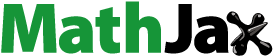
ABSTRACT
In tropical forests, the shade provided by tree canopies and extreme climate causes inhibition of plant seedling growth due to the lack of light. However, the plants can acclimate to such environmental stress by generating specific responses. The present study aimed to investigate the effects of shading conditions on ecophysiological performance of Narra seedlings (Pterocarpus indicus L.) via a mesocosm experiment. A pot experiment was conducted for 20 weeks in a greenhouse with different shading treatments, 75% (control), 25%, and 4% of full sunlight (FS). As a result, the photosynthetic rate (PN), Rubisco enzyme activity, maximum carboxylation rate (VCmax), and maximum electron transport rate (Jmax) in 25% FS treatment were higher or similar to those in control after three weeks of the beginning of shade treatment, whereas the highest values after ten weeks were observed in control. In contrast, the photosynthetic pigments were highest in control after three weeks, while the values were highest in 25% FS treatment after ten weeks. The growth parameters, such as biomass and leaf area, were highest in 75% FS treatment. The expression of Rubisco, phosphoglycerate kinase, glyceraldehyde-3-phosphate dehydrogenase, and fructose-1,6-bisphosphatase were up-regulated in 4% FS treatment compared to control after ten weeks, contributing to tolerating the shade stress. Our findings indicated the capacity of P. indicus seedlings to tolerate and acclimate low light conditions causing shade stress by generating specific physiological and morphological responses, especially Rubisco enzyme activity as well as gene expression related to photosynthetic activity. The present study will improve our understanding of the tolerance mechanism of Narra plant under light-deficient conditions, thereby providing a better strategy for efficiently growing seedlings of this species in tropical rainforests.
Introduction
Sunlight is the most crucial natural resource for plant growth and survival. However, in the terrestrial ecosystem, varying spectral environments can modify the quantity and quality of light, contributing to vegetation diversity and abundance. In tropical rainforests, competition in the absorption of light resources among plant species commonly occurs due to differences in their height. Such unintended blockage of light by the canopy of tall plants can cause abiotic stress (i.e., shade) to the plants with a lesser height, which is partly responsible for tree mortality.Citation1 As per the estimations of Hiromi et al.Citation2 and Kenzo et al.,Citation3 there were significant differences in irradiance between canopy layer (1,800 μmol m−2 s−1) and understory (5–10 μmol m−2 s−1) in the tropical rainforest. Because of sunlight block caused by the canopy of tall trees, plants with a lesser height present in the dim understory rely on sun flecks for their survival, which accounts for up to 60–90% of the total daily photon flux density.Citation4 In addition, the sun fleck can be responsible for up to 65% of the total daily carbon gain via photosynthesis, albeit not always constant.Citation4
Photosynthesis is a crucial factor that regulates plant growth and productivity. Light governs the rate of photosynthesis in leaves and also regulates the structure and function of the photosynthetic apparatus.Citation5 Light deficiency caused by shade restricts the capacity of plants to capture sunlight and in turn reduces the photosynthetic capacity, thereby degrading the whole plant growth processes from germination and seedling development to florescence.Citation6 A decrease in PSII quantum efficiency and reaction center, electron transport, and ATPase and Rubisco activities etc. due to shade stress is strongly associated to the low photosynthetic capacity and efficiency.Citation7,Citation8 Additionally, the abiotic stress causes photoinhibition or photodamage of PSI or PSII, resulting in the loss of carbon gain in plants.Citation4,Citation9 To acclimate to inappropriate light conditions, therefore, plants adjust the photosynthetic apparatus by altering carbon reduction cycle-related enzymes, electron transport components, proteins, and pigmentsCitation8, thereby obtaining as much light energy as needed to fix CO2 and accumulate carbohydrates.Citation10,Citation11 In addition to physiological response, the ability of plants to acclimate to insufficient light environment can display with morphological or genetic responses to enhance the efficiency of light capture and maximize carbon gain via photosynthesis.Citation12–15 However, it varies greatly with plant species traits and environmental conditions.Citation14 So far, to determine such plant performance in low light environment, various parameters of morphology, anatomy, physiology, phenology, and genetics have been assessed in many studies to improve understanding of shade-tolerant mechanism in plants.Citation5,Citation9,Citation14 However, most of these previous studies had been conducted for short-term or under field conditions primarily affected by diverse environmental factors. Hence, for a more precise understanding of each tree species, a long-term study on how plant seedlings acclimate to shade stress in the environment where edaphic and biotic factors are controlled is required.Citation16
Narra plant (Pterocarpus indicus L.) is native to tropical and temperate Asia, including the Philippines, India, Borneo, and the Pacific region, Celebes, New Guinea, and the Caroline Islands.Citation17,Citation18 However, there has been a significant decline in its habitat distribution due to deforestation, illegal logging, and cuttingCitation18 as well as climate change.Citation19 Currently, this species is listed as endangered on the IUCN Red List of Threatened Species, thus P. indicus is gaining attention for reforestation projects in several tropical countries, including Philippines.Citation20 In particular, the Narra plants have a capacity to store nitrogen and other major nutrients in soil,Citation18 contributing to improvement of forest soil quality and forest ecosystem sustainability. There is also a high interest in cultivating this species due to its high market value as a timber.Citation21,Citation22 Thus, such benefits are expected to contribute to the conservation of tropical forests and the revival of the timber industry through afforestation of this species. However, in the forest environment, blocking sunlight by tree canopies and extreme climate impact induce a decrease in the viability of plant seedlings, affecting the efficiency of tree farming and reforestation. According to previous studies, such tropical plant species, particularly at the seedling stage, require the least quantity of light via the photosynthetic apparatus under a shaded environment for their survival and fitness,Citation23,Citation24 and this phenomenon can be explained by irradiance altering the physiological and biochemical responses, gene expression, and biomass of plants.Citation24 Therefore, it is necessary to study the environmental (i.e., shading) and biological variables that are inappropriate for seedling growth not only for genetic conservation and management, but also for commercial purpose. This study aimed to examine the acclimation ability of P. indicus seedlings to different shaded treatments via physiological responses, including photosynthesis, the performance of Rubisco enzyme activity, and biomass, and identify gene expressions related to the photosynthetic mechanism and potential acclimation capacity under different shade environments.
Material and methods
Mesocosm study
A pot experiment was performed in a greenhouse at the University of Seoul from spring to early autumn. For this study, one three-year-old seedling of Narra (P. indicus), a nitrogen-fixing species, was planted in each pot (diameter: 15.5 cm and height: 19.8 cm). All pots were filled with artificial soil that consisted of perlite, vermiculite, and peat moss (1:1:1 = v:v:v). The soil substrates contained ammonium (NH4–N: 150 mg L−1), nitrate (NO3–N: 200–350 mg L−1), and phosphate (PO4–P: 200–350 mg L−1). Before shading treatment, the plants were allowed to acclimate to the surrounding environment of the glasshouse for a week. Net houses (height: 2 m, length: 6 m, width: 1.5 m, spectral range: 400–700 nm) covered with black shade net were installed in the glasshouse.
The shading treatments were set up depending on the thickness of the shade net: 75% (control), 25%, and 4% of full sunlight (FS) irradiance levels. The variations of photosynthetic photon flux density (PPFD) in accordance with the seasonal effect and shading treatment were measured using an LI-190SA sensor; the recorded data for PPFD are shown in . During the plant growth period (five months), water was supplied every two days. Also, the temperature in the glasshouse was maintained between 25°Ϲ and 35°Ϲ over the period. Each treatment consists of five replicate pots.
Table 1. Temporal difference in values of photosynthetic photon flux density (PPFD; μmol m−2 s−1) depending on different light intensities of full sunlight (FS) for 20 weeks cultivation in a glasshouse.
Photosynthesis rate
The net photosynthetic rate (PN), stomatal conductance (Gs), transpiration rate (Tr), and water use efficiency (WUE) were measured using an XT LI-6400 portable photosynthesis system equipped with a leaf chamber fluorometer (Li-Cor Inc., Lincoln, NE, USA). The fully expanded leaves from each plant’s middle to upper parts were randomly selected and measured at three different times: three, ten, and twenty weeks from the beginning date of shading treatment. Before measurements, the leaves were acclimated for 2 min at each PPFD, including 0, 20, 40, 60, 80, 100, 300, 500, 800, 1200, 1500, and 2000 μmol mol−1. The ambient CO2 concentration (Ca) and vapor pressure deficit were set up as 380 μmol mol−1 and 2.0 ± 0.4 kPa, respectively. The photosynthetic WUE was calculated as follows:Citation25 WUE = PN (μmol CO2 m−2 s−1)/Tr (mmol H2O m−2 s−1).
Photosynthetic pigments
Chlorophyll (Chl) and carotenoid (Car) in the leaves after three, ten, and twenty weeks from the beginning date of the shade treatment were determined quantitatively. The fresh leaves (0.1 g) of P. indicus seedlings were sampled at each time point and placed in a glass tube containing 10 mL of 80% acetone. The tube was stored in a refrigerator at 4°C for seven days, and the extract was measured at three different wavelengths (663, 645, and 480 nm) with a UV spectrophotometer (Shimadzu UV–160A, Shimadzu Co., Japan). Chl a, Chl b, Chl (a + b), Chl a:b, Car, and Chl (a + b)/Car were estimated by the following formula:Citation26
Chl. a = 12.7×A663 – 2.69×A645
Chl. b = 22.9×A645 – 4.68×A663
Total Chl (a + b) = 20.29×A645 + 8.02×A663
Chl a/b = Chl a/Chl b
Total Car = (1,000×A470 – 1.82×Chl a – 85.02 × Chl b)/198
Leaf gas exchange measurement
To estimate the photosynthetic capacity of P. indicus seedlings, the net carbon fixation (A/Ci) was calculated with PN to intercellular CO2 assimilation (Ci) detected using an XT LI-6400 infra-red gas analyzer equipped with a leaf chamber fluorometer (Li-Cor Inc., USA). The ambient conditions were similar to the Philippines environment as described by Baek and Woo:Citation27 temperature (25 ± 1°C), humidity (68%), and CO2 concentration (380 ppm). The fourth and sixth leaves selected from the upper part of each plant were clamped for 10–15 min at 50 µmol mol−1 CO2 to achieve maximum stomatal conductance before the measurement. The PN in the leaves was measured for 2 min at different intercellular CO2 concentrations, including 0, 50, 100, 150, 200, 400, 600, 800, and 1000 µmol mol−1. Rubisco maximum carboxylation rate (VCmax) and daytime respiration (Rd) were calculated by nonlinear regression from the data of the PN-Ci curve for 0 < Ci <200 µmol mol−1, and maximum electron transport rate (Jmax) was calculated from the PN-Ci curves by fitting the equation given below to a near-plateau (Ci > 600 µmol mol−1) based on Farquhar et al.Citation28 The values of Γ*, Kc, and Ko at the measured leaf temperatures were calculated with the temperature dependence equations and parameters from Bernacchi et al.Citation29 and Onoda et al.Citation30 Rd was assumed to be 0.02 of VCmax.Citation30
Where Ac is the PN limited by Rubisco activity (µmol m−2 s−1)
Ci is the intercellular CO2 concentration (µmol mol−1)
Kc and Ko indicate Michaelis – Menten constants of Rubisco activity for CO2 and O2, respectively (Kc = 405 µmol mol−1, Ko = 278 mmol mol−1)
O is the intercellular O2 concentration (mmol mol−1)
Γ* is the CO2 compensation point (42.8 µmol mol−1)
Rd = 0.02*VCmax
Aj is the PN limited by RuBP regeneration (µmol m−2 s−1)
Rubisco enzyme activity
Rubisco enzyme in the leaves was extracted with a modified method of Kasai (2008)Citation31 and Lu et al. (2009)Citation32. Fresh leaves (0.1 g) of P. indicus seedlings were collected after three and ten weeks during the pot experiment. The leaves were homogenized with liquid nitrogen in a mortar and pestle. The extraction was carried out with a buffer solution (1.5 mL) containing 50 mM Tris-HCl (pH 7.5), 1 mM EDTA, 10 mM MgCl2, 1% (w/w) polyvinyl pyrrolidine, 12% (v/v) glycerol, and 0.1% (v/v) β-mercaptoethanol. After centrifugation at 15,000 for 15 min at 4°C (Micro 17TR, Hanil Science Ltd., Korea), an assay buffer containing 50 mM HEPES-KOH (pH 8.0), 1 mM Na2-EDTA, 2.5 mM DTT, 10 mM NaHCO3, 20 mM MgCl2, 5 mM creatine phosphate, and 0.15 mM NADH was added into the homogenate, followed by storage for 10 min at 25°C. Then, a response buffer [10 unit PGK, 10 unit GAPDH, and 10 unit phosphocreatine kinase] was added. To measure Rubisco enzyme activity, change or decrease in absorbance at 340 nm during the initial 90 s was monitored using a UV 160A spectrophotometer.
Where A = ∆ (Absorbance at 340 nm)
TV = Total assay volume (µL)
F = Dilution factor from sample preparation
Ɛ = Extinction coefficient for NADH at 340 nm (6.3 µmol−1 cm−1)
d = Light path (cm)
SV = Sample volume (µL)
SG = Sample weight (g)
T = ∆Time
Plant growth parameters
Following five months of cultivation, all plants were harvested to measure their growth. The leaves, shoots, and roots were separated. Various plant growth parameters were recorded as follows: fresh weight (FW), dry weight (DW), leaf area (LA), specific leaf area (SLA; the ratio of leaf area to leaf dry mass), leaf mass ratio (LMR; total leaf mass to the entire plant biomass), and root mass ratio (RMR). Before DW measurement, the samples were oven-dried for three days at 85°C.
RNA extraction, cDNA synthesis, and quantitative RT-PCR
The total RNA was extracted from the fifth leaves from the top side of P. indicus seedlings sampled in the tenth week (summer season) after acclimating to shading treatments (75%, 25%, and 4% FS) using the cetyltrimethylammonium bromide (CTAB) method modification (Kim and Hamada 2005)Citation33. First-strand cDNA was synthesized from 1 µg of total RNA using reverse transcriptase (MMLV Reverse Transcriptase, Clontech, USA) and oligo dT primer. After the RT reaction was performed for 2 h at 42°C, 1 µL of RT reaction mixture was used as a template in 50 µL PCR-amplification reaction mixture composed of SYBR Green Master Mix (Takara, Japan), and 10 pmol of each primer. The tubulin gene was used as control and the primers used for RT-PCR analysis are listed in . The PCR reaction was run for 35 cycles of 30 s at 94°C, 30 s at 60°C, and 1 min at 72°C, with a 5 min final extension at 72°C. The amplified PCR products were visualized by electrophoresis on 1% agarose gel.
Table 2. Forward and reverse primers of P. indicus for photosynthesis-related genes such as Rubisco, FBPase, GAPDH, PGK, and tubulin (control).
Protein extraction and 2-dimensional gel electrophoresis
The leaf protein of P. indicus seedlings was extracted with Mg/NP-40 buffer, including 0.5 M Tris-HCl (pH 8.3), 2% v/v NP-40, 20 mM MgCl2, 5% β-mercaptoethanol, 1 mM phenylmethylsulfonyl fluoride, and 1% (w/v) polyvinyl polypyrrolidone and fractionated with polyethylene glycol (PEG) 4000 after twenty weeks (autumn season). The protocol for in-gel digestion and MALDI TOF-MS followed that provided by Kim et al. (2007).Citation34
Statistical analysis
The data for plant physiological parameters were statistically analyzed using a SAS software package (Systat 9.13, Systat Software Inc., Richmond, USA), with one-way analysis of variance (ANOVA) and Fisher’s least significance difference (LSD) post-hoc test to identify differences in the parameters among shade treatments (p < 0.05).
Results
Photosynthesis parameters in response to shading treatments
After three weeks of shading, the maximum rate of photosynthesis (PNmax) at a saturating light intensity of 2,000 μmol m−2 s−1 was highest in 25% FS treatment (6.46 μmol m−2 s−1), followed by 75% and 4% FS treatments (). On the other hand, after ten weeks, the highest PNmax was found in 75% FS (5.87 μmol m−1 s−1), followed by 25% and 4% FS treatments. Indeed, over ten weeks, the PNmax value increased in control while decreasing in shade treatments of 25% and 4% FS treatments. After twenty weeks, there was no difference in the PNmax values among all the treatments.
Figure 1. Variation of the net photosynthesis rates (PN) of P. indicus upon photosynthetic photon flux density (PPFD) among different shade treatments including 75%, 25%, and 4% of full sunlight (FS). The PN was measured at the 3rd week, 10th week, and 20th week from the beginning of shading treatment. Data represent the mean of the PN values at each PPFD ± standard errors (n = 6).

The Gs values of P. indicus seedlings grown in 75% and 25% FS treatments after three weeks were 0.04 and 0.05 mmol m−2 s−1, respectively, and higher than that in 4% FS treatment (p < 0.05; ). Likewise, the Gs value was similar for the 75% and 25% FS treatments after ten weeks. On the other hand, there was no significant difference in the Gs value among different shade treatments after twenty weeks (p > 0.05).
Table 3. Differences in photosynthetic parameters, such as stomatal conductance (Gs), transpiration rate (Tr), and water use efficiency (WUE), in leaves of P. indicus at different light intensities including 75%, 25%, and 4% of full sunlight (FS) during the pot experiment. Values in brackets represent standard errors of the means (n = 6). Same letters within each measurement time indicate no significant difference among the shade treatments (LSD, p < 0.05).
The transpiration rate (Tr) of P. indicus seedlings was higher in 25% FS than in 75% and 4% FS treatments after three weeks (p < 0.05; ). After ten weeks, the Tr value was similar for 75% and 25% FS treatments, accounting for over 1.1 mmol m−2 s−1. Overall, the Tr values of the seedlings after ten weeks were higher than those after three weeks. After twenty weeks, there was no significant difference in the Tr value among the three shade treatments (p > 0.05). Unlike the PN, Gs, and Tr trends, WUE had no significant difference in shade treatments during the experiment ().
Variation of pigment contents
There was a similar trend that the concentrations of Chl (a + b) and Car did not differ among the shade treatments after three weeks, whereas those in 25% FS were higher than those in other treatments after ten weeks (p < 0.05; ). The highest ratio of chlorophyll a to b was found in 75% FS treatment after three and ten weeks (). On the other hand, the highest ratio of chlorophyll to carotenoid was in 4% FS, followed by 25% and 75% FS treatments after three weeks (). As with the ratio of Chl a/b, the lowest ratio of Chl (a + b)/Car was in 25% FS after ten weeks. In general, after twenty weeks, there were no significant differences in those values related to photosynthetic pigments from the three shade treatments (p > 0.05).
Figure 2. Concentrations of photosynthetic pigments in leaves of P. indicus at different light intensities including 75%, 25%, and 4% of full sunlight (FS) during the pot experiment: chlorophyll (chl) (a+b), carotenoid (car), chl a/b ratio, and chl (a+b)/Car ratio. Values indicate the mean ± standard errors (n = 6). Same letters represent no significant difference among different shade treatments of each season (LSD, p < 0.05).

Photosynthetic carbon assimilation
For the concentration of intercellular CO2, a clear difference among different shade treatments was observed after ten weeks, but not three and twenty weeks (). Compared to two shade treatments, the plants in 75% FS as control had an elevated value of the Ci when measured after ten weeks (). On the other hand, after three and twenty weeks, the Ci values of plant leaves in 75% and 25% FS treatments were similar but higher than that in 4% FS treatment ().
Figure 3. Variation of the net photosynthetic rate (PN) of P. indicus upon different intercellular CO2 levels (Ci) among different light intensities including 75%, 25%, and 4% of full sunlight (FS). The PN was measured at the 3rd week, 10th week, and 20th week from the beginning of shade treatment. The Ci concentrations were ranged from 0 to 1,000 [μmol CO2 mol−1]. Data represent the means of the PN value at each Ci concentration ± standard errors (n = 6).
![Figure 3. Variation of the net photosynthetic rate (PN) of P. indicus upon different intercellular CO2 levels (Ci) among different light intensities including 75%, 25%, and 4% of full sunlight (FS). The PN was measured at the 3rd week, 10th week, and 20th week from the beginning of shade treatment. The Ci concentrations were ranged from 0 to 1,000 [μmol CO2 mol−1]. Data represent the means of the PN value at each Ci concentration ± standard errors (n = 6).](/cms/asset/892e9329-fc55-48fa-81f6-8f0d1e128a0c/kpsb_a_2245625_f0003_b.gif)
Rubisco enzyme activity
Depending on light intensity and shading duration, Rubisco enzyme activity varied (). The highest values of Rubisco activity were found in 75% FS after ten weeks. In addition, the value of Rubisco enzyme in leaves sampled after ten weeks was higher than after three weeks. Over time, the Rubisco enzymatic activity increased 2.8, 1.3, and 2.5 times in 75%, 25%, and 4% FS treatments, respectively.
Figure 4. Rubisco activity of P. indicus leaves under different light intensities including 75%, 25%, and 4% of full sunlight (FS) at the 3rd week and 10th week from the beginning of shade treatment. Values indicates the means ± standard errors (n = 3). Same letters of each season represent no significant difference among the shade treatments (LSD, p < 0.05).

The VCmax and Jmax in the leaves of P. indicus seedlings grown at different light intensities are shown in . The values of VCmax and Jmax varied by shading duration. Both values in 75% and 25% FS treatments increased from the 3rd week to 10th week but decreased at the 20th week. Overall, both values at the third and twentieth weeks were similar, but there was a significant difference among the treatments (p < 0.05). On the other hand, after ten weeks, the VCmax and Jmax considerably differed by light intensity. In particular, VCmax responded highly to the degree of light intensity after ten weeks ().
Table 4. Differences in the maximum rate of RuBP carboxylation capacity (VCmax), the maximum electron transport rate driving RuBP regeneration (Jmax), and the ratio of Jmax to VCmax (J/V) in leaves of P. indicus at different light intensities including 75%, 25%, and 4% of full sunlight (FS) during the pot experiment. Values in brackets indicate standard errors of the mean (n = 3). Same letters within each measurement time and column represent no significant difference among the shading treatments (LSD, p < 0.05).
Plant growth characteristics
The growth of P. indicus seedlings was affected by shade treatments (, ). The values of FW, DW, and LA in 75% FS were higher than those in 25% and 4% FS treatments (p < 0.05). The three growth factors decreased with a decrease in light intensities, but there was no significant difference between the shading treatments (p > 0.05). Such a tendency was found in LMR. Meanwhile, SLA showed an opposite trend; it increased with a decrease in light intensities (). The SLA in 4% FS was higher by around 85% than in 75% FS treatment. RMR did not significantly differ among the three shading conditions (p > 0.05). The plant height of P. indicus seedlings decreased with a decrease in light intensities (). The height was higher (about 32%) in 75% FS than in 4% FS (p < 0.05), while the diameter representing no significant difference among all treatments (p > 0.05).
Figure 5. Height and diameter of P. indicus seedlings grown in 75%, 25%, and 4% of full sunlight (FS). Bars indicate means ± standard errors (n = 6). Same letters of each parameter represent no significant difference among the shade treatments (LSD, p < 0.05).

Table 5. Growth characteristics of P. indicus seedlings grown for 20 weeks under different light treatments, including 75%, 25%, and 4% of full sunlight (FS). Values in blankets indicate standard errors of the means (n = 9). The variables include fresh weight (FW), dry weight (DW), leaf area (LA), specific leaf area (SLA), leaf mass ratio (LMR), and root mass ratio (RMR). Same letters in each column indicate no significant difference among the treatments (LSD, p < 0.05).
Photosynthetic gene and proteome expression
The results of key photosynthesis-related genes expression pattern under different shade treatments are shown in . The most shaded treatment, 4% FS, had higher levels of Rubisco, PGK, GAPDH, and FBPase by 117%, 202%, 96%, and 82%, respectively, compared to 75% FS after ten weeks. The changes in the identified leaf proteins of P. indicus seedlings grown in different shade treatments were observed (Supplemental Figure S1). We compared the expression difference in the total photosynthetic proteome extracted from 2-DE in the different shade treatments. Ten proteins were identified (see Supplemental Table S1); four expressed spots (#1, #2, #3, and #4) were predominantly expressed in 75% FS. Another four expressed spots (#5, #6, #7, and #8) were found in 25% FS, and the other two spot numbers (#9 and #10) were prominent in 4% FS.
Figure 6. Transcription analysis of photosynthesis related genes including tubulin (control), ribulose 1, 5-bisphosphate carboxylase/oxygenase (Rubisco), 3-phosphoglycerate kinase (PGK), glyceraldehyde 3-phosphate dehydrogenase (GAPDH), and fructose 1, 6-bisphosphate aldolase (FBPase) by semiquantitative RT-PCR. Total RNA was isolated from P. indicus leaves at the 10th week from the beginning of shade treatments including 75%, 25%, and 4% of full sunlight (FS).

Discussion
In this study, the difference in light intensities by shade treatment varied its impacts on the physiological response of three-year-old seedlings of P. indicus. Also, the effect of these difference in light intensity on plant characteristics tended to become more pronounced along the shading duration. Photosynthesis is strongly associated with light intensity, contributing to photosynthetic performance and plant production.Citation35 In this study, with an increase in light intensity, the PN of unshaded seedlings alone increased after ten weeks (). This indicated that lower intensities of the light via shade treatment resulted in the limitation of carbon gain in the leaves of P. indicus and that the amount of photon quantum yields in PSII might be decreased.Citation36 However, a higher value of PN was observed after shade treatment of 25% FS than 75% FS at the third week, suggesting that the plants acclimation to the moderate shade conditions might be attributed to enhanced photosynthetic adaptation triggered by high carbon acquisition.Citation37,Citation38 A similar observation was reported by BoardmanCitation39 and Krause et al.,Citation40 where the shade conditions enhanced the electron transport capacity and photon yield within the chloroplasts for increasing enzyme activity related to photosynthetic response. Moreover, under moderate shade H2O in the leaves is essential in fixing CO2 uptake by exporting carbon for photosynthetic induction, resulting in a quick stomatal opening within shading leaves.Citation41 This is in agreement with the reports of the present study, where stomatal conductance and transpiration rate were higher in 25% FS than in 75% FS treatment after three weeks (). Likewise, the WUE was higher under the shaded condition maybe due to the maintenance of water capacity to compensate for photosynthesis damage.Citation36 On the other hand, the lower WUE under natural sunlight of 75% FS is attributed to reductions in Gs and Tr (),Citation42 limiting CO2 uptake and water consumption within stomata. The results indicated that, under shade conditions, carbon gain and water consumption of P. indicus leaves might be balanced by enhancing tolerance to the abiotic stress.
The effect of enhanced tolerance to shade stress was found in photosynthetic pigments, in particular, after ten weeks (). Such an effect in the pigments, i.e., total Chl (a+b), occurred in the moderate shade treatment, indicating that P. indicus could increase chlorophyll pigments at low irradiance, leading to maximizing light-harvesting capacity.Citation5,Citation42,Citation43 The shade leaves of P. indicus seedlings have different chlorophyll concentrations depending on the light intensity; the thinner shade leaves contain more significant amounts of chlorophyll.Citation44 Carotenoid and Chl a/b ratio, which are indicators of the photosynthetic activity of the plant leaves,Citation45 have several physiological functions associated with abiotic stress.Citation46 In this study, we found an enhanced Car content in the shaded leaves of P. indicus seedlings, particularly in 25% FS (). Such an increase in the pigment molecule in response to shade stress may be attributed to the expansion of the light-harvesting capacity of the antenna of the photosystem ІІ (P680) in the chloroplast.Citation47,Citation48 As such, the Car status in the plant leaves has been considered as an essential index for shade tolerance ability.Citation49,Citation50 On the other hand, the low ratio of Chl a/b in the shade treatment was related to an increase in Chl b content, indicating that leaves of P. indicus seedlings might optimize light absorption by enhancing electron transport efficiency.Citation13 and increasing the light harvest complex (LHC ІІ) proteins in the reaction center.Citation51 An increase in carotenoid content in the leaves is correlated with an increase in the chlorophyll a/b ratio.Citation46 Furthermore, the results for the chlorophyll (a+b): carotenoid ratio showed that leaves grown in 4% FS possessed a higher value ratio in comparison with 75% and 25% FS after three and ten weeks, respectively (). This result can be explained by the P. indicus leaves in complete sunlight treatment having fewer light-harvesting chlorophyll proteins and a more significant number of reaction center pigment proteins, such as CPa and CP I, on a total chlorophyll basis compared with the shaded leaves.Citation51,Citation52 The lower ratio of Chl/Car in FS also reflects that a higher proportion of carotenoids might protect the chlorophyll damage from either photooxidation or ultraviolet radiation.Citation53
The high levels of photosynthetic parameters, such as Gs, Tr, VCmax, and Jmax in plants grown in 25% FS shading treatment after three weeks were found, indicating that the tree species can have an acclimation of photosynthesis to the moderate shade stress (). Ten weeks after, VCmax and Jmax tended to increase in 75% and 25% FS treatments but decrease in 4% FS, suggesting a significant shade stress impact. In addition, the VCmax and Jmax values where decreased in all the shade treatments at the 20th week (), indicating that the autumn temperature may affect the photosynthetic and leaf biochemistry dynamics.Citation54 Such decrease in VCmax and Jmax by shading and seasonal cold effects can limit PN, leading to a decrease in nitrogen partitioning to Rubisco.Citation55,Citation56
Rubisco in plants play a crucial role in carbon acquisition in the “light-independent reactions” of photosynthesis and photorespiratory oxygen consumption.Citation57,Citation58 However, Rubisco content in plants is considerably reduced during shade acclimation, together with alterations in leaf’s area, chlorophyll, and nitrogen content.Citation59 Likewise, in this study, the shaded leaves of P. indicus had lower Rubisco activity than the unshaded leaves (). In general, the amount of Rubisco enzyme and light-saturated photosynthesis in leaves have a co-relationship.Citation60 Hence, when P. indicus seedlings were grown in FS, the RuBP regeneration and phosphorylation regeneration capacity in the Calvin cycle could have high light energy efficiency via ATP and NADPH electron transports in the thylakoid membrane of the chloroplast.Citation61,Citation62 On the other hand, in shaded treatments, the amount of ATP and NADPH contributing to Rubisco enzyme activity and the availability of CO2 assimilation might decrease in the Calvin cycle.Citation62 Additionally, the lower Rubisco content in shaded leaves may be derived from low investment in photosynthetic protein yield and a low light-saturated PN.Citation63 In particular, a greater reduction in Rubisco activity caused by shading treatment was found after ten weeks when the intensity of sunlight was strong across the present experiment (), accordingly influencing overall plant growth and characteristics.
Plants can respond to change in light intensity by adjusting morphological characteristics. In this study, 75% FS treatment, the highest light intensity, resulted in higher values in the growth parameters, such as FW, DW, and LA, than the other two shading treatments (). Additionally, the higher the light intensity, the higher LMR can be explained by the leaves grown in full sunlight having thicker leaves, a higher PN, and a higher quantum yield capacity than shaded leaves.Citation63 A similar pattern in those was found in Australian rainforest species.Citation64 On the other hand, the lowest value of RMR was observed in control, and it tended to have an inverse relationship with LMR (). Generally, an increased LMR in a high irradiance environment correlates with the reduced biomass allocated toward root mass, resulting in water limitation.Citation64,Citation65 Salgado-Luarte and GianoliCitation66 explained that shaded leaves have a small amount (number) of branches, longer internodes, and petioles, and the leaf blade is horizontally stretched. Furthermore, the results in relation to height and the constant value of diameter are in accordance with Kelly et al.Citation64 and Zhao et al.Citation67 An increase in shoot height has a positive co-relationship with an increase in light intensity,Citation68,Citation69 contributing to an increase in PN, active strength of plants, and high CO2 assimilation rate in full sunlight condition.Citation67,Citation69,Citation70 However, under light-deficient conditions plants can be stimulated to have a light harvest capacity by enlarging their leaf size for higher photosynthesis.Citation63,Citation71,Citation72 Among the growth parameters, SLA is considered as an index of morphological response, including leaf structure, thickness, and mechanical tissues, to changing light conditions.Citation73,Citation74 As reported by Liu et al.,Citation14 plants grown in shaded condition have a higher SLA, which aids improve the efficiencies of light capture and carbon gain. Likewise, SLA of P. indicus seedlings increased as the light intensities decreased in this study (). Similarly, Lichtenthaler et al.Citation45 demonstrated that higher SLA in leaves of ash, hornbeam, maple, and linden trees on the lower canopy compared to those on the upper canopy.
We also found the molecular response of P. indicus seedlings to shade stress. The expression of photosynthetic genes, including Rubisco, GAPDH, FBPase, and PGK, differed by light intensities (). All genes in the leaves of P. indicus seedlings were up-regulated in the most shaded treatments of 4% FS compared to the other two treatments. This suggests that this tree species can have a high tolerance against shade stress, by facilitating the Calvin cycle with increased gene activation related to Rubisco, GAPDH, and FBPase at least. Similar to this study, Hu et al. (2012)Citation75 reported that the PGK gene of maize (Zea mays L.) seedlings was increased in dim-light conditions. Also, ginseng (Panax ginseng L.) plants grown under shaded conditions, similar to the light intensity of 4% FS (30 µmol m−2 s−1) in this study, exhibited upregulation of photosynthesis-related genes, including PGK and GAPDH.Citation76 On the contrary, the highest light intensity of 75% FS treatment revealed downregulation of gene expressions despite the high enzyme activity of Rubisco at the tenth week as shown in . According to Uematsu et al.,Citation38 saturated photosynthetic response under excessive light conditions during long periods limits regeneration of RuBP and phosphorylation and photoinhibition. Downregulation of photosynthetic genes is related to increased carbohydrate accumulation, reducing photosynthetic enzyme activities, including Rubisco, GAPDH, and FBPase.Citation37,Citation77 Also, the downregulation in Rubisco gene due to light stress has been reported by Escoubas et al.Citation78 and Erickson et al.Citation79
Moreover, plant acclimation to light stress for long periods induces changes in photosynthetic gene expressions, inhibiting photosynthetic proteins and protein synthesis.Citation80,Citation81 It has been demonstrated that plants exposed to high light intensity, like 75% FS at the tenth week, exhibited photoinhibition affecting the loss of O2-evolving activity and facilitating the synthesis of D1 polypeptide.Citation82 On the other hand, it is possible that photosynthetic genes of Rubisco, GAPDH, and FBPase provided the leaves with essential storage proteins, sucrose, and starch under low irradiance.Citation38,Citation83 This study identified spots indicating different proteins from the 2-DE gel tests (Supplemental Figure S1). As a result, higher Rubisco proteome levels in the seedlings grown under high and moderate light intensities (75% and 25% FS) enhanced Rubisco enzyme activity. However, Rubisco protein was not found in the low light of 4% FS. This may be due to the shade stress leads to oxidative damage and D1 protein degradation by generating active oxygen species,Citation57,Citation84 decreasing Rubisco enzyme activity. Meanwhile, in the leaves of P. indicus seedlings grown in 4% FS, ferric leghemoglobin reductase (FLBR) and glycine dehydrogenase were up-regulated compared to other treatments. FLBR is an essential protein inhabiting the root nodules of legume crops that binds to O2 for maintaining O2 concentration and improving N2-fixing bacteroids in a microaerobic environment.Citation85–87 FLBR allows O2 respiration in the root nodules, protecting them from disease, and is a defense against biotic stresses.Citation88,Citation89 Although the leaves in 4% FS had shaded stress in the absence of the Rubisco proteome, the roots of P. indicus seedlings in the low light may have stress tolerance by increasing FLBR. Glycine dehydrogenase is usually decreased under abiotic stresses, including chilling (cold) and heavy metal exposure, which results in low photorespiration efficiency in the leaves.Citation90 Contrary to this, Lee et al.Citation91 reported that glycine dehydrogenase was up-regulated in the roots of rice (Oryza sativa L.) seedlings under abiotic stress. Glycine dehydrogenase may regulate electron transport to prevent photoinhibition under a stressful environment.Citation92 A higher level of glycine dehydrogenase could generate higher energy for coping with abiotic stress.Citation91 In this study, the upregulation of glycine dehydrogenase in the leaves grown in dark conditions of 4% FS may positively affect the generation of shading stress tolerance.
Conclusion
In tropical forests where sunlight is blocked due to canopy and climate change, securing shade tolerance is the most essential factor for the survival of tree seedlings. Our findings obtained from this mesocosm experiment demonstrated that P. indicus seedlings were capable to tolerate and acclimate to the low light environment. Albeit the adverse effect of shading treatment on biomass production was clear, the Narra seedlings showed an acclimation ability to shading stress during the 20-week experiment, via generating specific responses that modulate physiological (e.g., photosynthesis, pigments, Rubisco enzyme activity, and biomass), morphological (e.g., specific leaf area), and molecular (e.g., photosynthetic genes, such as Rubisco, GAPDH, EBPase, and PGK) characteristics. Although the acclimatory responses of Narra seedlings varied considerably with light intensity and shading duration, it seems that this species was able to maintain their viability under harsh light conditions through an enhanced tolerance mechanism to the abiotic stress. These results in the present study will improve our understanding of the ecophysiological response traits of Narra seedlings under light-deficient conditions and provide a better strategy for efficiently growing seedlings of this species in tropical rainforests.
Abbreviations
Ac | = | the photosynthetic rate limited by Rubisco activity |
Aj | = | the photosynthetic rate limited by RuBP regeneration |
Ca | = | ambient CO2 concentration |
Ci | = | intercellular CO2 concentration |
Chl | = | chlorophyll |
Car | = | carotenoid |
DW | = | dry weight |
FBPase | = | fructose 1,6-bisphosphate aldolase |
FLBR | = | ferric leghemoglobin reductase |
FS | = | full sunlight |
FW | = | fresh weight |
GAPDH | = | glyceraldehyde 3-phosphate dehydrogenase |
Gs | = | stomatal conductance |
Jmax | = | the maximum electron transport rate |
Kc | = | Rubisco Michaelis-Menten constant for CO2 |
Ko | = | Rubisco Michaelis-Menten constant for O2 |
LA | = | leaf area |
LMR | = | leaf mass ratio |
PN | = | the net photosynthetic rate |
PNmax | = | tthe maximum rate of light-saturated photosynthesis |
PPFD | = | photosynthetic photon flux density |
PGA | = | D-phosphoglyceric acid |
PGK | = | 3-Phosphoglycerate kinase |
Rd | = | day respiration |
Rubisco | = | ribulose 1,5-bisphosphae carboxylase/oxygenase |
RMR | = | root mass ratio |
RT-PCR | = | reverse transcription polymerase chain reaction |
SLA | = | specific leaf area |
SMR | = | shoot mass ratio |
Γ* | = | the CO2 compensation point |
Tr | = | transpiration rate |
VCmax | = | the maximum rate of Rubisco carboxylation |
WUE | = | water use efficiency |
Author contributions
Conceptualization: K-AL, SYW. Formal analysis and investigation: K-AL, VK, Y-NK. Writing – original draft preparation: K-AL, VK. Writing – review and editing: Y-NK, YBL. Supervision: SYW. All authors read and approved the final manuscript.
Supplemental Material
Download Rich Text Format File (377.4 KB)Disclosure statement
No potential conflict of interest was reported by the authors.
Data availability statement
The original datasets presented in the study are available from the corresponding authors.
Supplementary material
Supplemental data for this article can be accessed online at https://doi.org/10.1080/15592324.2023.2245625
Additional information
Funding
References
- Grote R, Gessler A, Hommel R, Poschenrieder W, Priesack E. Importance of tree height and social position for drought-related stress on tree growth and mortality. Trees. 2016;30(5):1467–12. doi:10.1007/s00468-016-1446-x.
- Hiromi T, Ichie T, Kenzo T, Ninomiya I. Interspecific variation in leaf water use associated with drought tolerance in four emergent dipterocarp species of a tropical rain forest in Borneo. J For Res. 2012;17(4):369–377. doi:10.1007/s10310-011-0303-4.
- Kenzo T, Ichie T, Watanabe Y, Yoneda R, Ninomiya I, Koike T. Changes in photosynthesis and leaf characteristics with tree height in five dipterocarp species in a tropical rain forest. Tree Physiol. 2006;26(7):865–873. doi:10.1093/treephys/26.7.865.
- Leakey A, Press M, Scholes J. High‐temperature inhibition of photosynthesis is greater under sunflecks than uniform irradiance in a tropical rain forest tree seedling. Plant, Cell & Environ. 2003;26(10):1681–1690. doi:10.1046/j.1365-3040.2003.01086.x.
- Umesh MR, Angadi S, Begna S, Gowda P, Prasad PVV. Shade tolerance response of legumes in terms of biomass accumulation, leaf photosynthesis, and chlorophyll pigment under reduced sunlight. Crop Sci. 2023;63(1):278–292. doi:10.1002/csc2.20851.
- Azevedo GFC, Marenco RA. Growth and physiological changes in saplings of Minquartia guianensis and Swietenia macrophylla during acclimation to full sunlight. Photosynthetica. 2012;50(1):86–94. doi:10.1007/s11099-012-0001-2.
- Foyer CH, Neukermans J, Queval G, Noctor G, Harbinson J. Photosynthetic control of electron transport and the regulation of gene expression. J Exp Bot. 2012;63(4):1637–1661. doi:10.1093/jxb/ers013.
- Mathur S, Jain L, Jajoo A. Photosynthetic efficiency in sun and shade plants. Photosynt. 2018;56(SPECIAL ISSUE):354–365. doi:10.1007/s11099-018-0767-y.
- Vialet-Chabrand SR, Matthews JS, Simkin A, Raines CA, Lawson T. Importance of fluctuations in light on plant photosynthetic acclimation. Plant Physiol. 2017;173(4):2163–2179. doi:10.1104/pp.16.01767.
- Mi G, Liu L, Zhang Z, Ren H. Changes in photosynthesis and activities of enzymes involved in carbon metabolism during exposure to low light in cucumber (Cucumis sativus) seedlings. Afr J Biotechnol. 2012;11(34):8537–8545. doi:10.5897/AJB11.1632.
- Zivcak M, Brestic M, Kalaji HM, Govindjee. Photosynthetic responses of sun- and shade-grown barley leaves to high light: is the lower PSII connectivity in shade leaves associated with protection against excess of light? Photosynth Res. 2014;119(3):339–354. doi:10.1007/s11120-014-9969-8.
- Ding Z, Zhang Y, Xiao Y, Liu F, Wang M, Zhu X, Liu P, Sun Q, Wang W, Peng M. Transcriptome response of cassava leaves under natural shade. Sci Rep. 2016;6(1):31673. doi:10.1038/srep31673.
- Huang D, Wu L, Chen JR, Dong L. Morphological plasticity, photosynthesis and chlorophyll fluorescence of Athyrium pachyphlebium at different shade levels. Photosynthetica. 2011;49(4):611–618. doi:10.1007/s11099-011-0076-1.
- Liu Y, Dawson W, Prati D, Haeuser E, Feng Y, van Kleunen M. Does greater specific leaf area plasticity help plants to maintain a high performance when shaded? Ann Bot. 2016;118(7):1329–1336. doi:10.1093/aob/mcw180.
- Wang Y, Lu Y, Chang Z, Wang S, Ding Y, Ding C. Transcriptomic analysis of field-grown rice (Oryza sativa L.) reveals responses to shade stress in reproductive stage. Plant Growth Regul. 2018;84(3):583–592. doi:10.1007/s10725-017-0363-3.
- Cifuentes L, Moreno F, Mencuccini M. Trait coordination at leaf level explains the resistance to excess light stress in shade-tolerant tropical tree species. Tree Physiol. 2022;42(7):1325–1336. doi:10.1093/treephys/tpac014.
- Bar E, Rise M, Vishkautsan M, Arad SM. Pigment and structural changes in Chlorella zofingiensis upon light and nitrogen stress. J Plant Physiol. 1995;146(4):527–534. doi:10.1016/S0176-1617(11)82019-5.
- Gazal RM, Blanche CA, Carandang WM. Root growth potential and seedling morphological attributes of narra (Pterocarpus indicus willd.) transplants. Forest Ecol Manag. 2004;195(1–2):259–266. doi:10.1016/j.foreco.2004.03.023.
- Paquit JC, Pampolina NM, Tiburan CL Jr, Manalo MMQ. Maxent modeling of the habitat distribution of the critically endangered Pterocarpus indicus willd. forma indicus inmindanao, Philippines. Int J Network Nat Sci. 2017;10:112–122.
- Vanclay JK, Gregorio NO, Herbohn JL. Competition in a mixed-species planting with four contrasting tree species. Small-Scale For. 2022;1–19. doi:10.1007/s11842-022-09532-w.
- Aguilos R, Marquez C, Adornado H, Aguilos M. Domesticating commercially important native tree species in the Philippines: early growth performance level. Forests. 2020;11(8):885. doi:10.3390/f11080885.
- Kandasamy N, Kaliappan K, Palanisamy T. Upcycling sawdust into colorant: ecofriendly natural dyeing of fabrics with ultrasound assisted dye extract of Pterocarpus indicus willd. Ind Crops Prod. 2021;171:113969. doi:10.1016/j.indcrop.2021.113969.
- Meng LZ, Martin K, Weigel A, Yang XD, Lortie CJ. Tree diversity mediates the distribution of longhorn beetles (Coleoptera: Cerambycidae) in a changing tropical landscape (Southern Yunnan, SW China). PLoS One. 2013;8(9):e75481. doi:10.1371/journal.pone.0075481.
- Sterck FJ, Duursma RA, Pearcy RW, Valladares F, Cieslak M, Weemstra M, Turnbull M. Plasticity influencing the light compensation point offsets the specialization for light niches across shrub species in a tropical forest understorey. J Ecol. 2013;101(4):971–980. doi:10.1111/1365-2745.12076.
- Galmés J, Medrano H, Flexas J. Photosynthetic limitations in response to water stress and recovery in Mediterranean plants with different growth forms. New Phytol. 2007;175(1):81–93. doi:10.1111/j.1469-8137.2007.02087.x.
- Arnon DI. Copper enzymes in isolated chloroplasts. Polyphenoloxidase in Beta vulgaris. Plant Physiol. 1949;24(1):1. doi:10.1104/pp.24.1.1.
- Baek S, Woo S. Physiological and biochemical responses of two tree species in urban areas to different air pollution levels. Photosynt. 2010;48(1):23–29. doi:10.1007/s11099-010-0005-8.
- Farquhar GD, von Caemmerer S, Berry JA. A biochemical model of photosynthetic CO2 assimilation in leaves of C3 species. Planta. 1980;149(1):78–90. doi:10.1007/BF00386231.
- Bernacchi CJ, Singsaas EL, Pimentel C, Portis Jr AR Jr, Long SP. Improved temperature response functions for models of Rubisco‐limited photosynthesis. Plant, Cell & Environ. 2001;24(2):253–259. doi:10.1111/j.1365-3040.2001.00668.x.
- Onoda Y, Hikosaka K, Hirose T. Seasonal change in the balance between capacities of RuBP carboxylation and RuBP regeneration affects CO2 response of photosynthesis in Polygonum cuspidatum. J Exp Bot. 2005;56(412):755–763. doi:10.1093/jxb/eri052.
- Kasai M. Regulation of leaf photosynthetic rate correlating with leaf carbohydrate status and activation state of Rubisco under a variety of photosynthetic source/sink balances. Physiol Plant. 2008;134(1):216–226. doi:10.1111/j.1399-3054.2008.01105.x.
- Lu KX, Cao BH, Feng XP, He Y, Jiang DA. Photosynthetic response of salt-tolerant and sensitive soybean varieties. Photosynthetica. 2009;47(3):381–387. doi:10.1007/s11099-009-0059-7.
- Kim SH, Hamada T. Rapid and reliable method of extracting DNA and RNA from sweetpotato, Ipomoea batatas (L). Lam. Biotechnol Lett. 2005;27(23–24):1841–1845. doi:10.1007/s10529-005-3891-2.
- Kim ST, Kim SG, Kang YH, Wang Y, Kim JY, Yi N, Kim JK, Rakwal R, Koh HJ, Kang KY. Proteomics analysis of rice lesion mimic mutant (spl1) reveals tightly localized probenazole-induced protein (PBZ1) in cells undergoing programmed cell death. J Proteome Res. 2008;7(4):1750–1760. doi:10.1021/pr700878t.
- Ishii H, Ohsugi Y. Light acclimation potential and carry-over effects vary among three evergreen tree species with contrasting patterns of leaf emergence and maturation. Tree Physiol. 2011;31(8):819–830. doi:10.1093/treephys/tpr079.
- Coste S, Roggy JC, Schimann H, Epron D, Dreyer E. A cost–benefit analysis of acclimation to low irradiance in tropical rainforest tree seedlings: leaf life span and payback time for leaf deployment. J Exp Bot. 2011;62(11):3941–3955. doi:10.1093/jxb/err092.
- Krapp A, Hofmann B, Schäfer C, Stitt M. Regulation of the expression of rbcS and other photosynthetic genes by carbohydrates: a mechanism for the ‘sink regulation’of photosynthesis? Plant J. 1993;3(6):817–828. doi:10.1111/j.1365-313X.1993.00817.x.
- Uematsu K, Suzuki N, Iwamae T, Inui M, Yukawa H. Increased fructose 1,6-bisphosphate aldolase in plastids enhances growth and photosynthesis of tobacco plants. J Exp Bot. 2012;63(8):3001–3009. doi:10.1093/jxb/ers004.
- Boardman NK. Comparative photosynthesis of sun and shade plants. Ann Rev Plant Physiol. 1977;28(1):355–377. doi:10.1146/annurev.pp.28.060177.002035.
- Krause GH, Winter K, Matsubara S, Krause B, Jahns P, Virgo A, Aranda J, García M. Photosynthesis, photoprotection, and growth of shade-tolerant tropical tree seedlings under full sunlight. Photosynth Res. 2012;113(1–3):273–285. doi:10.1007/s11120-012-9731-z.
- Stokes VJ, Morecroft MD, Morison JIL. Comparison of leaf water use efficiency of oak and sycamore in the canopy over two growing seasons. Trees. 2010;24(2):297–306. doi:10.1007/s00468-009-0399-8.
- Dai Y, Shen Z, Liu Y, Wang L, Hannaway D, Lu H. Effects of shade treatments on the photosynthetic capacity, chlorophyll fluorescence, and chlorophyll content of Tetrastigma hemsleyanum diels et Gilg. Environ Exp Bot. 2009;65(2–3):177–182. doi:10.1016/j.envexpbot.2008.12.008.
- Barros FDV, Goulart MF, Sá Telles SB, Lovato MB, Valladares F, Lemos‐Filho JD. Phenotypic plasticity to light of two congeneric trees from contrasting habitats: Brazilian Atlantic forest versus cerrado (savanna). Plant Biol. 2012;14(1):208–215. doi:10.1111/j.1438-8677.2011.00474.x.
- Martin CE, Hsu RCC, Lin TC. Sun/Shade adaptations of the photosynthetic apparatus of Hoya carnosa, an epiphytic CAM vine, in a subtropical rain forest in northeastern Taiwan. Acta Physiol Plant. 2010;32(3):575–581. doi:10.1007/s11738-009-0434-9.
- Lichtenthaler HK, A A, Marek MV, Kalina J, Urban O. Differences in pigment composition, photosynthetic rates and chlorophyll fluorescence images of sun and shade leaves of four tree species. Plant Physiol Biochem. 2007;45(8):577–588. doi:10.1016/j.plaphy.2007.04.006.
- Nyongesah MJ, Wang Q, Li P. Effectiveness of photochemical reflectance index to trace vertical and seasonal chlorophyll a/b ratio in Haloxylon ammodendron. Acta Physiol Plant. 2015;37(2):1–11. doi:10.1007/s11738-014-1747-x.
- Favaretto VF, Martinez CA, Soriani HH, Furriel RP. Differential responses of antioxidant enzymes in pioneer and late-successional tropical tree species grown under sun and shade conditions. Environ Exp Bot. 2011;70(1):20–28. doi:10.1016/j.envexpbot.2010.06.003.
- Valladares F, Martinez-Ferri E, Balaguer L, Perez-Corona E, Manrique E. Low leaf-level response to light and nutrients in Mediterranean evergreen oaks: a conservative resource-use strategy? New Phytol. 2000;148(1):79–91. doi:10.1046/j.1469-8137.2000.00737.x.
- Beneragama CK, Goto K. Chlorophyll a: b ratio increases under low-light in “shade-tolerant. Euglena Gracilis Trop Agric Res. 2010;22(1):12–25. doi:10.4038/tar.v22i1.2666.
- Casierra-Posada F, Ávila-León OF. Shade tolerance of marigold plants (calendula officinalis). Rev UDCA Act Div Cien. 2015;18(1):119–126. doi:10.31910/rudca.v18.n1.2015.460.
- Sarijeva G, Knapp M, Lichtenthaler HK. Differences in photosynthetic activity, chlorophyll and carotenoid levels, and in chlorophyll fluorescence parameters in green sun and shade leaves of Ginkgo and Fagus. J Plant Physiol. 2007;164(7):950–955. doi:10.1016/j.jplph.2006.09.002.
- Gomes-Laranjo J, Coutinho JP, Galhano V, Ferreira-Cardoso JV. Differences in photosynthetic apparatus of leaves from different sides of the chestnut canopy. Photosynt. 2008;46(1):63–72. doi:10.1007/s11099-008-0012-1.
- Knapp AK, Gilliam FS. Response of Andropogon gerardii (poaceae) to fire‐induced high vs. low irradiance environments in tallgrass prairie: leaf structure and photosynthetic pigments. Am J Bot. 1985;72(11):1668–1671. doi:10.1002/j.1537-2197.1985.tb08435.x.
- Stinziano JR, Way DA. Autumn photosynthetic decline and growth cessation in seedlings of white spruce are decoupled under warming and photoperiod manipulations. Plant, Cell & Environ. 2017;40(8):1296–1316. doi:10.1111/pce.12917.
- Rzigui T, Cherif J, Zorrig W, Khaldi A, Nasr Z. Adjustment of photosynthetic carbon assimilation to higher growth irradiance in three-year-old seedlings of two Tunisian provenances of cork oak (Quercus suber L.). IForest. 2017;10(3):618–624. doi:10.3832/ifor2105-010.
- Tian Y, Yuan H, Xie J, Zheng Y. Shade tolerance and suitability of tree species for planting in rubber plantations. J For Sci. 2016;78(1):11–18. doi:10.2989/20702620.2015.1089093.
- Fox AR, Barberini ML, Ploschuk EL, Muschietti JP, Mazzella MA. A proteome map of a quadruple photoreceptor mutant sustains its severe photosynthetic deficient phenotype. J Plant Physiol. 2015;185:13–23. doi:10.1016/j.jplph.2015.07.004.
- Touchette BW, Burkholder JM. Overview of the physiological ecology of carbon metabolism in seagrasses. J Exp Mar Bio Ecol. 2000;250(1):169–205. doi:10.1016/S0022-0981(00)00196-9.
- Sage RF, McKown AD. Is C4 photosynthesis less phenotypically plastic than C3 photosynthesis? J Exp Bot. 2006;57(2):303–317. doi:10.1093/jxb/erj040.
- Chen LS, Cheng L. The sun-exposed peel of apple fruit has a higher photosynthetic capacity than the shaded peel. Funct Plant Biol. 2007;34(11):1038–1048. doi:10.1071/FP07111.
- Hidema J, Makino A, Mae T, Ojima K. Photosynthetic characteristics of rice leaves aged under different irradiances from full expansion through senescence. Plant Physiol. 1991;97(4):1287–1293. doi:10.1104/pp.97.4.1287.
- Seemann JR. Light adaptation/acclimation of photosynthesis and the regulation of ribulose-1,5-bisphosphate carboxylase activity in sun and shade plants. Plant Physiol. 1989;91(1):379–386. doi:10.1104/pp.91.1.379.
- Niinemets Ü. Responses of forest trees to single and multiple environmental stresses from seedlings to mature plants: past stress history, stress interactions, tolerance and acclimation. Forest Ecol Manag. 2010;260(10):1623–1639. doi:10.1016/j.foreco.2010.07.054.
- Kelly J, Jose S, Nichols JD, Bristow M. Growth and physiological response of six Australian rainforest tree species to a light gradient. Forest Ecol Manag. 2009;257(1):287–293. doi:10.1016/j.foreco.2008.09.008.
- Poorter L. Growth responses of 15 rainforest tree species to a light gradient: the relative importance of morphological and physiological traits. Funct Ecology. 1999;13(3):396–410. doi:10.1046/j.1365-2435.1999.00332.x.
- Salgado‐Luarte C, Gianoli E. Herbivory may modify functional responses to shade in seedlings of a light‐demanding tree species. Funct Ecol. 2011;25(3):492–499. doi:10.1111/j.1365-2435.2010.01763.x.
- Zhao D, Hao Z, Tao J. Effects of shade on plant growth and flower quality in the herbaceous peony (Paeonia lactiflora pall.). Plant Physiol Biochem. 2012;61:187–196. doi:10.1016/j.plaphy.2012.10.005.
- Cregg BM, Teskey RO, Dougherty PM. Effect of shade stress on growth, morphology, and carbon dynamics of loblolly pine branches. Trees. 1993;7(4):208–213. doi:10.1007/BF00202075.
- Kenzo T, Inoue Y, Yoshimura M, Yamashita M, Tanaka-Oda A, Ichie T. Height-related changes in leaf photosynthetic traits in diverse Bornean tropical rain forest trees. Oecologia. 2015;177(1):191–202. doi:10.1007/s00442-014-3126-0.
- Senevirathna AMWK, Stirling CM, Rodrigo VHL. Growth, photosynthetic performance and shade adaptation of rubber (Hevea brasiliensis) grown in natural shade. Tree Physiol. 2003;23(10):705–712. doi:10.1093/treephys/23.10.705.
- Gyimah R, Nakao T. Early growth and photosynthetic responses to light in seedlings of three tropical species differing in successional strategies. New For. 2007;33(3):217–236. doi:10.1007/s11056-006-9028-1.
- Retuerto R, Woodward F. The influences of increased CO2 and water supply on growth, biomass allocation and water use efficiency of Sinapis alba L. grown under different wind speeds. Oecologia. 1993;94(3):415–427. doi:10.1007/BF00317118.
- Gratani L. Plant phenotypic plasticity in response to environmental factors. Adv Bot. 2014;2014:208747. doi:10.1155/2014/208747.
- Umaña MN, Swenson NG, Marchand P, Cao M, Lin L, Zhang C. Relating leaf traits to seedling performance in a tropical forest: building a hierarchical functional framework. Ecology. 2021;102(7):e03385. doi:10.1002/ecy.3385.
- Hu X, Wu X, Li C, Lu M, Liu T, Wang Y, Wang W, Schönbach C. Abscisic acid refines the synthesis of chloroplast proteins in maize (Zea mays) in response to drought and light. PLoS One. 2012;7(11):e49500. doi:10.1371/journal.pone.0049500.
- Zhang YX, Niu YQ, Wang XF, Wang ZH, Wang ML, Yang J, Wang YG, Zhang WJ, Song ZP, Li LF. Phenotypic and transcriptomic responses of the shade-grown species Panax ginseng to variable light conditions. Ann Bot. 2022;130(5):749–762. doi:10.1093/aob/mcac105.
- Kappachery S, Sasi S, Alyammahi O, Alyassi A, Venkatesh J, Gururani MA. Overexpression of cytoplasmic solanum tuberosum glyceraldehyde 3-phosphate dehydrogenase (GAPDH) gene improves PSII efficiency and alleviates salinity stress in Arabidopsis. J Plant Interact. 2021;16(1):398–410. doi:10.1080/17429145.2021.1962420.
- Escoubas JM, Lomas M, LaRoche J, Falkowski PG. Light intensity regulation of cab gene transcription is signaled by the redox state of the plastoquinone pool. Proc Natl Acad Sci U S A. 1995;92(22):10237–10241. doi:10.1073/pnas.92.22.10237.
- Erickson E, Wakao S, Niyogi KK. Light stress and photoprotection in Chlamydomonas reinhardtii. Plant J. 2015;82(3):449–465. doi:10.1111/tpj.12825.
- Mikko T, Mirva P, Sari S, Paula M, Julia V, Alexander V, Yagut A, Eva-Mari A. State transitions revisited–a buffering system for dynamic low light acclimation of Arabidopsis. Plant Mol Biol. 2006;2006(4):779–793. doi:10.1007/s11103-006-9044-8.
- Walters RG. Towards an understanding of photosynthetic acclimation. J Exp Bot. 2005;56(411):435–447. doi:10.1093/jxb/eri060.
- Viola S, Roseby W, Santabarbara S, Nürnberg D, Assunção R, Dau H, Sellés J, Boussac A, Fantuzzi A, Rutherford AW. Impact of energy limitations on function and resilience in long-wavelength photosystem II. Elife. 2022;11:e79890. doi:10.7554/eLife.79890.
- Li T, Liu LN, Jiang CD, Liu YJ, Shi L. Effects of mutual shading on the regulation of photosynthesis in field-grown sorghum. J Photochem Photobiol. 2014;137:31–38. doi:10.1016/j.jphotobiol.2014.04.022.
- Keren N, Berg A, Van Kan PJ, Levanon H, Ohad I. Mechanism of photosystem II photoinactivation and D1 protein degradation at low light: the role of back electron flow. Proc Natl Acad Sci. 1997;94(4):1579–1584. doi:10.1073/pnas.94.4.1579.
- Becana M, Salin ML, Ji L, Klucas RV. Flavin-mediated reduction of ferric leghemoglobin from soybean nodules. Planta. 1991;183(4):575–583. doi:10.1007/BF00194279.
- Brear EM, Day DA, Smith PM. Iron: an essential micronutrient for the legume-rhizobium symbiosis. Front Plant Sci. 2013;4:359. doi:10.3389/fpls.2013.00359.
- Ji L, Wood S, Becana M, Klucas RV. Purification and characterization of soybean root nodule ferric leghemoglobin reductase. Plant Physiol. 1991;96(1):32–37. doi:10.1104/pp.96.1.32.
- Ma J, Lv C, Xu M, Hao P, Wang Y, Shen W, Gao Z, Chen G, Lv C. Analysis of chlorophyll a fluorescence and proteomic differences of rice leaves in response to photooxidation. Acta Physiol Plant. 2017;39(2):1–14. doi:10.1007/s11738-016-2342-0.
- Sangwan I, O’Brian MR. Expression of the soybean (Glycine max) glutamate 1-semialdehyde aminotransferase gene in symbiotic root nodules. Plant Physiol. 1993;102(3):829–834. doi:10.1104/pp.102.3.829.
- Hossain Z, Makino T, Komatsu S. Proteomic study of β-aminobutyric acid-mediated cadmium stress alleviation in soybean. J Proteom. 2012;75(13):4151–4164. doi:10.1016/j.jprot.2012.05.037.
- Lee DG, Ahsan N, Lee SH, Lee JJ, Bahk JD, Kang KY, Lee BH. Chilling stress-induced proteomic changes in rice roots. J Plant Physiol. 2009;166(1):1–11. doi:10.1016/j.jplph.2008.02.001.
- Yan SP, Zhang QY, Tang ZC, Su WA, Sun WN. Comparative proteomic analysis provides new insights into chilling stress responses in rice. Molecular & Cellular Proteomics: MCP. 2006;5(3):484–496. doi:10.1074/mcp.M500251-MCP200.