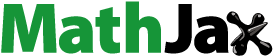
ABSTRACT
Blueberries confront substantial challenges from climate change, such as rising temperatures and extreme heat, necessitating urgent solutions to ensure productivity. We hypothesized that ericoid mycorrhizal fungi (ErM) and plant growth-promoting bacteria (PGPB) would establish symbiotic relationships and increase heat stress tolerance in blueberries. A growth chamber study was designed with low (25/20°C) and high temperature (35/30°C) conditions with micropropagated blueberry plantlets inoculated with ErM, PGPB, and both. Gas exchange and chlorophyll fluorescence properties of the leaves were monitored throughout the growth. At harvest, biochemical assays and biomass analysis were performed to evaluate potential oxidative stress induced by elevated temperatures. ErM application boosted root biomass under 25/20°C conditions but did not impact photosynthetic efficiency. In contrast, PGPB demonstrated a dual role: enhancing photosynthetic capacity and reducing stomatal conductance notably under 35/30°C conditions. Moreover, PGPB showcased conflicting effects, reducing oxidative damage under 25/20°C conditions while intensifying it during 47°C heat shock. A significant highlight lies in the opposing effects of ErM and PGPB on root growth and stomatal conductance, signifying their reciprocal influence on blueberry plant behavior, which may lead to increased water uptake or reduced water use. Understanding these complex interactions holds promise for refining sustainable strategies to overcome climate challenges.
Introduction
Blueberries (Vaccinium corymbosum) stand as high-valued fruiting bushes within the Ericaceae family. Their esteemed position in the market is owed to many factors, primarily their exceptional nutritional profile. Blueberries are rich in antioxidants and surpass many other fruits in their potency.Citation1 However, this ascendancy in the agricultural domain encounters significant impediments stemming from the increasingly unpredictable climate, characterized by rising temperatures and erratic weather patterns. These environmental shifts pose substantial challenges to the sustained productivity of blueberry plantations, raising concerns about the future of this industry.
Among the various threats crops face in climate change, extreme heat, and drought stress emerge as particularly detrimental factors. Studies have illustrated the adverse effects of heightened temperaturesCitation2 and prolonged droughtCitation3 on numerous crops, including blueberries. The repercussions of these stressors transcend mere yield reduction, encompassing compromised plant health and vitality. Addressing these challenges becomes imperative for ensuring the resilience and viability of agricultural practices, especially concerning high-value crops like blueberries.
In this landscape of agricultural adversity, exploring novel strategies becomes pivotal. One promising avenue lies in leveraging the potential of beneficial microorganisms, notably mycorrhizae and plant growth-promoting bacteria (PGPB). This research focuses on a cultivar from the southern highbush blueberry group renowned for its remarkable resilience to heat stress. The cultivar under scrutiny, “Jewel”,Citation4 boasts commendable berry production and exhibits low chilling requirements, rendering it adaptable to cultivation in warmer climates such as subtropical and cooler tropical regions.Citation5 An additional advantage lies in the expiration of the patent for this cultivar, making it accessible for public utilization.Citation6
The absence of root hairs in blueberries, distinctive within the Ericaceae family, prompts an evolutionary adaptation. These plants engage in a symbiotic relationship with fungi establishing ericoid mycorrhizae (ErM) to compensate for the lack of root hairs, thereby augmenting root surface area.Citation7 The ErM’s symbiotic association with the Ericaceae family dates back to 140 million years ago, ascertaining the importance of symbiosis in the host plants’ acclimation and adaptation to environmental stresses.Citation8 The manifestation of this association is observable after staining roots by the occurrence of coils within the root cells,Citation9 with a concentration of mycelium in nascent roots that gradually diminishes toward the plant’s base.Citation10
Previous research underscores the pivotal roles of beneficial symbiotic microorganisms in bolstering plant growth and resilience to stressors.Citation11 Inoculation with ErM has exhibited a capacity to enhance root biomassCitation12,Citation13 and facilitate nutrient uptakeCitation14 in blueberry plants. Similarly, the inoculation of PGPB has shown a spectrum of effects, ranging from neutral outcomesCitation15 to positive impacts. These encompass heightened growth,Citation16 improved fruit quality,Citation17 and augmented defense mechanisms against pathogens.Citation18 Although numerous studies have explored the benefits of symbiotic microbial inoculation in enhancing resilience and yield in various crops,Citation19 the specific implications and potentials for blueberries remain underexplored.
This research delves into the intricate interplay between beneficial symbiotic microorganisms and their impact on blueberry plants. Our focus is to unravel the dynamic interactions between ErM and PGPB when concurrently applied to these plants. At the heart of this exploration lie the essential components: blueberries, ErM, PGPB, and the intricacies of the host plant’s behavior and resilience to heat stress within these complex relationships. Thus, our research questions were: 1) Can ErM and PGPB co-exist in their blueberry host plant? 2) Can both of them establish a symbiotic relationship with the host? and 3) Can the symbiotic relationships increase the heat stress tolerance of the host and how? Our initial hypotheses centered around the potential for both ErM and PGPB to foster growth, development, and heat stress tolerance in blueberry plants. Moreover, we anticipated synergistic effects from the dual symbiotic associations with both ErM and PGPB, especially in elevated temperature conditions. Throughout this investigation, our aim was to meticulously document the behavioral changes in blueberry hosts influenced by these symbiotic interactions, dissecting their impact on biochemical, physiological, and morphological levels.
Materials and methods
Micropropagation of plant materials – blueberry cultivation
Mature “Jewel” blueberries cultivated in a greenhouse served as stock plants. On October 7, 2022, single-shoot explants were obtained and subjected to a rigorous sterilization process. After a two-hour wash under running water, the explants were sterilized with 1% bleach for 20 minutes, then rinsed with 70% alcohol twice for 2 minutes each. Subsequently, the explants were placed in a plant multiplication medium (M724, Sigma-Aldrich, St. Louis, MO, United States) for 4 weeks to regenerate sterile shoots. The sterile shoots were then multiplied in Woody Plant Media (M6744, Sigma-Aldrich)Citation20,Citation21 supplemented with 2 mg L−1 of Zeatin (Z0164, Sigma-Aldrich) to promote multiplication efficiency.Citation22–24 We re-cultured every 4–5 weeks to ensure a sufficient amount of plant materials with enhanced sterility for further assays and analyses.
Once sufficient quantities were obtained (after four multiplications), plantlets were transferred to a mixture of low-pH peat (King Root Brand, Dayi Agritech Co., Changzhi, Pingtung, Taiwan) and perlite in a 9:1 ratio.Citation25 Peat underwent sterilization via autoclaving to ensure optimal sterility. The conditions provided were within a sealed container with high humidity and a light intensity of 100 µmol m−2 s−1 photosynthetic photon flux density (PPFD). The rooting hormone, NAA (N0640, Sigma-Aldrich) in 50% ethanol,Citation26,Citation27 was applied at a concentration of 1 mg L−1 for 10 secondsCitation25 to promote robust rooting.
Preparation and inoculation of microbes – ErM and PGPB
On August 5, 2023, inoculation of ErM and PGPG were performed successfully on micropropagated plants.
The ErM strains, Oidiodendron maius isolates, have shown capabilities in establishing symbiotic relationships with ericaceous plants, originally collected from Formosan rhododendron (Rhododendron formosanum Hemsl.).Citation28,Citation29 Oidiodendron maius RhYM3 fungal ErM strainCitation30 was maintained on 2% malt extract at 25°C and deposited in the Tree Mycorrhiza Laboratory of National Chiayi University. After 30-day incubation of Oidiodendron maius RhYM3, the inoculum prepared mycelial slurry of maintained in sterile liquid culture on 2% malt extract medium by fragmenting the mycelium in a blender for 30 seconds The inoculation method was done by pouring 10 mL of the inoculum in each blueberry plantlet.Citation31,Citation32
The PGPB strain, Klebsiella sp., was obtained from a green onion plantation and purified in the Vegetable Stress Physiology Laboratory of National Taiwan University under aseptic conditions. This strain, Klebsiella sp. GRB10, exhibited resilience to high temperatures and demonstrated efficacy in mitigating heat stress in lettuce.Citation33 The bacterial strain was cultured on LB agar until colonies formed, then grown in liquid LB broth until reaching an OD600 value of 1.0 (approximately 1 × 10Citation8 colony forming units). The resultant bacterial solution served as a direct inoculum, where rooted plantlets were soaked for 5 minutes.
For combined treatments involving both beneficial bacteria and mycorrhizae, equal parts of the respective liquid solutions were mixed beforehand. The plant roots were submerged for 4 minutes in this combined solution. Following inoculation, all plants were transplanted to sterile media and larger pots for further growth and assessment.
Experimental settings in growth chambers
All plants were cultivated in 6 cm diameter seedling trays and received regular watering. Initially, rooted micropropagated plantlets were grown at a consistent ambient temperature of 25/25°C. Following inoculation, the plants were relocated to a growth chamber set at 25°C with a 12-hour light cycle and an illumination intensity of 600 µmol m−2 s−1 PPFD. After one month, half of the plants were transferred to a growth chamber with a temperature cycle of 35/30°C, while the remaining plants continued at 25/20°C.
To summarize, the experimental design post-inoculation involved a factorial arrangement of two primary factors: growing temperatures (TEMP) and inoculations (INOC). Initially, TEMP featured two levels: 25/20°C (Low) and 35/30°C (High) day/night temperature cycles, later expanding to three levels with the addition of an acute heat shock treatment (Heat) at 47°C for 6.5 hours. The heat shock treatment was exclusively applied to plants grown under 35/30°C conditions.
The second factor, INOC, consisted of four levels: mock-inoculated control (Ctrl), ErMF-inoculated (Myc), PGPB-inoculated (Bac), and a combined Myc and Bac inoculation (MyBa). Each treatment condition was initially set with 10 biological replications in the design, although specific analyses involved subsampling.
Physiological measurements – diurnal and weekly gas exchange and chlorophyll fluorescence, and area estimation for newly expanded leaves
Leaf gas exchange and chlorophyll fluorescence of the blueberry leaves were monitored weekly over the experiment and daily on a day close to harvest. A newly developed fully expanded youngest leaf was clamped on a handheld porometer/fluorometer (LI-600, LI-COR Biosciences, Lincoln, NE, USA) and parameters, including stomatal conductance (gs), transpiration rate (E), leaf temperature (Tleaf), and the actual quantum yield of photosystem II (ΦPSII) were recorded. Weekly measurements were performed around 12:00, at 37, 70, 77, 81, 85, 93, 96, and 101 d after inoculation (DAI). Diurnal measurements were conducted commencing 2 hours before the onset of light at 4:00 until 2 hours after its cessation at 20:00, at 2-hour intervals on November 14, 2023, at 101 DAI.
Separately, net photosynthetic CO2 assimilation rate (A) were determined using a portable photosynthesis measuring instrument (GFS-3000, Heinz Walz, Effeltrich, Germany) with an LED cuvette in between 8:00 and 14:00 on November 26–28, 113–115 DAI. The cuvette air flow was set to 750 µmol s−1, CO2, temperature maintained at 25°C, relative humidity regulated at 60%, light intensity fixed at 800 µmol m−2 s−1 PPFD, and CO2 concentration set to 400 µmol mol−1 air.
Including the measured expanded leaves, the leaf area of the newest fully expanded leaves was estimated using a formula derived from linear measurements of leaf length and width.Citation34
Biomass and oxidative stress measurements
Plant samples were destructively collected at harvest on December 3, 2023, at 120 DAI to obtain biomass data. Tissues for oxidative stress assessments, including evaluations of oxidative damage indicators – H2O2, MDA, and leaf browning were sampled twice before and after the heat shock treatment at around 12:00 on November 29, 2023, at 116 DAI and December 3, at 120 DAI, respectively. Biomass analysis encompassed total leaf count, fresh weight (FW), and dry weight (DW) measurements for leaves, stems, and roots. Root/shoot ratios and relative water content were computed using FW and DW measurements. Oxidative damage assessments involved pre- and post-heat shock treatment samples grown only under 35/30°C conditions.
For H2O2 analysis,Citation35 samples from the newest expanded leaf were weighed (0.1 g) and crushed under liquid nitrogen. Each 0.1-g sample was combined with 0.25 mL of 0.1% trichloroacetic acid, 0.25 mL of 10 mM potassium phosphate, and 0.5 mL of 0.1 M potassium iodide. After centrifugation at 4°C for 15 minutes (at 12,000 × g), 200 µL of the supernatant was transferred to a 96-cell plate and left to react in darkness for 20 minutes, using a standard created directly with H2O2 in place of a sample.
Regarding MDA analysis,Citation36 powdered samples were mixed with 1.3 mL of 1% trichloroacetic acid and centrifuged for 20 minutes at 25°C (at 10,000 × g). Subsequently, 0.65 mL of the supernatant was mixed with 0.65 mL of 20% trichloroacetic acid and 5% tert-butyl-alcohol, heated to 95°C in a water bath, and then cooled for 5 minutes. After subsequent centrifugation, the resulting solution was measured using an ELISA analyzer at 532 nm and 600 nm wavelengths, determining MDA levels.
The leaf browning symptoms were visually indexed as a proportion of the brown leaf counts over the total leaf counts using a self-made scale (Figure S1).
Statistical analysis – ANOVA and contrast matrix
We conducted the assumptions check for ANOVA models. The model residuals’ normality check was performed visually by plotting quantile-quantile plots utilizing ‘ggqqplot()’ function from ‘ggpubr’ package, and outliers were eliminated from the data sets. Then, the equality of variance of the data sets was examined by Levene’s test utilizing ‘levene_test()’ function from ‘rstatix’ package, and we confirmed that P-values of the test results were greater than 0.05. For those with p < 0.05, we confirmed the sample size was the same from each group to ensure the robustness of ANOVA against violations of equal variances.
A two-way ANOVA model was used to analyze the two main effects – TEMP, INOC, and interaction effects (TEMP × INOC) on the measured variables. There were two levels in TEMP, Low and High, while four levels existed in INOC, Ctrl, Myc, Bac, MyBa. For time-series data, we used the ANOVA model to analyze the data collected at each time point. A one-way ANOVA was also employed within each temperature treatment to further examine INOC in details. Once significant differences were found in the one-way ANOVA, post hoc analysis to separate the means among treatments was conducted using Tukey’s HSD.
Contrasts matrices were also constructed to compare grouped treatments within TEMP treatments. Contrast 1 to set Ctrl against Myc, Bac, and MyBa combined for a comparison of control with all the PGPM inocula, Contrast 2 to set Myc against Bac for a comparison between ErM and PGPB, and Contrast 3 to set Myc/Bac against MyBa for a comparison between single-type and multi-type inocula.
All analyses were carried out using R statistical packageCitation37 in RStudio and significance codes were reported at p < 0.10, 0.05, 0.01, and 0.001 levels.
Results
Morphological traits of micropropagated blueberry plantlets altered by PGPM inoculations
The morphological traits of micropropagated blueberry plantlets were impacted by PGPM inoculations. Notably, plants inoculated with ErM displayed a more extensive root architecture, while those inoculated with PGPB exhibited larger leaves but a reduced leaf count (). Root responses by Myc were prominent under lower temperatures (25/20°C), whereas Bac-inoculated plants demonstrated distinct leaf responses under higher temperatures (35/30°C). The inoculation of ErM and PGPB significantly influenced blueberry growth under both 25/20°C and 35/30°C day-night temperature conditions.
Figure 1. Morphology of the micro-propagated blueberry plantlets (a) above ground and (b) below ground at harvest. The illustrated plantlets were the representatives of each treatment group. The blueberry plantlets were treated with a mock inoculum (ctrl), a mix of ericoid mycorrhizae inoculum (myc), a single-strain thermotolerant plant growth-promoting bacterial inoculum (Bac), and a combination of the two inocula (MyBa).

Visual observations aligned with biomass data. Plants under 35/30°C conditions displayed a greater number of leaves but with smaller leaf areas than those under 25/20°C conditions (). While no significant effect was detected on leaf counts due to temperature alone, a significant interaction effect between temperature and inoculation (TEMP × INOC) was observed (). Under high-temperature conditions, PGPM-inoculated plants from Myc, Bac, and MyBa exhibited lower leaf counts compared to control plants. Bac-inoculated plants under the high-temperature treatment had a 37% lower leaf count than control plants. Notably, a highly significant temperature effect was found on leaf fresh weight (FW), with plants under 35/30°C displaying lower FW than those under 25/20°C.
Table 1. Biomass allocation and physical traits of the blueberry plantlets at harvest under low (25/20°C) and high air temperature (35/30°C) conditions. The blueberry plantlets were treated with a mock inoculum (ctrl), a mix of ericoid mycorrhizae inoculum (myc), a single-strain thermotolerant plant growth-promoting bacterial inoculum (Bac), and a combination of the two inocula (MyBa).
Regarding root biomass (), significant TEMP and TEMP × INOC effects were observed on both root FW and DW (). Particularly under 25/20°C conditions, ErM inoculation increased root FW by 47% compared to control plants. However, the dual inoculation of ErM and PGPB at the same temperature did not increase root FW and DW; instead, a marginal decrease was observed compared to control plants.
A notable trend in biomass data was a 9% increase in total RWC with Bac inoculation under 35/30°C conditions (). Although not statistically significant, tissues from Bac-inoculated plants – leaves, stems, and roots – tended to exhibit higher RWC than control plants.
Stomatal conductance responses to ErM and PGPB inoculations
The influence of beneficial ErM and PGPB inoculations on blueberry growth under varying temperature conditions (25/20°C and 35/30°C) revealed distinct impacts on gs.
Comparative analysis between different inoculation types demonstrated consistent variations in gs during daytime hours. Specifically, plants with PGPB inoculation consistently exhibited lower diurnal gs compared to control plants, observed almost consistently across the monitored d (). Conversely, plants inoculated solely with ErM displayed higher gs and E (data not shown) than control plants.
Throughout the day, a significant effect of temperature on gs was evident, with plants under 35/30°C conditions displaying higher gs than those under 25/20°C conditions (). Notably, an INOC effect was observed from 8:00 to 18:00, with a significant TEMP × INOC effect specifically detected at 10:00. Both Myc and Bac inoculations demonstrated distinct stomatal behavior and resultant transpiration patterns compared to control plants ( and S2).
Figure 2. Diurnal (a) stomatal conductance (gs) and (b) actual quantum yield of PSII (ΦPSII) of the blueberry leaves under low (25/20°C) and high air temperature (35/30°C) conditions. The blueberry plantlets were treated with a mock inoculum (ctrl), a mix of ericoid mycorrhizae inoculum (myc), a single-strain thermotolerant plant growth-promoting bacterial inoculum (Bac), and a combination of the two inocula (MyBa). Error bars indicate ±1 SEs of the means (n = 10). Different alphabetical letters indicate significant difference between treatment groups separated by the Tukey’s HSD post hoc analysis at α = 0.05 level. Statistical significance codes: °, *, **, *** for α = 0.10, 0.05, 0.01, and < 0.001, respectively.

Myc-treated plants exhibited increased gs and E during peak photosynthetic gas exchange times (10:00–16:00), although a midday depression was noted. Particularly at 14:00 and 16:00 under 25/20°C and at 16:00 under 35/30°C, Myc-treated plants showcased a three-fold increase in gs compared to control plants (Figure S2a). By contrast, Bac inoculation led to decreased gs and E during active photosynthetic periods (10:00–16:00), extending until 18:00 under 35/30°C ( and S2b). Combining Myc and Bac (MyBa) seemed to neutralize these effects, displaying no significant difference in gs and E compared to control plants ( and S2c).
Weekly development of gs did not consistently show a temperature-dependent effect across time (). However, a significant INOC effect was observed in most measured points when Low and High temperature plants were pooled together. The TEMP × INOC interaction effect, although significant at 70 and 96 DAI, exhibited variations.
Figure 3. Weekly (a) stomatal conductance (gs) and (b) actual quantum yield of PSII (ΦPSII) of the blueberry leaves under low (25/20°C) and high air temperature (35/30°C) conditions. The blueberry plantlets were treated with a mock inoculum (ctrl), a mix of ericoid mycorrhizae inoculum (myc), a single-strain thermotolerant plant growth-promoting bacterial inoculum (Bac), and a combination of the two inocula (MyBa). The responses are plotted on a days-after-inoculation (DAI) scale. Error bars indicate ±1 SEs of the means (n = 10). Different alphabetical letters indicate significant difference between treatment groups separated by the Tukey’s HSD post hoc analysis at α = 0.05 level. Statistical significance codes: °, *, **, *** for α = 0.10, 0.05, 0.01, and < 0.001, respectively.

Significant differences in gs between control and inoculated plants were noted across various time points, particularly more pronounced under 35/30°C compared to 25/20°C. However, these differences were not consistently detected at an individual level in the post-hoc Tukey’s test, being valid only when Low and High temperature plants were grouped together. The observed stomatal behavior, contrasting between Myc-increased gs and Bac-decreased gs, was supported by Contrast 2 analysis (Myc vs. Bac).
Improvement in photosynthetic capacity through light harvesting and CO2 assimilation
The introduction of ErM and PGPB significantly influenced the photosynthetic capacity in terms of light-harvesting (actual quantum yield of PSII, ΦPSII – ) and CO2 assimilation (net CO2 assimilation rate A – ) within the photosynthetically active newly expanded leaf areas of blueberry plants grown under day-night temperatures of 25/20°C and 35/30°C.
Figure 4. Newly expanded leaf (a) area and (b) net CO2 assimilation rate (A) of the blueberry plantlets under low (25/20°C) and high air temperature (35/30°C) conditions. The blueberry plantlets were treated with a mock inoculum (ctrl), a mix of ericoid mycorrhizae inoculum (myc), a single-strain thermotolerant plant growth-promoting bacterial inoculum (Bac), and a combination of the two inocula (MyBa). Error bars indicate ±1 SEs of the means (n = 10 and 3 for (a) and (b), respectively). Different alphabetical letters indicate significant difference between treatment groups separated by the Tukey’s HSD post hoc analysis at α = 0.05 level. Statistical significance codes: °, *, **, *** for α = 0.10, 0.05, 0.01, and < 0.001, respectively.

Distinct significance was observed in plants solely inoculated with PGPB, particularly evident under 35/30°C conditions (). Contrarily, ErM-inoculated plants did not exhibit significant improvements compared to control plants. However, under 25/20°C, dual inoculation with ErM and PGPB increased the size of the newest expanded leaves (). Remarkably, plants solely inoculated with PGPB displayed the broadest newly expanded leaves, particularly under 35/30°C conditions.
Diurnal responses highlighted the significance of temperature, notably the increase in ΦPSII overall from 8:00 to 16:00 under High temperatures (). Differences by inoculation were significant only at 12:00 and 14:00. However, unlike stomatal responses, the interaction effect (TEMP × INOC) was negligible for Myc and Bac treatments.
Under 35/30°C, Bac inoculation and the combined MyBa inoculation effectively increased ΦPSII by 16% and 19%, respectively, at 12:00. Except for these rises, ΦPSII remained relatively stable throughout the day.
Weekly measurements showed overall significance in temperature, indicating higher temperatures (35/30°C) increased ΦPSII (). Notable differences by inoculation were detected at 70 DAI, revealing a 3% decrease in ΦPSII with Myc and a 4% increase with Bac.
Significant differences in leaf area were found across and within temperature treatments (), indicating increased leaf area for all inoculation treatments compared to control. Under 25/20°C conditions, Myc, Bac, and MyBa increased leaf area by 12%, 38%, and 64%, respectively. At 35/30°C, Bac significantly increased leaf area by 80%, differing from Myc and MyBa treatments.
Regarding photosynthetic CO2 fixation, only overall temperature significance was noted in the two-way ANOVA (). Plants under High temperatures exhibited greater A values. Notably, within 35/30°C, Bac-inoculated plants displayed higher A than other treatments.
Elevation of ROS and MDA levels and heat shock responses
Upon subjecting plants to a 47°C heat shock for 6.5 hours, the impact of PGPB inoculation was notably adverse. Plants inoculated with PGPB exhibited higher levels of ROS and MDA contents () along with a greater percentage of browning () compared to control plants and those inoculated solely with ErM.
Figure 5. Oxidative stress indicator (a) hydrogen peroxide (H2O2) and (b) malondialdehyde (MDA) contents of the blueberry leaves under low (25/20°C) and high air temperature (35/30°C) conditions. The blueberry plantlets were treated with a mock inoculum (ctrl), a mix of ericoid mycorrhizae inoculum (myc), a single-strain thermotolerant plant growth-promoting bacterial inoculum (Bac), and a combination of the two inocula (MyBa). Error bars indicate ±1 SEs of the means (n = 5). Different alphabetical letters indicate significant difference between treatment groups separated by the Tukey’s HSD post hoc analysis at α = 0.05 level. Statistical significance codes: °, *, **, *** for α = 0.10, 0.05, 0.01, and < 0.001, respectively.

Figure 6. Leaf browning symptoms after heat shock treatment (exposure to 47°C for 6.5 hours) of the blueberry leaves. The illustrated plantlets in (a) were the representatives of each treatment group with (b) the proportion of the brown leaves as a severity index. The blueberry plantlets were treated with a mock inoculum (ctrl), a mix of ericoid mycorrhizae inoculum (myc), a single-strain thermotolerant plant growth-promoting bacterial inoculum (Bac), and a combination of the two inocula (MyBa). Error bars indicate ±1 SEs of the means (n = 5). Different alphabetical letters indicate significant difference between treatment groups separated by the Tukey’s HSD post hoc analysis at α = 0.05 level. Statistical significance codes: °, *, **, *** for α = 0.10, 0.05, 0.01, and < 0.001, respectively.

The temperature effect on ROS contents in blueberry leaves was highly significant (), attributed mainly to the heat shock treatment. Correspondingly, the INOC effect in the two-way ANOVA was also notably significant. Moreover, the interaction (TEMP × INOC) showed a strong positive additive influence between temperature and inoculation on ROS production in blueberry leaves. Specifically, while the Myc treatment did not notably elevate ROS levels, the Bac treatment demonstrated a 58% increase following the 47°C heat shock. Similarly, the dual inoculation, MyBa, exhibited a 49% increase compared to control plants, albeit with marginal significance.
The statistical analysis of MDA contents mirrored the patterns observed in the ROS data (). Significant INOC effects were evident within each temperature treatment, including Low, High, and Heat. Contrast 2 (Myc vs. Bac) showed significance under both 35/30°C and 47°C, indicating that while the MDA contents of Myc-treated plants remained similar to control plants, Bac-treated plants displayed a two-fold and four-fold increase under High and Heat, respectively. Contrast 1 (Ctrl vs. Inoc) was significant for both ROS and MDA contents, signifying an overall increase in these indicators for all inoculations compared to control levels.
Distinct leaf browning symptoms, indicative of heat stress after the 6.5-hour heat shock treatment, were predominantly observed in Bac-treated plants (). The percentage of brown leaves increased by 157% with Bac inoculation compared to other treatments ().
Discussion
Adaptability of the blueberry cultivar “jewel” to high temperatures
The examination of morphological traits and biomass data provides compelling evidence affirming the commendable adaptability of the blueberry cultivar “Jewel” to high temperatures,Citation5 precisely at 35/30°C. Despite a reduction in total DW () compared to growth under 25/20°C conditions, “Jewel” exhibited no severe observable symptoms of heat stress on both physical and physiological components.
The noteworthy increase in leaf count in control plants under 35/30°C suggests a strategic response of “Jewel” to high temperatures (). This observed modification in leaf traits, expanding the leaf surface to facilitate evaporative cooling, further underscores the cultivar’s ability to mitigate heat stress effectively. The resilience of “Jewel” in maintaining root FW and DW through Myc inoculation reinforces the positive adaptation to high temperatures, even though the confined pot size used in the present study limited the potential magnitude of this increase.
Notably, the cultivar’s tendency to increase RWC under high-temperature conditions () serves as an additional indicator of its adaptability to a sub-tropical climate. This response aligns with the broader strategy of “Jewel” to cope with elevated temperatures by enhancing water retention.Citation2
Further evidence supporting the cultivar’s adaptability emerges from the augmented photosynthetic performance (). The increase in stomatal opening, leading to enhanced evapotranspiration and photosynthetic CO2 uptake, indicates an efficient utilization of resources under high-temperature conditions. The concurrent improvement in light harvesting efficiency contributes to the overall robust photosynthetic response observed in “Jewel.” However, it is notable that while photosynthetic responses were prominent, the biomass increase () was not as pronounced. This could be attributed to elevated respiration rates, potentially limiting the availability of carbon for biomass synthesis under high temperatures.Citation38
Differential stomatal responses induced by symbiotic microbial treatments
The natural adaptation of plants to higher temperatures involves increased gs and E at 35/30°C compared to baseline levels at 25/20°C, serving to reduce heat accumulation on leaf surfaces ().Citation39 However, the inoculation of ErM and PGPB introduces nuanced adjustments to stomatal behavior.
ErM inoculation notably promotes greater stomatal opening (). Similarly to our results, studies in arbuscular mycorrhizae symbiotic plants reported a 24% increase in gs, leading to promoting photosynthetic CO2 uptake and eventually biomass gain.Citation40 Although differences can be found in the symbiotic interactions between arbuscular mycorrhizae and ErM, the increases in gs could be attributed to ErM’s secondary effect in enhancing ericaceous plant roots and acting as growth bio-stimulants.Citation41,Citation42 This stimulation likely augments overall plant biomass, especially root development, as observed in our research. While gs increased at both 25/20°C and 35/30°C, there was no significant difference in plant DW at 35/30°C, potentially indicating an optimal temperature threshold for ErM activity, likely below 30°C, aligning with optimal temperature constraints observed in other mycorrhizal types.Citation43,Citation44
Conversely, PGPB inoculation significantly decreases gs in plants (). This aligns with prior research on the relationship between Salicaceae bacterial inoculation and gs in rice plants.Citation45 The reduction in gs might result from Klebsiella sp., the PGPB strain used, potentially producing abscisic acid (ABA), known for inducing stomatal closure.Citation46–48 While this behavior conserves water during stress, it could prove detrimental during heat shock. Stomata, opened to facilitate evaporative cooling, might cause increased damage during heat shock, as evidenced by severe browning in plants inoculated with Klebsiella sp. following exposure to 47°C heat shock for 6.5 hours. Additionally, we hypothesized that respiratory CO2 produced by PGPB, potentially an endophytic bacterium, might influence the photosynthetic CO2 assimilation of host plants,Citation49 suggesting a possible endophytic association between Klebsiella sp. and the blueberry host.
The combined ErM and PGPB treatment, MyBa, resulted in an overall decrease in gs in both conditions ( and S2c), suggesting the overriding effects of PGPB over ErM. This observation supports the hypothesis that PGPB-induced ABA accumulation leads to stomatal closure, potentially indicating that ErM effects are primarily confined to the root zone with lesser influence on above-ground plant components. This might also imply a differential water loss in Myc and Bac plants, supported by increased total RWC exclusively found in Bac plants at 35/30°C.
Enhanced photosynthetic capacity induced by ErM and PGPB, impact on biomass
Under 35/30°C conditions, blueberry plants inoculated with PGPB exhibited significantly higher ΦPSII () and A () than other treatments. This elevation in ΦPSII by PGPB at higher temperatures might denote an augmented heat stress tolerance conferred to the blueberry host by the PGPB strain, enabling superior photosynthetic performance at elevated temperatures. Similar enhancements in light reactions, represented by chlorophyll fluorescence parameters in stressed plants, have been reported across various species.Citation50,Citation51 The reported benefits of PGPB on photosynthesis further support these findings.Citation52–54 Notably, the optimal temperature for the PGPB strain Klebsiella sp. is around 30°C,Citation55 potentially explaining why plants inoculated with PGPB did not perform as well under 25/20°C. This strain, collected from a high-temperature environment,Citation33 might have undergone natural selection for thermophilic or thermotolerant traits.
In contrast, inoculation with ErM did not induce any noticeable alterations in the photosynthetic rate under all conditions (). While limited research focuses specifically on ErM, general studies on mycorrhizae have shown an association with increased photosynthesis ratesCitation56; thus, our results do not agree with such findings. Mycorrhizae act as robust carbon sinks, compelling plants to produce more energy to sustain these sinks.Citation57 Additionally, mycorrhizae can influence root architecture,Citation58 and the uptake of the key nutrients,Citation59 potentially leading to overall changes in plant photosynthesis. Notably, our ErM treatment did elevate root biomass under 25/20°C conditions.Citation60
Possible increased water-use efficiency by PGPB
One of the most intriguing observations involves the interplay between photosynthesis (ΦPSII and A) () and transpiration (gs and E) () in blueberry plants inoculated with PGPB and grown under 35/30°C conditions. Remarkably, these plants displayed elevated ΦPSII and A alongside reduced gs and E during peak photosynthetic activity, hinting at potentially heightened water-use efficiency (WUE). Water-use efficiency is often quantified through the ratio of photosynthesis rate to stomatal conductance defined at the leaf level,Citation61 suggesting that the observed low gs and E could signify enhanced WUE in these plants.
Similar outcomes have been documented in studies showcasing increased WUE in commercial olives following inoculation with beneficial bacteria.Citation53 While not a direct aim of the bacteria, the observed improvement in WUE could be a derivative effect stemming from several factors, including the production of ABA, respiratory CO2 affecting the intercellular CO2 concentration, and ROS generation, all known to induce stomatal closure.Citation45 Moreover, the increase in total RWC by Bac under 35/30°C conditions aligns with our hypothesis of enhanced WUE in Bac-inoculated plants.
The enhanced WUE observed with PGPB suggests that these host blueberry plants could outperform non-symbiotic plants under drought conditions, presenting a significant finding concerning potential applications for agricultural practices in water-limited environments. The WUE enhancement achieved by PGPB could be comparable to genetic approaches to enhance WUE by regulating stomata development and density.Citation62
More oxidative damage in symbiotic plants under extreme temperature
Normally, the upsurge in ROS within host plants triggered by symbiotic relationships primes them for stress responses, eventually leading to enhanced antioxidant production systems and reduced cell damage, often indicated by decreased MDA levels.Citation63 However, our findings, as evident in the ROS and MDA contents data, deviate from this expected trajectory (). Interestingly, our results suggest a contrasting pattern.
The ROS produced by endophytic microbes might contribute to an overall increase in the total ROS content of the host plant. When a specific threshold is surpassed, such as the 47°C threshold in our current study, the benefits previously conferred might be negated, giving way to incurred costs. These costs may manifest as exacerbated cell damage within the host plant, potentially leading to cellular breakdown.
Therefore, it becomes crucial for host plants to strike a delicate balance between the advantages derived from symbiotic interactionsCitation64 – where the signaling ROS acts as alerts – and the potential costs associated with heightened exposure to cellular damage and eventual cell death. This interplay underscores the complexity of symbiotic relationships under extreme stress conditions.
Exploring compound symbiotic effects – prospect for future studies
In forthcoming investigations, a nuanced exploration of below-ground changes influenced by Myc and above-ground alterations affected by Bac warrants attention. Myc exerts a more pronounced influence on root architecture and biomass relationships, while Bac significantly impacts stomatal development and canopy architecture. Understanding the independent or interdependent effects of these behavioral and developmental alterations during drought stress or combined drought-heat conditions is a pivotal future inquiry.Citation65 Whether these changes work synergistically or counteract each other in impacting WUE along distinct pathways remains unclear. Additionally, delving into carbon relations is an intriguing avenue, given our observation of increased photosynthetic rates that did not necessarily translate to amplified biomass due to potential carbon drain from the symbionts.Citation66 Re-evaluating “Jewel”’s heat stress threshold could provide further clarity in interpreting these interactions and reaching out to applications.
Conclusion
This study has shown the multifaceted effects of ErM and PGPB on the growth of micropropagated blueberry plants “Jewel,” with outcomes significantly influenced by the ambient temperature in which the plants and microorganisms are situated. Notably, the presence of ErM increased the root biomass under low temperatures at 25/20°C. Furthermore, ErM increased gs under both temperatures at 25/20°C and 35/30°C. Conversely, the presence of PGPB is associated with a significant reduction of gs under both temperatures while increasing the plant’s photosynthetic capacity, represented by ΦPSII and A, under warmer temperatures at 35/30°C. However, the presence of PGPB increased the amount of ROS in the form of H2O2 and MDA under both conditions. In addition, the integration of both ErM and PGPB appeared to increase leaf area, especially under low temperature conditions. These results showed the complex biotic and abiotic interactions between ErM, PGPB, the blueberry host plant, and the environment. Despite these promising findings, the present study lacks direct physical or chemical evidence of successful inoculation, underlining the gap that justifies further research and experiments to be conducted.
PSB_FigS2_Fransgo_.tiff
Download TIFF Image (1.2 MB)PSB_FigS1_Fransgo_.tif
Download TIFF Image (1.5 MB)Acknowledgments
The authors acknowledge Drs. Kuo-Tan Li and Hiran Ariyawansa at National Taiwan University for providing the plant and the bacterial materials used in the study, respectively. Also, we thank Dr. Syuan-You Lin at National Chung Hsing University for providing thoughtful insights into interpreting the results of the work. This study was supported by a fund from National Taiwan University [NTU-112L9005].
Disclosure statement
No potential conflict of interest was reported by the author(s).
Supplementary material
Supplemental data for this article can be accessed online at https://doi.org/10.1080/15592324.2024.2329842.
Additional information
Funding
References
- International Blueberry Organization. Global state of the blueberry industry report. Brazelton C, ed., 2023.
- Wahid A, Gelani S, Ashraf M, Foolad MR. Heat tolerance in plants: an overview. Environ Exp Bot. 2007;61(3):199–13. doi:10.1016/j.envexpbot.2007.05.011.
- Seleiman MF, Al-Suhaibani N, Ali N, Akmal M, Alotaibi M, Refay Y, Dindaroglu T, Abdul-Wajid HH, Battaglia ML. Drought stress impacts on plants and different approaches to alleviate its adverse effects. Plants. 2021;10(2):259. doi:10.3390/plants10020259.
- Retamales JB, Hancock JF. Blueberries. Cabi. 2018.
- Medina RB, Cantuarias-Avilés TE, Angolini SF, Silva SRd. Performance of ‘Emerald’ and ‘jewel’ blueberry cultivars under no-chill incidence. Pesqui Agropecu Trop. 2018;48:147–152. doi:10.1590/1983-40632018v4852093.
- Lyrene P. Development of highbush blueberry cultivars adapted to Florida. J Am Pomol Soc. 2002;56:79–85.
- Watkinson SC. Chapter 7 – mutualistic symbiosis between fungi and autotrophs. In: Watkinson S, Boddy L Money N. editors. The fungi. (Third Edition). Boston: Academic Press; 2016. pp. 205–243.
- Cullings KW. Single phylogenetic origin of ericoid mycorrhizae within the ericaceae. Can J Bot. 1996;74(12):1896–909. doi:10.1139/b96-227.
- Vohník M, Réblová M. Fungi in hair roots of Vaccinium spp. (ericaceae) growing on decomposing wood: colonization patterns, identity, and in vitro symbiotic potential. Mycorrhiza. 2023;33(1–2):69–86. doi:10.1007/s00572-023-01101-z.
- Valenzuela-Estrada LR, Vera-Caraballo V, Ruth LE, Eissenstat DM. Root anatomy, morphology, and longevity among root orders in Vaccinium corymbosum (ericaceae). Am J Bot. 2008;95(12):1506–14. doi:10.3732/ajb.0800092.
- Kamran M, Imran QM, Ahmed MB, Falak N, Khatoon A, Yun B-W. Endophyte-mediated stress tolerance in plants: a sustainable strategy to enhance resilience and assist crop improvement. Cells. 2022;11(20):3292. doi:10.3390/cells11203292.
- Ważny R, Jędrzejczyk RJ, Rozpądek P, Domka A, Turnau K. Biotization of highbush blueberry with ericoid mycorrhizal and endophytic fungi improves plant growth and vitality. Appl Microbiol Biotechnol. 2022;106(12):4775–86. doi:10.1007/s00253-022-12019-5.
- Mu D, Du N, Zwiazek JJ. Inoculation with ericoid mycorrhizal associations alleviates drought stress in lowland and upland velvetleaf blueberry (Vaccinium myrtilloides) seedlings. Plants. 2021;10(12):2786. doi:10.3390/plants10122786.
- Scagel CF. Inoculation with ericoid mycorrhizal fungi alters fertilizer use of highbush blueberry cultivars. HortScience. 2005;40(3):786–94. doi:10.21273/HORTSCI.40.3.786.
- de Silva A, Patterson K, Rothrock C, Moore J. Growth promotion of highbush blueberry by fungal and bacterial inoculants. HortScience. 2000;35(7):1228–30. doi:10.21273/HORTSCI.35.7.1228.
- Contreras-Pérez M, Hernández-Salmerón J, Rojas-Solís D, Rocha-Granados C, Orozco-Mosqueda M, Parra-Cota FI, de Los Santos-Villalobos S, Santoyo G. Draft genome analysis of the endophyte, Bacillus toyonensis COPE52, a blueberry (Vaccinium spp. var. Biloxi) growth-promoting bacterium. 3 Biotech. 2019;9(10):1–6. doi:10.1007/s13205-019-1911-5.
- Yu YY, Xu JD, Huang TX, Zhong J, Yu H, Qiu JP, Guo JH. Combination of beneficial bacteria improves blueberry production and soil quality. Food Sci Nutr. 2020;8:5776–84. doi:10.1002/fsn3.1772.
- Chacón FI, Sineli PE, Mansilla FI, Pereyra MM, Diaz MA, Volentini SI, Poehlein A, Meinhardt F, Daniel R, Dib JR. et al. Native cultivable bacteria from the blueberry microbiome as novel potential biocontrol agents. Microorganisms. 2022;10(5):969. doi:10.3390/microorganisms10050969.
- Rad AK, Zarei M, Astaikina A, Streletskii R, Etesami H. Chapter 1 - effects of microbial inoculants on growth, yield, and fruit quality under stress conditions. In: Seymen M, Kurtar E, Erdinc C, and Kumar A. editors. Sustainable Horticulture : Microbial Inoculants and Stress Interaction. London: Academic Press; 2022. p. 1–38.
- Liu C, Callow P, Rowland LJ, Hancock JF, Song G-q. Adventitious shoot regeneration from leaf explants of southern highbush blueberry cultivars. Plant Cell, Tissue Organ Culture (PCTOC). 2010;103(1):137–144. doi:10.1007/s11240-010-9755-z.
- Wang Y, Chen C, Li K. The establishment of the plantlet production of rabbiteye blueberry through tissue culture from in vitro derived leaf explants. J Taiwan Soc Hortic Sci. 2014;60:51–63.
- Cappai F, Amadeu RR, Benevenuto J, Cullen R, Garcia A, Grossman A, Ferrão LFV, Munoz P. High-resolution linkage map and QTL analyses of fruit firmness in autotetraploid blueberry. Front Plant Sci. 2020;11:562171. doi:10.3389/fpls.2020.562171.
- Cappelletti R, Sabbadini S, Mezzetti B. The use of TDZ for the efficient in vitro regeneration and organogenesis of strawberry and blueberry cultivars. Sci Hortic (Amsterdam). 2016;207:117–24. doi:10.1016/j.scienta.2016.05.016.
- Gajdošová S, Spíchal L, Kamínek M, Hoyerová K, Novák O, Dobrev PI, Galuszka P, Klíma P, Gaudinová A, Žižková E. et al. Distribution, biological activities, metabolism, and the conceivable function of cis-zeatin-type cytokinins in plants. J Exp Bot. 2011;62(8):2827–40. doi:10.1093/jxb/erq457.
- Fan S, Jian D, Wei X, Chen J, Beeson RC, Zhou Z, Wang X. Micropropagation of blueberry ‘bluejay’ and ‘pink lemonade’ through in vitro shoot culture. Sci Hortic (Amsterdam). 2017;226:277–84. doi:10.1016/j.scienta.2017.08.052.
- Li Q, Yu P, Lai J, Gu M. Micropropagation of the potential blueberry rootstock—vaccinium arboreum through axillary shoot proliferation. Sci Hortic (Amsterdam). 2021;280:109908. doi:10.1016/j.scienta.2021.109908.
- Pacholczak A, Nowakowska K. The ex vitro rooting of blueberry (Vaccinium corymbosum L.) microcuttings. Folia Hortic. 2015;27(2):145–150. doi:10.1515/fhort-2015-0024.
- Lin L-C, Lee M-J, Chen J-L. Resynthesis of ericoid mycorrhizae in Formosan rhododendron (rhododendron formosanum hemsl.) with an endophytic cryptosporiopsis species. Taiwan J For Sci. 2011;26:245–254.
- Lin L, Lin C, Lin W, Tung Y, Wu J. Effects of ericoid mycorrhizal fungi or dark septate endophytic fungi on the secondary metabolite of rhododendron pseudochrysanthum (R. Morii) seedlings. Appl Ecol Environ Res. 2021;19(2):1221–1232. doi:10.15666/aeer/1902_12211232.
- Lin L-C, Lin W-R, Hsu Y-C, Pan H-Y. Influences of three oidiodendron maius isolates and two inorganic nitrogen sources on the growth of rhododendron kanehirae. 2020;38(5):742–753. doi:10.7235/HORT.20200067.
- Gagnon J, Langlois C, Bouchard D, Tacon FL. Growth and ectomycorrhizal formation of container-grown Douglas-fir seedlings inoculated with laccaria bicolor under four levels of nitrogen fertilization. Can J For Res. 1995;25(12):1953–61. doi:10.1139/x95-210.
- Lin L-C, Chen H-Y, Lin W-R. Fostering growth in Cinnamomum kanehirae cuttings: the beneficial role of dark septate endophytes in forest nursery management. Forests. 2023;15(1):16. doi:10.3390/f15010016.
- Chan TH, Rho H, Ariyawansa HA. The effects of plant growth-promoting bacteria on the physiological properties, nutrition contents and growth performance in lettuce under heat-stress conditions. International Horticultural Congress; August 14-20; Angers, France; 2022. https://app.ihc2022.org/event/ihc-2022/planning/UGxhbm5pbmdfOTYxMzQ4
- Celik H, Odabas MS, Odabas F. Leaf area prediction models for highbush blueberries (Vaccinium corymbosum L.) from linear measurements. Adv Food Sci. 2011;33:16–21.
- Junglee S, Urban L, Sallanon H, Lopez-Lauri F. Optimized assay for hydrogen peroxide determination in plant tissue using potassium iodide. Am J Anal Chem. 2014;5(11):730–736. doi:10.4236/ajac.2014.511081.
- Ozden M, Demirel U, Kahraman A. Effects of proline on antioxidant system in leaves of grapevine (Vitis vinifera L.) exposed to oxidative stress by H2O2. Sci Hortic (Amsterdam). 2009;119(2):163–8. doi:10.1016/j.scienta.2008.07.031.
- Core-Team R-. R: a language and environment for statistical computing. R foundation for statistical computing. Vienna, Austria; 2021.
- Atkin OK, Tjoelker MG. Thermal acclimation and the dynamic response of plant respiration to temperature. Trends Plant Sci. 2003;8(7):343–51. doi:10.1016/S1360-1385(03)00136-5.
- Taiz L, Zeiger E, Møller IM, Murphy A. Plant physiology and development. New York, United States of America: Sinauer Associates Incorporated; 2015.
- Auge RM, Toler HD, Saxton AM. Arbuscular mycorrhizal symbiosis alters stomatal conductance of host plants more under drought than under amply watered conditions: a meta-analysis. Mycorrhiza. 2015;25(1):13–24. doi:10.1007/s00572-014-0585-4.
- Wei X, Chen J, Zhang C, Liu H, Zheng X, Mu J. Ericoid mycorrhizal fungus enhances microcutting rooting of Rhododendron fortunei and subsequent growth. Hortic Res. 2020;7(1):140. doi:10.1038/s41438-020-00361-6.
- Wei X, Zhang W, Zulfiqar F, Zhang C, Chen J. Ericoid mycorrhizal fungi as biostimulants for improving propagation and production of ericaceous plants. Front Plant Sci. 2022;13:1027390. doi:10.3389/fpls.2022.1027390.
- Gavito ME, Olsson PA, Rouhier H, Medina-Peñafiel A, Jakobsen I, Bago A, Azcón‐Aguilar C. Temperature constraints on the growth and functioning of root organ cultures with arbuscular mycorrhizal fungi. New Phytol. 2005;168(1):179–188. doi:10.1111/j.1469-8137.2005.01481.x.
- Kilpeläinen J, Aphalo PJ, Lehto T. Temperature affected the formation of arbuscular mycorrhizas and ectomycorrhizas in Populus angustifolia seedlings more than a mild drought. Soil Biol Biochem. 2020;146:107798. doi:10.1016/j.soilbio.2020.107798.
- Rho H, Epps VV, Wegley N, Doty SL, Kim S-H. Salicaceae endophytes modulate stomatal behavior and increase water use efficiency in rice. Front Plant Sci. 2018;9:188. doi:10.3389/fpls.2018.00188.
- Bharath P, Gahir S, Raghavendra AS. Abscisic acid-induced stomatal closure: An important component of plant defense against abiotic and biotic stress. Front Plant Sci. 2021;12:615114. doi:10.3389/fpls.2021.615114.
- Kudoyarova G, Arkhipova T, Veselov D. Water relations in plants treated with growth promoting rhizosphere bacteria. Plant Soil. 2024;494(1–2):51–72. doi:10.1007/s11104-023-06270-6.
- Shahzad R, Khan AL, Bilal S, Waqas M, Kang S-M, Lee I-J. Inoculation of abscisic acid-producing endophytic bacteria enhances salinity stress tolerance in Oryza sativa. Environ Exp Bot. 2017;136:68–77. doi:10.1016/j.envexpbot.2017.01.010.
- Rho H, Doty SL, Kim SH. Estimating microbial respiratory CO2 from endophytic bacteria in rice. Plant Signal Behav. 2018;13:e1500067. doi:10.1080/15592324.2018.1500067.
- Rho H, Doty SL, Kim SH, Foyer C. Endophytes alleviate the elevated CO2-dependent decrease in photosynthesis in rice, particularly under nitrogen limitation. J Exp Bot. 2020;71(2):707–18. doi:10.1093/jxb/erz440.
- Woodward C, Hansen L, Beckwith F, Redman RS, Rodriguez RJ. Symbiogenics: an epigenetic approach to mitigating impacts of climate change on plants. HortScience. 2012;47(6):699–703. doi:10.21273/HORTSCI.47.6.699.
- Eid AM, Fouda A, Abdel-Rahman MA, Salem SS, Elsaied A, Oelmüller R, Hijri M, Bhowmik A, Elkelish A, Hassan SED. et al. Harnessing bacterial endophytes for promotion of plant growth and biotechnological applications: an overview. Plants. 2021;10(5):935. doi:10.3390/plants10050935.
- Galicia-Campos E, Ramos-Solano B, Montero-Palmero MB, Gutierrez-Mañero FJ, García-Villaraco A. Management of plant physiology with beneficial bacteria to improve leaf bioactive profiles and plant adaptation under saline stress in Olea europea L. Foods. 2020;9(1):57. doi:10.3390/foods9010057.
- Vitale L, Vitale E, Francesca S, Lorenz C, Arena C. Plant-growth promoting microbes change the photosynthetic response to light quality in spinach. Plants. 2023;12(5):1149. doi:10.3390/plants12051149.
- Hennecke H, Shanmugam KT. Temperature control of nitrogen fixation in Klebsiella pneumoniae. Arc Microbiol. 1979;123(3):259–265. doi:10.1007/BF00406659.
- Gavito ME, Jakobsen I, Mikkelsen TN, Mora F. Direct evidence for modulation of photosynthesis by an arbuscular mycorrhiza-induced carbon sink strength. New Phytol. 2019;223(2):896–907. doi:10.1111/nph.15806.
- Lambers H, Chapin FS, Pons TL. Plant physiological ecology. New York, United States of America: Springer; 2008.
- Atkinson D, Berta G, Hooker JE. Impact of mycorrhizal colonisation on root architecture, root longevity and the formation of growth regulators. In: Gianinazzi S Schüepp H. editors. Impact of arbuscular mycorrhizas on sustainable agriculture and natural ecosystems. Basel: Birkhäuser Basel; 1994. p. 89–99.
- de Vries J, Evers JB, Kuyper TW, van Ruijven J, Mommer L. Mycorrhizal associations change root functionality: a 3D modelling study on competitive interactions between plants for light and nutrients. New Phytol. 2021;231(3):1171–82. doi:10.1111/nph.17435.
- Hetrick BAD. Mycorrhizas and root architecture. Experientia. 1991;47(4):355–62. doi:10.1007/BF01972077.
- Hatfield JL, Dold C. Water-use efficiency: advances and challenges in a changing climate. Front Plant Sci. 2019;10:103. doi:10.3389/fpls.2019.00103.
- Caine RS, Yin X, Sloan J, Harrison EL, Mohammed U, Fulton T, Biswal AK, Dionora J, Chater CC, Coe RA. et al. Rice with reduced stomatal density conserves water and has improved drought tolerance under future climate conditions. New Phytol. 2019;221(1):371–84. doi:10.1111/nph.15344.
- Hamilton CE, Gundel PE, Helander M, Saikkonen K. Endophytic mediation of reactive oxygen species and antioxidant activity in plants: a review. Fungal Divers. 2012;54(1):1–10. doi:10.1007/s13225-012-0158-9.
- Ntoukakis V, Gifford ML. Plant–microbe interactions: tipping the balance. J Exp Bot. 2019;70(18):4583–5. doi:10.1093/jxb/erz321.
- Rowland L, Ramirez-Valiente JA, Hartley IP, Mencuccini M. How woody plants adjust above- and below-ground traits in response to sustained drought. New Phytol. 2023;239(4):1173–89. doi:10.1111/nph.19000.
- Li H, Smith FA, Dickson S, Holloway RE, Smith SE. Plant growth depressions in arbuscular mycorrhizal symbioses: not just caused by carbon drain? New Phytol. 2008;178(4):852–62. doi:10.1111/j.1469-8137.2008.02410.x.