Desalination. Compos Part A Appl. Sci. Manuf. 2021, 149, 106535. DOI: 10.1016/j.compositesa.2021.106535.
- Li, C.; Ma, H.; Zhou, Z.; Xu, W.; Ren, F.; Yang, X. Preparation and Properties of Melamine-Formaldehyde Rigid Closed-Cell Foam Toughened by Ethylene Glycol/Carbon Fiber. Cell. Polym. 2021, 40, 55–72. DOI: 10.1177/0262489320929232.
- Shi, Y.; Hu, A.; Wang, Z.; Li, K.; Yang, S. Closed-Cell Rigid Polyimide Foams for High-Temperature Applications: The Effect of Structure on Combined Properties. Polymers (Basel) 2021, 13, 4434. DOI: 10.3390/polym13244434.
- Xu, M.; Yang, L.; Zhang, L.; Yan, S. Innovative Technologies for Printing and Packaging; Springer Nature, 2023; Vol. 991.
- Fan, L.; Wang, R.; Zhang, Q.; Liu, S.; He, R.; Zhang, R.; Shen, M.; Xiang, X.; Zhou, Y. In Situ Self-Foaming Preparation of Hydrophobic Polyurethane Foams for Oil/Water Separation. New J. Chem. 2021, 45, 13902–13908. DOI: 10.1039/D0NJ05208F.
- Borreguero, A. M.; Zamora, J.; Garrido, I.; Carmona, M.; Rodríguez, J. F. Improving the Hydrophilicity of Flexible Polyurethane Foams with Sodium Acrylate Polymer. Materials (Basel, Switzerland) 2021, 14, 2197. DOI: 10.3390/ma14092197.
- Trantidou, T.; Elani, Y.; Parsons, E.; Ces, O. Hydrophilic Surface Modification of PDMS for Droplet Microfluidics Using a Simple, Quick, and Robust Method via PVA Deposition. Microsyst. Nanoeng. 2017, 3, 16091. DOI: 10.1038/micronano.2016.91.
- Park, E. J.; Cho, Y. K.; Kim, D. H.; Jeong, M.-G.; Kim, Y. H.; Kim, Y. D. Hydrophobic Polydimethylsiloxane (PDMS) Coating of Mesoporous Silica and Its Use as a Preconcentrating Agent of Gas Analytes. Langmuir 2014, 30, 10256–10262. DOI: 10.1021/la502915r.
- Li, Q.; Sun, X.; Li, Y.; Xu, L. Hydrophobic Melamine Foam as the Solvent Holder for Liquid–Liquid Microextraction. Talanta 2019, 191, 469–478. DOI: 10.1016/j.talanta.2018.09.003.
- Akhiani, A. R.; Cornelis Metselaar, H. S.; Ang, B. C.; Mehrali, M.; Mehrali, M. Highly Hydrophobic Silanized Melamine Foam for Facile and Uniform Assembly of Graphene Nanoplatelet towards Efficient Light-to-Thermal Energy Storage. Mater. Today Energy 2022, 28, 101077. DOI: 10.1016/j.mtener.2022.101077.
- Kausar, A. Emerging Polyimide and Graphene Derived Nanocomposite Foam: Research and Technical Tendencies. J. Macromol. Sci. Part A 2021, 58, 643–658. DOI: 10.1080/10601325.2021.1934011.
- Gama, N.; Ferreira, A.; Barros-Timmons, A. Polyurethane Foams: Past, Present, and Future. Materials (Basel) 2018, 11, 1841. DOI: 10.3390/ma11101841.
- Hu, W.-J.; Xia, Q.-Q.; Pan, H.-T.; Chen, H.-Y.; Qu, Y.-X.; Chen, Z.-Y.; Zhang, G.-D.; Zhao, L.; Gong, L.-X.; Xue, C.-G.; Tang, L.-C. Green and Rapid Preparation of Fluorosilicone Rubber Foam Materials with Tunable Chemical Resistance for Efficient Oil–Water Separation. Polymers (Basel) 2022, 14, 1628. DOI: 10.3390/polym14081628.
- Jun, Y.-S.; Sy, S.; Ahn, W.; Zarrin, H.; Rasen, L.; Tjandra, R.; Amoli, B. M.; Zhao, B.; Chiu, G.; Yu, A. Highly Conductive Interconnected Graphene Foam Based Polymer Composite. Carbon N. Y. 2015, 95, 653–658. DOI: 10.1016/j.carbon.2015.08.079.
- Biron, M. Detailed Accounts of Thermoset Resins for Moulding and Composite Matrices. In Thermosets and Composites; Biron, M., Ed.; Woodhead Publishing: Oxford, UK, 2004; pp 183–327. DOI: 10.1016/B978-185617411-4/50006-1.
- Williams, M. K.; Holland, D. B.; Melendez, O.; Weiser, E. S.; Brenner, J. R.; Nelson, G. L. Aromatic Polyimide Foams: Factors That Lead to High Fire Performance. Polym. Degrad. Stab. 2005, 88, 20–27. DOI: 10.1016/j.polymdegradstab.2003.12.012.
- Guelcher, S. A.; Patel, V.; Gallagher, K. M.; Connolly, S.; Didier, J. E.; Doctor, J. S.; Hollinger, J. O. Synthesis and in Vitro Biocompatibility of Injectable Polyurethane Foam Scaffolds. Tissue Eng. 2006, 12, 1247–1259. DOI: 10.1089/ten.2006.12.1247.
- Singhal, P.; Rodriguez, J. N.; Small, W.; Eagleston, S.; Van de Water, J.; Maitland, D. J.; Wilson, T. S. Ultra Low Density and Highly Crosslinked Biocompatible Shape Memory Polyurethane Foams. J. Polym. Sci. B Polym. Phys. 2012, 50, 724–737. DOI: 10.1002/polb.23056.
- Victor, A.; Ribeiro, J.; F. Araújo, F, Sta Apolónia. Study of PDMS Characterization and Its Applications in Biomedicine: A Review. JMEB 2019, 4, 1–9. DOI: 10.24243/JMEB/4.1.163.
- Yan, C.; Luo, Y.; Zhang, W.; Zhu, Z.; Li, P.; Li, N.; Chen, Y.; Jin, T. Preparation of a Novel Melamine Foam Structure and Properties. J. Appl. Poly. Sci. 2022, 139, 51992. DOI: 10.1002/app.51992.
- Constantin, C. P.; Aflori, M.; Damian, R. F.; Rusu, R. D. Biocompatibility of Polyimides: A Mini-Review. Materials (Basel) 2019, 12, 3166. DOI: 10.3390/ma12193166.
- Rodrigues, I. C. P.; Woigt, L. F.; Pereira, K. D.; Luchessi, A. D.; Lopes, É. S. N.; Webster, T. J.; Gabriel, L. P. Low-Cost Hybrid Scaffolds Based on Polyurethane and Gelatin. J. Mater. Res. Technol. 2020, 9, 7777–7785. DOI: 10.1016/j.jmrt.2020.04.049.
- Tian, C.; Li, B.; Hu, X.; Wu, J.; Li, P.; Xiang, X.; Zu, X.; Li, S. Melamine Foam Derived 2H/1T MoS 2 as Flexible Interlayer with Efficient Polysulfides Trapping and Fast Li + Diffusion to Stabilize Li–S Batteries. ACS Appl. Mater. Interfaces. 2021, 13, 6229–6240. DOI: 10.1021/acsami.0c19725.
- ACS. Polyurethane Chemistry: Renewable Polyols and Isocyanates 2021, ACS Publications. DOI: 10.1021/bk-2021-1380.
- Wang, J.; Zhang, C.; Chen, D.; Sun, M.; Liang, N.; Cheng, Q.; Ji, Y.; Gao, H.; Guo, Z.; Li, Y.; et al. Fabrication of a Sensitive Strain and Pressure Sensor from Gold Nanoparticle-Assembled 3D-Interconnected Graphene Microchannel-Embedded PDMS. ACS Appl. Mater. Interfaces. 2020, 12, 51854–51863. DOI: 10.1021/acsami.0c16152.
- Zhang, X.; Xiang, D.; Zhu, W.; Zheng, Y.; Harkin-Jones, E.; Wang, P.; Zhao, C.; Li, H.; Wang, B.; Li, Y. Flexible and High-Performance Piezoresistive Strain Sensors Based on Carbon Nanoparticles@Polyurethane Sponges. Compos. Sci. Technol. 2020, 200, 108437. DOI: 10.1016/j.compscitech.2020.108437.
- Ma, C.; Zhou, R.; Xie, L. Recent Advances in Flexible Pressure/Strain Sensors Using Carbon Nanotubes. Int. J. Agric. Biol. Eng. 2022, 15, 1–12. DOI: 10.25165/j.ijabe.20221502.7364.
- Dai, H.; Thostenson, E. T. Large-Area Carbon Nanotube-Based Flexible Composites for Ultra-Wide Range Pressure Sensing and Spatial Pressure Mapping. ACS Appl. Mater. Interfaces. 2019, 11, 48370–48380. DOI: 10.1021/acsami.9b17100.
- Cao, M.; Su, J.; Fan, S.; Qiu, H.; Su, D.; Li, L. Wearable Piezoresistive Pressure Sensors Based on 3D Graphene. Chem. Eng. J. 2021, 406, 126777. DOI: 10.1016/j.cej.2020.126777.
- Huang, L.; Chen, J.; Xu, Y.; Hu, D.; Cui, X.; Shi, D.; Zhu, Y. Three-Dimensional Light-Weight Piezoresistive Sensors Based on Conductive Polyurethane Sponges Coated with Hybrid CNT/CB Nanoparticles. Appl. Surf. Sci. 2021, 548, 149268. DOI: 10.1016/j.apsusc.2021.149268.
- Ko, W.-Y.; Huang, L.-T.; Lin, K.-J. Green Technique Solvent-Free Fabrication of Silver Nanoparticle–Carbon Nanotube Flexible Films for Wearable Sensors. Sens. Actuators A Phys. 2021, 317, 112437. DOI: 10.1016/j.sna.2020.112437.
- Ma, L.; Lei, X.; Guo, X.; Wang, L.; Li, S.; Shu, T.; Cheng, G. J.; Liu, F. Carbon Black/Graphene Nanosheet Composites for Three-Dimensional Flexible Piezoresistive Sensors. ACS Appl. Nano Mater. 2022, 5, 7142–7149. DOI: 10.1021/acsanm.2c01081.
- Han, Z.; Li, H.; Xiao, J.; Song, H.; Li, B.; Cai, S.; Chen, Y.; Ma, Y.; Feng, X. Ultralow-Cost, Highly Sensitive, and Flexible Pressure Sensors Based on Carbon Black and Airlaid Paper for Wearable Electronics. ACS Appl. Mater. Interfaces. 2019, 11, 33370–33379. DOI: 10.1021/acsami.9b12929.
- Hu, J.; Yu, J.; Li, Y.; Liao, X.; Yan, X.; Li, L. Nano Carbon Black-Based High Performance Wearable Pressure Sensors. Nanomaterials (Basel) 2020, 10, 664. DOI: 10.3390/nano10040664.
- Czech, Z.; Kowalczyk, A.; Pełech, R.; Wróbel, R. J.; Shao, L.; Bai, Y.; Świderska, J. Using of Carbon Nanotubes and Nano Carbon Black for Electrical Conductivity Adjustment of Pressure-Sensitive Adhesives. Int. J. Adhes. Adhes. 2012, 36, 20–24. DOI: 10.1016/j.ijadhadh.2012.04.004.
- Natsuki, J. A Review of Silver Nanoparticles: Synthesis Methods, Properties and Applications. IJMSA. 2015, 4, 325. DOI: 10.11648/j.ijmsa.20150405.17.
- Gómez-Hernández, R.; Panecatl-Bernal, Y.; Méndez-Rojas, M. Á. High Yield and Simple One-Step Production of Carbon Black Nanoparticles from Waste Tires. Heliyon 2019, 5, e02139. DOI: 10.1016/j.heliyon.2019.e02139.
- Wu, D.; Wu, L.; Zhou, W.; Sun, Y.; Zhang, M. Relations between the Aspect Ratio of Carbon Nanotubes and the Formation of Percolation Networks in Biodegradable Polylactide/Carbon Nanotube Composites. J. Polym. Sci. B Polym. Phys. 2010, 48, 479–489. DOI: 10.1002/polb.21909.
- Wang, C.; Murugadoss, V.; Kong, J.; He, Z.; Mai, X.; Shao, Q.; Chen, Y.; Guo, L.; Liu, C.; Angaiah, S.; Guo, Z. Overview of Carbon Nanostructures and Nanocomposites for Electromagnetic Wave Shielding. Carbon N Y 2018, 140, 696–733. DOI: 10.1016/j.carbon.2018.09.006.
- Li, Q.; Zhong, B.; Zhang, W.; Jia, Z.; Jia, D.; Qin, S.; Wang, J.; Razal, J. M.; Wang, X. Ti 3 C 2 MXene as a New Nanofiller for Robust and Conductive Elastomer Composites. Nanoscale 2019, 11, 14712–14719. DOI: 10.1039/C9NR03661J.
- Yang, K.; Yin, F.; Xia, D.; Peng, H.; Yang, J.; Yuan, W. A Highly Flexible and Multifunctional Strain Sensor Based on a Network-Structured MXene/Polyurethane Mat with Ultra-High Sensitivity and a Broad Sensing Range. Nanoscale 2019, 11, 9949–9957. DOI: 10.1039/C9NR00488B.
- Hasan, M. M.; Hossain, M. M.; Chowdhury, H. K. Two-Dimensional MXene-Based Flexible Nanostructures for Functional Nanodevices: A Review. J. Mater. Chem. A 2021, 9, 3231–3269. DOI: 10.1039/D0TA11103A.
- Wu, Z.; Wei, L.; Tang, S.; Xiong, Y.; Qin, X.; Luo, J.; Fang, J.; Wang, X. Recent Progress in Ti3C2T x MXene-Based Flexible Pressure Sensors. ACS Nano. 2021, 15, 18880–18894. DOI: 10.1021/acsnano.1c08239.
- Alsharaeh, E. Polystyrene-Poly(Methyl Methacrylate) Silver Nanocomposites: Significant Modification of the Thermal and Electrical Properties by Microwave Irradiation. Materials (Basel) 2016, 9, 458. DOI: 10.3390/ma9060458.
- Saha, D. R.; Mandal, A.; Mitra, S.; Mada, M. R.; Boughton, P.; Bandyopadhyay, S.; Chakravorty, D. 2013 Nanoindentation Studies on Silver Nanoparticles. In AIP Conference Proceedings; American Institute of Physics; Vol. 1536, pp 257–258. DOI: 10.1063/1.4810198.
- Zhou, M.; Wei, Z.; Qiao, H.; Zhu, L.; Yang, H.; Xia, T. Particle Size and Pore Structure Characterization of Silver Nanoparticles Prepared by Confined Arc Plasma. J. Nanomater. 2009, 2009, 1–5. DOI: 10.1155/2009/968058.
- Wen, M.; Sun, X.; Su, L.; Shen, J.; Li, J.; Guo, S. The Electrical Conductivity of Carbon Nanotube/Carbon Black/Polypropylene Composites Prepared through Multistage Stretching Extrusion. Polymer (Guildf) 2012, 53, 1602–1610. DOI: 10.1016/j.polymer.2012.02.003.
- Li, Y.; Huang, X.; Zeng, L.; Li, R.; Tian, H.; Fu, X.; Wang, Y.; Zhong, W.-H. A Review of the Electrical and Mechanical Properties of Carbon Nanofiller-Reinforced Polymer Composites. J. Mater. Sci. 2019, 54, 1036–1076. DOI: 10.1007/s10853-018-3006-9.
- Kaptakov, M. O. Investigation of Effective Mechanical Characteristics of Nanomodified Carbon-Epoxide Composite by Numerical and Analytical Methods. TURCOMAT 2021, 12, 535–541. DOI: 10.17762/turcomat.v12i5.1049.
- Deng, L.; Eichhorn, S. J.; Kao, C.-C.; Young, R. J. The Effective Young’s Modulus of Carbon Nanotubes in Composites. ACS Appl. Mater. Interfaces. 2011, 3, 433–440. DOI: 10.1021/am1010145.
- Poirier, E.; Chahine, R.; Bénard, P.; Cossement, D.; Lafi, L.; Mélançon, E.; Bose, T. K.; Désilets, S. Storage of Hydrogen on Single-Walled Carbon Nanotubes and Other Carbon Structures. Appl. Phys. A 2004, 78, 961–967. DOI: 10.1007/s00339-003-2415-y.
- Wang, S.; Tian, Y.; Hang, C.; Wang, C. Cohesively Enhanced Electrical Conductivity and Thermal Stability of Silver Nanowire Networks by Nickel Ion Bridge Joining. Sci. Rep. 2018, 8, 5260. DOI: 10.1038/s41598-018-21777-0.
- Jing, G. Y.; Duan, H. L.; Sun, X. M.; Zhang, Z. S.; Xu, J.; Li, Y. D.; Wang, J. X.; Yu, D. P. Surface Effects on Elastic Properties of Silver Nanowires: Contact Atomic-Force Microscopy. Phys. Rev. B 2006, 73, 235409. DOI: 10.1103/PhysRevB.73.235409.
- Lee, J.-U.; Yoon, D.; Cheong, H. Estimation of Young’s Modulus of Graphene by Raman Spectroscopy. Nano Lett. 2012, 12, 4444–4448. DOI: 10.1021/nl301073q.
- Stoller, M. D.; Park, S.; Zhu, Y.; An, J.; Ruoff, R. S. Graphene-Based Ultracapacitors. Nano Lett. 2008, 8, 3498–3502. DOI: 10.1021/nl802558y.
- Shayesteh Zeraati, A.; Mirkhani, S. A.; Sun, P.; Naguib, M.; Braun, P. V.; Sundararaj, U. Improved Synthesis of Ti 3 C 2 T x MXenes Resulting in Exceptional Electrical Conductivity, High Synthesis Yield, and Enhanced Capacitance. Nanoscale 2021, 13, 3572–3580. DOI: 10.1039/D0NR06671K.
- Firestein, K. L.; von Treifeldt, J. E.; Kvashnin, D. G.; Fernando, J. F. S.; Zhang, C.; Kvashnin, A. G.; Podryabinkin, E. V.; Shapeev, A. V.; Siriwardena, D. P.; Sorokin, P. B.; Golberg, D. Young’s Modulus and Tensile Strength of Ti3C2 MXene Nanosheets as Revealed by in Situ TEM Probing, AFM Nanomechanical Mapping, and Theoretical Calculations. Nano Lett. 2020, 20, 5900–5908. DOI: 10.1021/acs.nanolett.0c01861.
- Yang, S.-Y.; Lin, W.-N.; Huang, Y.-L.; Tien, H.-W.; Wang, J.-Y.; Ma, C.-C. M.; Li, S.-M.; Wang, Y.-S. Synergetic Effects of Graphene Platelets and Carbon Nanotubes on the Mechanical and Thermal Properties of Epoxy Composites. Carbon N. Y. 2011, 49, 793–803. DOI: 10.1016/j.carbon.2010.10.014.
- Liu, L.; Zhang, J.; Zhao, J.; Liu, F. Mechanical Properties of Graphene Oxides. Nanoscale 2012, 4, 5910–5916. DOI: 10.1039/c2nr31164j.
- Zhang, S.; Wang, H.; Liu, J.; Bao, C. Measuring the Specific Surface Area of Monolayer Graphene Oxide in Water. Mater. Lett. 2020, 261, 127098. DOI: 10.1016/j.matlet.2019.127098.
- Boland, C. S.; Khan, U.; Binions, M.; Barwich, S.; Boland, J. B.; Weaire, D.; Coleman, J. N. Graphene-Coated Polymer Foams as Tuneable Impact Sensors. Nanoscale 2018, 10, 5366–5375. DOI: 10.1039/C7NR09247D.
- Moosa, A. A.; Sa, A. R.; Ibrahim, M. N. Mechanical and Electrical Properties of Graphene Nanoplates and Carbon-Nanotubes Hybrid Epoxy Nanocomposites. Am. J. Mater. Sci. 2016, 6, 157–165. DOI: 10.5923/j.materials.20160606.03.
- Li, Z.-F.; Zhang, H.; Liu, Q.; Sun, L.; Stanciu, L.; Xie, J. Fabrication of High-Surface-Area Graphene/Polyaniline Nanocomposites and Their Application in Supercapacitors. ACS Appl. Mater. Interfaces. 2013, 5, 2685–2691. DOI: 10.1021/am4001634.
- P, A.; Naina Mohamed, S.; Singaravelu, D. L.; Brindhadevi, K.; Pugazhendhi, A. A Review on Graphene/Graphene Oxide Supported Electrodes for Microbial Fuel Cell Applications: Challenges and Prospects. Chemosphere 2022, 296, 133983. DOI: 10.1016/j.chemosphere.2022.133983.
- Dobson, J. F.; Gould, T.; Vignale, G. How Many-Body Effects Modify the Van Der Waals Interaction between Graphene Sheets. Phys. Rev. X 2014, 4, 021040. DOI: 10.1103/PhysRevX.4.021040.
- Tsoi, S.; Dev, P.; Friedman, A. L.; Stine, R.; Robinson, J. T.; Reinecke, T. L.; Sheehan, P. E. Van Der Waals Screening by Single-Layer Graphene and Molybdenum Disulfide. ACS Nano. 2014, 8, 12410–12417. DOI: 10.1021/nn5050905.
- Sumfleth, J.; Buschhorn, S. T.; Schulte, K. Comparison of Rheological and Electrical Percolation Phenomena in Carbon Black and Carbon Nanotube Filled Epoxy Polymers. J. Mater. Sci. 2011, 46, 659–669. DOI: 10.1007/s10853-010-4788-6.
- Song, P.; Song, J.; Zhang, Y. Stretchable Conductor Based on Carbon Nanotube/Carbon Black Silicone Rubber Nanocomposites with Highly Mechanical, Electrical Properties and Strain Sensitivity. Compos B Eng. 2020, 191, 107979. DOI: 10.1016/j.compositesb.2020.107979.
- Atif, R.; Inam, F. Reasons and Remedies for the Agglomeration of Multilayered Graphene and Carbon Nanotubes in Polymers. Beilstein J. Nanotechnol. 2016, 7, 1174–1196. DOI: 10.3762/bjnano.7.109.
- Mohan, V. B.; Brown, R.; Jayaraman, K.; Bhattacharyya, D. Characterisation of Reduced Graphene Oxide: Effects of Reduction Variables on Electrical Conductivity. Mater. Sci. Eng. 2015, 193, 49–60. DOI: 10.1016/j.mseb.2014.11.002.
- Davis, J. R.; Tucker, R. C. Handbook Thermal Spray Technology, ASM International: Materials Park, OH, 2004. DOI: 10.31399/asm.hb.v05a.9781627081719.
- Karthikeyan, J. Cold Spray Technology: The Cold Spray Process Has the Potential to Reduce Costs and Improve Quality in Both Coatings and Freeform Fabrication of near-Net-Shape Parts. Adv. Mater. Processes 2005, 163, 33–36.
- Assadi, H.; Gärtner, F.; Stoltenhoff, T.; Kreye, H. Bonding Mechanism in Cold Gas Spraying. Acta Mater. 2003, 51, 4379–4394. DOI: 10.1016/S1359-6454(03)00274-X.
- Mattox, D. M. Vacuum Deposition, Reactive Evaporation, and Gas Evaporation. In Surface Engineering; Sedriks, A. J., Ed.; ASM International: Materials Park, OH, ASM International, 1994; pp 556–572. DOI: 10.31399/asm.hb.v05.a0001287.
- Cramer, S. D.; Covino, B. S. Jr, Corrosion: Fundamentals, Testing, and Protection, Volume 13A, ASM Handbook. J. Therm. Spray Technol. 2003, 12, 459.
- Guo, Q.; Ghadiri, R.; Weigel, T.; Aumann, A.; Gurevich, E.; Esen, C.; Medenbach, O.; Cheng, W.; Chichkov, B.; Ostendorf, A. Comparison of in Situ and Ex Situ Methods for Synthesis of Two-Photon Polymerization Polymer Nanocomposites. Polymers (Basel) 2014, 6, 2037–2050. DOI: 10.3390/polym6072037.
- Chun, S.; Hong, A.; Choi, Y.; Ha, C.; Park, W. A Tactile Sensor Using a Conductive Graphene-Sponge Composite. Nanoscale 2016, 8, 9185–9192. DOI: 10.1039/C6NR00774K.
- Huang, Y.; Zhang, J.; Pu, J.; Guo, X.; Qiu, J.; Ma, Y.; Zhang, Y.; Yang, X. Resistive Pressure Sensor for High-Sensitivity e-Skin Based on Porous Sponge Dip-Coated CB/MWCNTs/SR Conductive Composites. Mater. Res. Express 2018, 5, 065701. DOI: 10.1088/2053-1591/aac8c0.
- Krebs, F. C. Fabrication and Processing of Polymer Solar Cells: A Review of Printing and Coating Techniques. Sol. Energy Mater. Sol. Cells 2009, 93, 394–412. DOI: 10.1016/j.solmat.2008.10.004.
- Zhang, P.; Chen, Y.; Li, Y.; Zhang, Y.; Zhang, J.; Huang, L. Erratum: A Flexible Strain Sensor Based on the Porous Structure of a Carbon Black/Carbon Nanotube Conducting Network for Human Motion Detection (Sensors, (2020). 20, 1154, 10.3390/S20041154). Sensors (Switzerland) 2020, 20, 2901. DOI: 10.3390/s20102901.
- Aziz, F.; Ismail, A. F. Spray Coating Methods for Polymer Solar Cells Fabrication: A Review. Mater. Sci. Semicond. Process 2015, 39, 416–425. DOI: 10.1016/j.mssp.2015.05.019.
- Paszkiewicz, S.; Szymczyk, A. Graphene-Based Nanomaterials and Their Polymer Nanocomposites. In Nanomaterials and Polymer Nanocomposites; Elsevier, 2019; pp 177–216. DOI: 10.1016/B978-0-12-814615-6.00006-0.
- Hu, Z.; Xin, Y.; Fu, Q. Ultrahigh Sensitivity and Wide Strain Range of Porous Pressure Sensor Based on Binary Conductive Fillers by in-Situ Polymerization. J. Polym. Res. 2021, 28, 134. DOI: 10.1007/s10965-021-02484-3.
- Behrisch, R.; Wittmaack, K. Sputtering by Particle Bombardment I. In Topics in Applied Physics, Behrisch, R. ed., Springer: Berlin, 1981; Vol. 47. DOI: 10.1007/3-540-10521-2.
- Tewari, A.; Gandla, S.; Bohm, S.; McNeill, C. R.; Gupta, D. Highly Exfoliated MWNT–RGO Ink-Wrapped Polyurethane Foam for Piezoresistive Pressure Sensor Applications. ACS Appl. Mater. Interfaces. 2018, 10, 5185–5195. DOI: 10.1021/acsami.7b15252.
- Ma, Z.; Wei, A.; Ma, J.; Shao, L.; Jiang, H.; Dong, D.; Ji, Z.; Wang, Q.; Kang, S. Lightweight, Compressible and Electrically Conductive Polyurethane Sponges Coated with Synergistic Multiwalled Carbon Nanotubes and Graphene for Piezoresistive Sensors. Nanoscale 2018, 10, 7116–7126. DOI: 10.1039/c8nr00004b.
- Zhong, W.; Ding, X.; Li, W.; Shen, C.; Yadav, A.; Chen, Y.; Bao, M.; Jiang, H.; Wang, D. Facile Fabrication of Conductive Graphene/Polyurethane Foam Composite and Its Application on Flexible Piezo-Resistive Sensors. Polymers (Basel) 2019, 11, 1289. DOI: 10.3390/polym11081289.
- Liu, Q.; Liu, Y.; Shi, J.; Liu, Z.; Wang, Q.; Guo, C. F. High-Porosity Foam-Based Iontronic Pressure Sensor with Superhigh Sensitivity of 9280 KPa − 1. Nanomicro. Lett. 2022, 14, 21. DOI: 10.1007/s40820-021-00770-9.
- Beccatelli, M.; Villani, M.; Gentile, F.; Bruno, L.; Seletti, D.; Nikolaidou, D. M.; Culiolo, M.; Zappettini, A.; Coppedè, N. All-Polymeric Pressure Sensors Based on PEDOT:PSS-Modified Polyurethane Foam. ACS Appl. Polym. Mater. 2021, 3, 1563–1572. DOI: 10.1021/acsapm.0c01389.
- Wang, L.; Wang, D.; Wu, Z.; Luo, J.; Huang, X.; Gao, Q.; Lai, X.; Tang, L.-C.; Xue, H.; Gao, J. Self-Derived Superhydrophobic and Multifunctional Polymer Sponge Composite with Excellent Joule Heating and Photothermal Performance for Strain/Pressure Sensors. ACS Appl. Mater. Interfaces. 2020, 12, 13316–13326. DOI: 10.1021/acsami.0c00150.
- Li, X. P.; Li, Y.; Li, X.; Song, D.; Min, P.; Hu, C.; Zhang, H. B.; Koratkar, N.; Yu, Z. Z. Highly Sensitive, Reliable and Flexible Piezoresistive Pressure Sensors Featuring Polyurethane Sponge Coated with MXene Sheets. J. Colloid Interface Sci. 2019, 542, 54–62. DOI: 10.1016/j.jcis.2019.01.123.
- Wang, X.; Li, H.; Wang, T.; Niu, X.; Wang, Y.; Xu, S.; Jiang, Y.; Chen, L.; Liu, H. Flexible and High-Performance Piezoresistive Strain Sensors Based on Multi-Walled Carbon Nanotubes@Polyurethane Foam. RSC Adv. 2022, 12, 14190–14196. DOI: 10.1039/D2RA01291J.
- Nabeel, M.; Varga, M.; Kuzsella, L.; Fiser, B.; Vanyorek, L.; Viskolcz, B. The Effect of Pore Volume on the Behavior of Polyurethane-Foam-Based Pressure Sensors. Polymers (Basel) 2022, 14, 3652. DOI: 10.3390/polym14173652.
- Feng, D.; Xu, D.; Wang, Q.; Liu, P. Highly Stretchable Electromagnetic Interference (EMI) Shielding Segregated Polyurethane/Carbon Nanotube Composites Fabricated by Microwave Selective Sintering. J. Mater. Chem. C 2019, 7, 7938–7946. DOI: 10.1039/C9TC02311A.
- Zhai, Y.; Yu, Y.; Zhou, K.; Yun, Z.; Huang, W.; Liu, H.; Xia, Q.; Dai, K.; Zheng, G.; Liu, C.; Shen, C. Flexible and Wearable Carbon Black/Thermoplastic Polyurethane Foam with a Pinnate-Veined Aligned Porous Structure for Multifunctional Piezoresistive Sensors. Chem. Eng. J. 2020, 382, 122985. DOI: 10.1016/j.cej.2019.122985.
- Lü, X.; Yu, T.; Meng, F.; Bao, W. Wide‐Range and High‐Stability Flexible Conductive Graphene/Thermoplastic Polyurethane Foam for Piezoresistive Sensor Applications. Adv. Mater. Technol. 2021, 6, 2100248. DOI: 10.1002/admt.202100248.
- Gai, C.; Li, D.; Zhang, X.; Zhang, H.; Li, N.; Zheng, X.; Wu, D.; Sun, J. Scalable Fabrication of Flexible Single‐Layer Strain and Double‐Layer Pressure Sensors by Inkjet Printing for Subtle Vibration Detection. Adv. Mater. Inter. 2021, 8, 2100632. DOI: 10.1002/admi.202100632.
- Mersch, J.; Winger, H.; Nocke, A.; Cherif, C.; Gerlach, G. Experimental Investigation and Modeling of the Dynamic Resistance Response of Carbon Particle‐Filled Polymers. Macro. Materials Eng. 2020, 305, 2000361. DOI: 10.1002/mame.202000361.
- Azizkhani, M. B.; Kadkhodapour, J.; Rastgordani, S.; Anaraki, A. P.; Shirkavand Hadavand, B. Highly Sensitive, Stretchable Chopped Carbon Fiber/Silicon Rubber Based Sensors for Human Joint Motion Detection. Fibers Polym. 2019, 20, 35–44. DOI: 10.1007/s12221-019-8662-0.
- Lv, B.; Chen, X.; Liu, C. A Highly Sensitive Piezoresistive Pressure Sensor Based on Graphene Oxide/Polypyrrole@Polyurethane Sponge. Sensors (Basel) 2020, 20, 1219. DOI: 10.3390/s20041219.
- Nabeel, M.; Varga, M.; Kuzsela, L.; Filep, Á.; Fiser, B.; Viskolcz, B.; Kollar, M.; Vanyorek, L. Preparation of Bamboo-Like Carbon Nanotube Loaded Piezoresistive Polyurethane-Silicone Rubber Composite. Polymers (Basel) 2021, 13, 2144. DOI: 10.3390/polym13132144.
- Zhao, L.; Qiang, F.; Dai, S.-W.; Shen, S.-C.; Huang, Y.-Z.; Huang, N.-J.; Zhang, G.-D.; Guan, L.-Z.; Gao, J.-F.; Song, Y.-H.; Tang, L.-C. Construction of Sandwich-like Porous Structure of Graphene-Coated Foam Composites for Ultrasensitive and Flexible Pressure Sensors. Nanoscale 2019, 11, 10229–10238. DOI: 10.1039/C9NR02672J.
- Qiang, F.; Hu, L.-L.; Gong, L.-X.; Zhao, L.; Li, S.-N.; Tang, L.-C. Facile Synthesis of Super-Hydrophobic, Electrically Conductive and Mechanically Flexible Functionalized Graphene Nanoribbon/Polyurethane Sponge for Efficient Oil/Water Separation at Static and Dynamic States. Chem. Eng. J. 2018, 334, 2154–2166. DOI: 10.1016/j.cej.2017.11.054.
- Zhao, X.; Meng, F.; Peng, Y. Flexible and Highly Pressure-Sensitive Ternary Composites-Wrapped Polydimethylsiloxane Sponge Based on Synergy of Multi-Dimensional Components. Compos B Eng. 2022, 229, 109466. DOI: 10.1016/j.compositesb.2021.109466.
- Wu, X.; Han, Y.; Zhang, X.; Zhou, Z.; Lu, C. Large-Area Compliant, Low-Cost, and Versatile Pressure-Sensing Platform Based on Microcrack-Designed Carbon Black@Polyurethane Sponge for Human–Machine Interfacing. Adv. Funct. Mater. 2016, 26, 6246–6256. DOI: 10.1002/adfm.201601995.
- Ding, Y.; Xu, T.; Onyilagha, O.; Fong, H.; Zhu, Z. Recent Advances in Flexible and Wearable Pressure Sensors Based on Piezoresistive 3D Monolithic Conductive Sponges. ACS Appl. Mater. Interfaces. 2019, 11, 6685–6704. DOI: 10.1021/acsami.8b20929.
- Chen, X.; Li, R.; Niu, G.; Xin, M.; Xu, G.; Cheng, H.; Yang, L. Porous Graphene Foam Composite-Based Dual-Mode Sensors for Underwater Temperature and Subtle Motion Detection. Chem. Eng. J. 2022, 444, 136631. DOI: 10.1016/j.cej.2022.136631.
- Sencadas, V.; Tawk, C.; Alici, G. Highly Sensitive Soft Foam Sensors to Empower Robotic Systems. Adv. Mater. Technol. 2019, 4, 1900423. DOI: 10.1002/admt.201900423.
- Xu, B.; Ye, F.; Chen, R.; Luo, X.; Xue, Z.; Li, R.; Chang, G. A Supersensitive Wearable Sensor Constructed with PDMS Porous Foam and Multi-Integrated Conductive Pathways Structure. Ceram. Int. 2023, 49, 4641–4649. DOI: 10.1016/j.ceramint.2022.09.351.
- Cheng, L.; Feng, J. Facile Fabrication of Stretchable and Compressible Strain Sensors by Coating and Integrating Low-Cost Melamine Foam Scaffolds with Reduced Graphene Oxide and Poly (Styrene-b-Ethylene-Butylene-b-Styrene). Chem. Eng. J. 2020, 398, 125429. DOI: 10.1016/j.cej.2020.125429.
- Huang, L.; Wang, H.; Zhan, D.; Fang, F. Flexible Capacitive Pressure Sensor Based on Laser-Induced Graphene and Polydimethylsiloxane Foam. IEEE Sensors J. 2021, 21, 12048–12056. DOI: 10.1109/JSEN.2021.3054985.
- Yang, J.; Ye, Y.; Li, X.; Lü, X.; Chen, R. Flexible, Conductive, and Highly Pressure-Sensitive Graphene-Polyimide Foam for Pressure Sensor Application. Compos Sci. Technol. 2018, 164, 187–194. DOI: 10.1016/j.compscitech.2018.05.044.
- Hashim, A.; Hadi, A. Novel Pressure Sensors Made from Nanocomposites (Biodegradable Polymers–Metal Oxide Nanoparticles): Fabrication and Characterization. Ukr. J. Phys. 2018, 63, 754. DOI: 10.15407/ujpe63.8.754.
- Zheng, S.; Wu, X.; Huang, Y.; Xu, Z.; Yang, W.; Liu, Z.; Huang, S.; Xie, B.; Yang, M. Highly Sensitive and Multifunctional Piezoresistive Sensor Based on Polyaniline Foam for Wearable Human-Activity Monitoring. Compos Part A Appl. Sci. Manuf. 2019, 121, 510–516. DOI: 10.1016/j.compositesa.2019.04.014.
- Sengupta, D.; Pei, Y.; Kottapalli, A. G. P. Ultralightweight and 3D Squeezable Graphene-Polydimethylsiloxane Composite Foams as Piezoresistive Sensors. ACS Appl. Mater. Interfaces. 2019, 11, 35201–35211. DOI: 10.1021/acsami.9b11776.
- Jiang, H.; Zhang, J.; Qin, M.; Zhang, J.; Zou, X.; Weng, X. A Flexible Piezoresistive Strain Sensor Based on Black Phosphorus/Gold Nanocomposites Interspersed Sponge for Motion Sensing. Sens. Actuators A Phys. 2023, 356, 114359. DOI: 10.1016/j.sna.2023.114359.
- Wan, Y.; Jiang, H.; Ren, Y.; Liu, Y.; Zhang, L.; Lei, Q.; Zhu, D.; Liu, J.; Zhang, X.; Ma, N.; Cong, X. Photothermal Self-Healable Polypyrrole-Polyurethane Sponge with Dynamic Covalent Oximino Bonds for Flexible Strain Sensors. Eur. Polym. J. 2023, 193, 112097. DOI: 10.1016/j.eurpolymj.2023.112097.
- Li, K.; Yang, W.; Shen, Z.; Zhang, X.; Yi, M. Flexible Graphene Pressure Sensor Based on Sponge Sewn with Cotton. Sens Actuators A Phys. 2023, 354, 114266. DOI: 10.1016/j.sna.2023.114266.
- Wang, L.; Wang, H.; Xiao, W.; Wan, Q.; Gao, J. Mechanically Durable and Amphiphobic Rubber Foam Composites for Strain/Pressure Sensors. Compos. Commun. 2023, 40, 101590. DOI: 10.1016/j.coco.2023.101590.
- Lin, X.; Wu, F.; He, Y.; Liu, M. Flexible and Wearable Strain–Temperature Sensors Based on Chitosan/Ink Sponges. Molecules 2023, 28, 4083. DOI: 10.3390/molecules28104083.
- Xu, B.; Ye, F.; Chen, R.; Luo, X.; Chang, G.; Li, R. A Wide Sensing Range and High Sensitivity Flexible Strain Sensor Based on Carbon Nanotubes and MXene. Ceram. Int. 2022, 48, 10220–10226. DOI: 10.1016/j.ceramint.2021.12.235.
- Luo, R.; Cui, Y.; Li, H.; Wu, Y.; Du, B.; Zhou, S.; Hu, J. Fragmented Graphene Aerogel/Polydimethylsiloxane Sponges for Wearable Piezoresistive Pressure Sensors. ACS Appl. Nano Mater. 2023, 6, 7065–7076. DOI: 10.1021/acsanm.3c01285.
- Li, Y.; Cui, Y.; Zhang, M.; Li, X.; Li, R.; Si, W.; Sun, Q.; Yu, L.; Huang, C. Ultrasensitive Pressure Sensor Sponge Using Liquid Metal Modulated Nitrogen-Doped Graphene Nanosheets. Nano Lett. 2022, 22, 2817–2825. DOI: 10.1021/acs.nanolett.1c04976.
- Li, B.; Luo, J.; Huang, X.; Lin, L.; Wang, L.; Hu, M.; Tang, L.; Xue, H.; Gao, J.; Mai, Y. W. A Highly Stretchable, Super-Hydrophobic Strain Sensor Based on Polydopamine and Graphene Reinforced Nanofiber Composite for Human Motion Monitoring. Compos B Eng. 2020, 181, 107580. DOI: 10.1016/j.compositesb.2019.107580.
- Wang, Q.; Huang, X.; Han, F.; Wu, Y.; Wang, L.; Dai, H.; Song, P.; Tang, L.; Gao, J. Superhydrophobic, Biocompatible and Durable Nanofiber Composite with an Asymmetric Structure for Anisotropic Strain Sensing and Body Motion Detection. Chem. Eng. J. 2022, 450, 137899. DOI: 10.1016/j.cej.2022.137899.
- Li, S.-N.; He, X.-F.; Zeng, Z.-F.; Jiang, B.; Wu, Q.; Gong, L.-X.; Li, Y.; Bae, J.; Wang, S.; Tang, L.-C. Mechanically Ductile, Ionically Conductive and Low-Temperature Tolerant Hydrogel Enabled by High-Concentration Saline towards Flexible Strain Sensor. Nano Energy 2022, 103, 107789. DOI: 10.1016/j.nanoen.2022.107789.
- Li, S. N.; Li, B.; Yu, Z. R.; Gong, L. X.; Xia, Q. Q.; Feng, Y.; Jia, D.; Zhou, Y.; Tang, L. C. Chitosan in-Situ Grafted Magnetite Nanoparticles toward Mechanically Robust and Electrically Conductive Ionic-Covalent Nanocomposite Hydrogels with Sensitive Strain-Responsive Resistance. Compos Sci. Technol. 2020, 195, 108173. DOI: 10.1016/j.compscitech.2020.108173.
- Wu, L.; Luo, J.; Li, Y.; Zhang, W.; Wang, L.; Huang, X.; Xiao, W.; Tang, L.; Gao, J. Emulsion Dipping Based Superhydrophobic, Temperature Tolerant, and Multifunctional Coatings for Smart Strain Sensing Applications. Compos Sci. Technol. 2021, 216, 109045. DOI: 10.1016/j.compscitech.2021.109045.