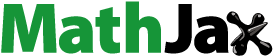
ABSTRACT
Partially hydrolysed poly(2-oxazoline)s possess unique properties. However, much of the focus in this area has been on water soluble poly(2-oxazoline)s. Where hydrophobic poly(2-oxazoline)s have been used, this is often for selective hydrolysis. However, hydrolysis of very hydrophobic polymers could lead to interesting solution behaviour. Herein, we describe universal conditions for the hydrolysis of poly(2-alkyl-2-oxazoline)s suitable for both hydrophobic and hydrophilic 2-oxazolines. We show that the system utilised gives comparable rates to that of water alone for poly(2-ethyl-2-oxazoline). In addition, poly(2-fatty acid-2-oxazoline) was hydrolysed using the developed system and was found to proceed in a controlled manner allowing the targeting of specific degrees of hydrolysis, albeit much slower than for poly(2-ethyl-2-oxazoline). Finally, we demonstrate the partial functionalisation of poly(2-oxazoline)-poly(ethylene imine) co-polymers via aza-Michael addition.
Introduction
Poly(2-oxazoline)s are important bio-medically relevant polymers formed from the cationic ring-opening polymerization (CROP) of 2-oxazolines owing to their improved performance over common biomedical polymers such as poly(ethylene glycol) [Citation1–4]. Notwithstanding the range of commercial monomers available, a number of functional monomers have been reported in the literature [Citation5]. However, monomer design is limited in that nucleophilic side groups have to be avoided which limits the synthetic possibilities of poly(2-oxazoline)s. Therefore, a number of post-polymer modification strategies such as click reactions to functional monomers [Citation6–10], polymer–polymer coupling reactions [Citation11], star polymers via click reactions [Citation12–14], cyclisation [Citation15–21], brush polymers [Citation22–24] as well as hydrolysis [Citation25,Citation26] have been used to increase the functionality and/or create novel macromolecular architectures. In particular, both acidic and basic hydrolysis has received a lot of attention due to the controlled and facile nature of the process in forming poly(2-oxazoline) (POx)/poly(ethylene imine) (PEI) co-polymers [Citation27,Citation28]. Moreover, POx-PEI copolymers show good RNA and DNA transfection with the ability to tune the hydrolysis being of critical importance because the charge density, molecular weight and amount of PEI all impact the properties of the co-polymers [Citation24,Citation29–34]. In addition, comb PEI structures have also been developed utilising the controlled hydrolysis of poly(2-oxazoline)s [Citation32,Citation35]. The hydrolysis of poly(2-ethyl-2-oxazoline) and poly(2-methyl-2-oxazoline) has been extensively studied with numerous studies on the mechanism and kinetics of the hydrolysis [Citation25,Citation29,Citation36]. Indeed, selective hydrolysis of hydrophilic blocks has been demonstrated, allowing the altering of properties, such as solution behaviour [Citation37–39].
Moreover, several post-hydrolysis functionalisation techniques have been explored to further increase the functionality [Citation28]. This allows functional groups to be included in the polymer that would have otherwise been prohibited on account of their CROP interfering properties. Examples of these include acylation [Citation40–42], nucleophilic substitutions to alkyl halides [Citation24] and reductive amination [Citation43]. In addition, Michael additions to linear PEI have been reported [Citation44].
In this study, we report on the kinetics of the hydrolysis of a very hydrophobic poly(2-oxazoline) possessing C17 alkyl chains (PFAOx) in a THF/water co-solvent mixture. In addition, we have applied these conditions to poly(2-ethyl-2-oxazoline) (PEtOx) alongside aqueous systems to measure the impact of this solvent system on the kinetics. Finally, we demonstrate the partial aza-Michael functionalisation of partially hydrolysed poly(2-oxazoline)s with selected vinyl monomers ().
Experimental
Materials
Hydrochloric acid (Fisher, 37%), thiophenol (Fisher, 99%), azobisisobutyronitrile (AIBN, Sigma Aldrich, 98%), propargyl acrylate (Sigma, 98%), 2-hydroxyethyl acrylate (Sigma, 96%), 2-(dimethylamine)ethyl methacrylate (Sigma, 98%) and 1,4-butanediol (Sigma-Aldrich, 98%) were all used as received.
Instruments
Most GPC chromatograms were measured on an Agilent Technologies 1260 Infinity fitted with a refractive index detector, a PLgel 5 µm guard column and a PL gel 5 µm mixed D column (300 × 7.5 mm). THF with 2% triethylamine was used as the eluent. Samples were run at 40 °C with a flow rate of 1 mL min−1 and measured against narrow poly(methyl methacrylate) standards. was measured in a similar way but employed 2× PL gel 5 µm mixed D columns.
All 1H NMR were measured at 298 K on a Bruker HD300 or HD400 in CDCl3.
Procedure for the hydrolysis kinetic of PFAOx
PFAOx (3.8880 g) was dissolved in THF (21 mL) and the resulting stock solution divided into microwave vials in 1.6 mL portions. Thirty-five percent hydrochloric acid (0.4 mL) was added, and the resulting solution reacted at 120 °C for the indicated time. At the end of the reaction, the solution was precipitated into acetone. The resulting solids were then dissolved in THF and neutralised to a pH <9 with ~4 M NaOH(aq). The THF was removed in vacuo with the resulting water removed by freeze drying. To analyse the samples, the polymer was dissolved out of the residue using deuterated chloroform (for 1H NMR) or THF/2% TEA (for GPC).
Procedure for the hydrolysis kinetics of PEtOx
PEtOx (1.0033 g) was dissolved in THF (17 mL) and the resulting stock solution was divided into microwave vials in 1.6 mL portions. Thirty-five percent hydrochloric acid (0.4 mL) was added, and the resulting solution reacted at 120 °C for the indicated time. At the end of the reaction, the solution was neutralised to a pH <9 with ~4 M NaOH(aq). The THF was removed in vacuo with the resulting water removed by freeze drying. The samples were dissolved in deuterated methanol for analysis. The kinetics for the other systems used were identical, replacing THF with water and 1,4-butanediol, as appropriate.
Procedure for the aza-Michael addition to hydrolysed poly(2-oxazoline)s
A typical procedure was as follows: The polymer was dissolved in THF to a concentration of 100 mg/mL and the appropriate amounts of monomer and TEA were added (see ). The reaction was reacted at the temperature and time indicated in . Where purified, the PFAOx Michael addition conjugates were precipitated in methanol, and the PEtOx conjugates were precipitated in diethyl ether.
Table 1. Summary table for the conditions studied for the aza-Michael additions carried out for PEtOx (PE1-PE7) and PFAOx (PF1-PF4.).
Procedure for the thiol-yne reaction
PE4 (25.2 mg, 1 eq.) was dissolved in DMF (0.75 mL) along with AIBN (2.2 mg, 59 mol%) and purged with nitrogen. Thiophenol (0.03 mL, excess) was added, and the reaction was left for 15 h at 90 °C. The reaction was precipitated in diethyl ether before analysis.
Results and discussion
Poly(2-oxazoline) hydrolysis kinetics
shows the hydrolysis kinetics for both PFAOx with a [M]:[I] of 50:1 and several conditions for PEtOx with a [M]:[I] of 46:1. Given the solubility in water of PEtOx, the hydrolysis has been reported to be carried out in aqueous hydrochloric acid at various temperatures, leading to well controlled partially hydrolysed poly(ethyl oxazoline)-poly(ethyleneimine) copolymers [Citation26,Citation29]. PFAOx being inherently hydrophobic is non-water soluble and, therefore, the hydrolysis cannot proceed in a purely aqueous environment. Given this, a tetrahydrofuran (THF)/HClaq 8:2 co-solvent mixture was employed, which assuming complete and thorough mixing of solvent gives an acid concentration of 2.4 M. The amide concentration used was 0.48 M calculated using an average repeating unit molecular weight of 306 Da and 99 Da for PFAOx and PEtOx, respectively.
Figure 1. a) hydrolysis kinetics showing PFAOx hydrolysed in 8:2 THF/HClaq (black), PEtOx hydrolysed in 8:2 THF/HClaq (red), PEtOx hydrolysed in 6.3:1.3:2.4 1,4-butanediol/water/HClaq (blue), PEtOx hydrolysed in 8:2 THF/HClaq at 100 °C (green) and PEtOx hydrolysed in 8:2 water/HClaq (purple). b) corresponding first-order kinetic plot. All reactions were carried out with 35–37% HClaq such that [H+]=2.4 M and [A]=0.48 M. PFAOx hydrolysis was calculated according to EquationEquation 1(1)
(1) and all PEtOx hydrolysis rates were calculated according to Equation 12.
![Figure 1. a) hydrolysis kinetics showing PFAOx hydrolysed in 8:2 THF/HClaq (black), PEtOx hydrolysed in 8:2 THF/HClaq (red), PEtOx hydrolysed in 6.3:1.3:2.4 1,4-butanediol/water/HClaq (blue), PEtOx hydrolysed in 8:2 THF/HClaq at 100 °C (green) and PEtOx hydrolysed in 8:2 water/HClaq (purple). b) corresponding first-order kinetic plot. All reactions were carried out with 35–37% HClaq such that [H+]=2.4 M and [A]=0.48 M. PFAOx hydrolysis was calculated according to EquationEquation 1(1) DegreeofHydrolysis%=100IH,tf−IH,t0IP(1) and all PEtOx hydrolysis rates were calculated according to Equation 12.](/cms/asset/7e3ed19b-299b-47c4-a8e6-2a970c688186/tdmp_a_2267232_f0001_oc.jpg)
Upon dissolution of PFAOx in THF, a phase separation between the organic and aqueous layers is observed at room temperature. Due to this phase separation, a stock solution using both acid and THF was not possible. To allow proper comparison, all other kinetic sequences discussed herein used the same set-up method and therefore any error should be consistent. However, upon heating no phase separation is observed, although upon cooling the separation returns. At the completion of the reactions, the samples were prepared (see experimental) and the 1H NMR was measured to obtain the degree of hydrolysis. In the 1H NMR, the hydrolysis peak overlaps with a side chain peak on the spectrum (see Figure S1, ESI) which means a direct measurement could not be obtained. Therefore, given that the ratio of this peak to the other side chain peaks remains constant at any given degree of hydrolysis, any increase in the integration of this peak can be attributed to the hydrolysis. Hence, the hydrolysis was calculated according to equation EquationEquation 1(1)
(1) , setting the integral value for one of the side chain peaks to the same value in both tfinal and t0:
Where IH,tf is the integral for the hydrolysis co-peak at t final, IH,t0 is the integral for the hydrolysis co-peak at t0 and IP is the integral for the main polymer backbone peak.
As can be seen from , the hydrolysis proceeds in a controlled fashion with the hydrolysis increasing linearly with time. This would allow specific hydrolysis rates to be targeted which could have interesting solubility behaviour.
For comparison, the hydrolysis of PEtOx was also carried out in THF/water using the same conditions as for PFAOx. It is important to note that the target molecular weights of both polymers were different (although the [M]:[I] ratios were approximately equal), however, Hoogenboom et al. [Citation26] found no molecular weight dependency on the hydrolysis kinetics of PEtOx. The appearance of a second peak that overlaps with the non-hydrolysed backbone peak means that this peak could not be used. As hydrolysis progresses the overlap is absent because of a much smaller amount of non-hydrolysed backbone and thus a smaller peak is observed for that species. This extra peak is due to the hydrolysis of THF forming 1,4-butanediol as a side reaction (see Figure S2, ESI). Nevertheless, the non-hydrolysed side chains were used, according to Equation 2. For accurate comparisons, all other PEtOx kinetics also utilised equation 2.
Where IH is the integral for the hydrolysis peak and ICH2 is for the integral of the remaining CH2 groups on the polymer chain.
As can be seen from the figure, PEtOx experiences significantly faster hydrolysis under THF/HClaq 8:2 conditions compared to PFAOx with ca. 90% hydrolysis being reached in 45 min compared to 14% hydrolysis for PFAOx; an over 6-fold difference. Interestingly, the polymer precipitated out of the reaction solution upon cooling down due to the high number of positive charge which make the polymer insoluble in THF. This significant difference in rate is because the rate determining step in the hydrolysis is the nucleophilic addition of water [Citation28]. With PFAOx, the more sterically hindered, hydrophobic polymer chains cause the rate of this nucleophilic attack to be dramatically lowered thus leading to an overall lower hydrolysis rate. In addition, the kinetic for PEtOx using THF/HClaq 8:2 was carried out at 100 °C. As can be seen from , the rate of hydrolysis is lower than at 120 °C, as expected. Highlighting the slower rate of PFAOx hydrolysis, even at 100 °C the hydrolysis of PEtOx, is faster than that of PFAOx at 120 °C under the same conditions.
As previously mentioned, there was evidence of THF hydrolysis in the reaction of PEtOx at 120 °C in THF/HClaq 8:2. In order to investigate if this side reaction had an impact on the kinetics, a kinetic experiment was carried out, but the THF/HClaq 8:2 solvent mixture was replaced with 1,4-butanediol/water/HClaq 6.7:1.3:2 with [A] = 0.48 M. As can be observed from , a very slight acceleration in the rate is observed, but this is not significant and is virtually the same as the THF/HClaq mixture. Similarly, the kinetic was also carried out in water under the same acid and amide concentrations, and the hydrolysis was observed to proceed in the same manner as the THF/HClaq 8:2 mixture. These results suggest that a THF/HClaq mixture gives similar hydrolysis behaviour to water alone, which is important given the poor suitability in aqueous solutions for very hydrophobic poly(2-oxazoline)s.
To verify the results obtained from Equation 2, other methods were also carried out. For the hydrolysis of PEtOx in water two equations, commonly used in the literature [Citation45], were used in addition to Equation 2. These results are plotted and shown in the supplementary information (Figure S3). In general, there was good agreement between all three equations used differing by only a few percent. At higher hydrolysis, the difference was greater but still within approximately 5%.
The hydrolysis of poly(2-oxazoline)s have been reported to proceed in a first-order fashion [Citation26]. Therefore, the first-order plot for the linear portions of the hydrolysis kinetics was plotted for all conditions used and is displayed in . From the slopes, the hydrolysis rate constant, , can be obtained. The results show that the rate of hydrolysis for PFAOx is approximately 10 times slower than PEtOx, under the same conditions. The data also shows that the rate of hydrolysis is very similar for all conditions used, further indicating the suitability of THF/water systems for the hydrolysis of poly(2-oxazoline)s.
shows the GPC chromatograms for the PFAOx kinetic and shows the reduction in apparent molecular weight as the hydrolysis progresses. As expected, a low molecular weight shoulder is evident and increases as the degree of hydrolysis increases. The origin of the low molecular weight tailing is due to increased column interactions as the level of hydrolysis increases. Due to the tailing making integration difficult, rather than using Mn,GPC the Mp,GPC has been used and was plotted along with the degree of hydrolysis (). As can be observed, the Mp,GPC decrease in a linear fashion as the degree of hydrolysis increases. However, the Mp,GPC is far lower than would be expected for the 120-min data point. One potential reason for this could be reduced solubility in THF of the hydrolysed material as hydrolysis increases. Notwithstanding this, taken together, the data suggests that THF/HClaq mixtures are effective for the hydrolysis of both hydrophilic and hydrophobic poly(2-oxazoline)s.
Aza-Michael addition of vinyl monomers to Poly(2-Oxazoline)s
The acylation of (partially) hydrolysed PEtOx has been reported in order to create poly(oxazoline)s with functional groups that otherwise would not be possible from a monomeric route [Citation41,Citation42]. Here, the aza-Michael addition of the PEI secondary amines to vinyl monomers was investigated for PEtOx and PFAOx to provide a route to introducing additional functionality.
PE1-PE7 used a poly(2-ethyl-2-oxazoline) polymer with a targeted [M]:[I] = 100:1 and a targeted 10–13% hydrolysis rate. Although only necessary for the calculation of PE6 and PE7, the starting polymer for all entries except PE1 and PE3 had a hydrolysis rate of 13.1%, with the other hydrolysed polymer having a hydrolysis rate of 11.5%. All hydrolysis was performed in the same way as Hoogenboom et al. [Citation26] utilising the kinetic they obtained at 100 °C in water. All equivalents of base and monomer were calculated using the theoretical molecular weight including the degree of hydrolysis.
Where ICH2 is the integral for the CH2 at 4.5 ppm adjacent to the triple bond and IH is the integral for the hydrolysis peak
For PE1-PE5 (), monomer A (propargyl acrylate) was used because the -CH2- adjacent to the triple bond has a clear and unobstructed peak in the 1H NMR at 4.5 ppm. This peak was used to calculate the amount of functionalisation after the Michael addition by calculating the ratio between the peak and the hydrolysis backbone peak (see Figure S4, ESI). The main advantage of this method is that the degree of hydrolysis does not need to be known and this method is independent of molecular weight. The importance of this will become more apparent when PFAOx is discussed. PE1-PE4 all used 1.5 equivalents of monomer and base with the only difference being to change the reaction time and/or temperature. Carried out at 60 °C for 15 h, PE1 shows a functionalisation of 20%. Conversely, PE2 was reacted at double the temperature for a fraction of the time (2 h) and shows a higher functionalisation of 27% which suggests temperature has an effect. PE2 was then purified and re-reacted with 1.5 equivalents of both base and monomer A and reacted again for 2 h at 120 °C (PE3). As can be seen in the degree of functionalisation increases from 27% to 41%. The effect of leaving the reaction at high temperatures for 15 h was also investigated (PE4) and the functionalisation increased from 27% to 59% going from 2 h to 15 h. Forcing conditions of 140 °C, 5 equivalents of monomer and base for 5 h (PE5) was also investigated, and the functionalisation was found to be 59%, the same as for PE4.
In addition to monomer A, two other monomers were also investigated to look at the substrate scope of these reactions. In the same way as monomer A, for monomer B (hydroxyethyl acrylate) the – CH2- peaks adjacent to the OH could be used to calculate the ratio between monomer B and the hydrolysed backbone peak and therefore the functionalisation. The same conditions were used as PE2 using monomer B (PE6) and the functionalisation was found to be 21% some 6% lower than when using monomer A. This suggests that the nature of the Michael acceptor is a determining factor in the amount of functionalisation, as expected. Moreover, the functionalisation was also measured when a methacrylate (monomer C) was utilised, and the functionalisation found to be significantly lower at 4% (PE7). The drop in functionalisation is likely due to methacrylates being poorer Michael acceptors due to the σ-donation of the methyl group. It is important to note, though, that unlike with monomer A, no clear and unobstructed peak in the 1H NMR exists for monomer B and therefore an alternative calculation was required. In this case, the reduction in the hydrolysis peak was used. This method is less favourable because a 1% difference on the hydrolysis calculation leads to a 10% difference in the functionalisation for a polymer with a hydrolysis level of 10%.
Discussion now turns to PF1-PF4 where PFAOx was used. For these polymers, the starting polymer had a targeted [M]:[I] = 50:1 and a targeted 24% hydrolysis rate formed using the THF/water 8:2 system developed here. The starting polymer was used directly after the hydrolysis with the exception of a precipitation in acetone step. Therefore, the polymer was still the hydrochloride salt, and hence, an elevated amount of TEA was used. As can be seen from , all entries have a similar level of functionalisation suggesting that a ceiling is reached beyond which the functionalisation cannot proceed. It is likely that steric factors and conformation in solution play a role in preventing further functionalisation. Additionally, equivalents of base or monomer were not found to impact the functionalisation. It is worth noting the contrast between PF1 and PE2, whereby similar conditions were employed. PF1 shows significantly more functionalisation which is suggestive of a conformational role given that the steric hindrance in the case of PFAOx is far higher. Alternatively, the sterically hindered chains could encourage hydrolysis on adjacent amides, allowing a more block-type architecture to form lowering steric hindrance and making functionalisation more facile.
Thiol-yne on PEtOx-monomer A conjugate
To further highlight the scope of reaction, a monomer A conjugate, PE4, was reacted in a thiol-yne reaction with thiophenol. A large excess (10 equivalents) with respect to the alkyne group, as calculated using the theoretical molecular weight, with AIBN being used as catalyst (see ). The 1H NMR of PE4 and the crude material after reaction are shown in . The data indicates the complete loss of the singlet peak at 4.65 ppm which is indicative of the – CH2 adjacent to the triple bond. In a thiol-yne 2 thiol additions are possible with one addition leading to the formation of a double bond, with the second addition being significantly faster [Citation46]. In , the 1H NMR before (PE4, red) and after the thiophenol addition (blue) is shown. It provides clear evidence that the thiol-yne reaction proceeded in a quantitative manner with all propargyl groups reacting, as evidenced by the disappearance of the CH2 adjacent to the triple bond (light blue circle). Moreover, the presence of the post-reaction peaks (grey, black and green circles) further confirms that the addition has proceeded in the expected fashion. There is no evidence of double bond formation indicating that a complete bis-addition has occurred.
Figure 3. 1H NMR spectrum of PE4 before and after thiol-yne click reaction (a), and the GPC traces (RI detector) of PE4 before and after reaction with thiophenol (b).

Additionally, the GPC shown in , shows a shift to higher molecular weight consistent with addition. The disappearance of the high molecular weight shoulder in PE4, an artefact from the initial starting polymer, is noted. Having established that the alkyne group is still accessible and reactive to thiols, there are no obvious reasons that copper catalysed azide alkyne cycloadditions cannot also be performed to further functionalise the material potentially using azide terminated polymers.
Conclusion
In conclusion, a universal hydrolysis system suitable for any poly(2-oxazoline) has been developed and the rate of hydrolysis shown to be comparable to the commonly used aqueous systems. The hydrolysis of long chained, hydrophobic poly(2-fatty acid-2-oxazoline) was found to proceed at a vastly reduced rate due to steric hindrance and hydrophobicity. In addition, the aza-Michael addition of propargyl acrylate was also demonstrated with PFAOx and PEtOx reaching 59% and 60% functionalisation, respectively. In addition, 2-hydroxyethyl acrylate was found to proceed in a comparable manner to that of propargyl acrylate whereas 2-(dimethylamino)ethyl methacrylate was observed to have a much lower functionalisation of 4%. Finally, the aza-Michael conjugate of PEtOx and propargyl acrylate was used in a thiol-yne click reaction with the reaction proceeding to completion. The future would include looking into the solution behaviour of partially hydrolysed PFAOx.
Supplemental Material
Download MS Word (301.9 KB)Acknowledgments
This manuscript is submitted for the memory of late Prof. Dr Yusuf Yagci who has been an inspiring and supportive mentor for many young polymer chemists. His excellent scientific contributions and colourful personality will be greatly missed. Infineum UK Ltd. is acknowledged for funding this work. Authors also thank Dr Beatriz Cattoz and Dr. Daniel Phillips for discussions on this study.
Disclosure statement
No potential conflict of interest was reported by the author(s).
Supplementary material
Supplemental data for this article can be accessed online at https://doi.org/10.1080/15685551.2023.2267232.
Data availability statement
FURTHER DATA WILL BE AVAILABLE UPON REQUEST.
References
- Sedlacek O, Monnery BD, Filippov SK, et al. Poly(2-oxazoline)s – are they more advantageous for biomedical applications than other polymers? Macromol Rapid Commun. 2012;33(19):1648–1662. doi: 10.1002/marc.201200453
- Adams N, Schubert US. Poly(2-oxazolines) in biological and biomedical application contexts. Adv Drug Deliv Rev. 2007;59(15):1504–1520. doi: 10.1016/j.addr.2007.08.018
- de la Rosa VR. Poly(2-oxazoline)s as materials for biomedical applications. J Mater Sci Mater Med. 2014;25(5):1211–1225. doi: 10.1007/s10856-013-5034-y
- Schlaad H, Diehl C, Gress A, et al. Poly(2-oxazoline)s as smart bioinspired polymers. Macromol Rapid Commun. 2010;31(6):511–525. doi: 10.1002/marc.200900683
- Glassner M, Vergaelen M, Hoogenboom R. Poly(2-oxazoline)s: a comprehensive overview of polymer structures and their physical properties. Polym Int. 2018;67(1):32–45. doi: 10.1002/pi.5457
- Cesana S, Kurek A, Baur MA, et al. Polymer-Bound thiol groups on Poly(2-oxazoline)s. Macromol Rapid Commun. 2007;28(5):608–615. doi: 10.1002/marc.200600737
- Yilmaz G, Uzunova V, Hartweg M, et al. The effect of linker length on ConA and DC-SIGN binding of S-glucosyl functionalized poly(2-oxazoline)s. Polym Chem. 2018;9(5):611–618. doi: 10.1039/C7PY01939D
- Šrámková P, Zahoranová A, Kroneková Z, et al. Poly(2-oxazoline) hydrogels by photoinduced thiol-ene “click” reaction using different dithiol crosslinkers. J Polym Res. 2017;24(5):82. doi: 10.1007/s10965-017-1237-0
- Dargaville TR, Forster R, Farrugia BL, et al. Poly(2-oxazoline) hydrogel monoliths via thiol-ene coupling. Macromol Rapid Commun. 2012;33(19):1695–1700. doi: 10.1002/marc.201200249
- Luef KP, Petit C, Ottersböck B, et al. UV-mediated thiol-ene click reactions for the synthesis of drug-loadable and degradable gels based on copoly(2-oxazoline)s. Eur Polym J. 2017;88:701–712. doi: 10.1016/j.eurpolymj.2016.08.012
- Drain BA, Becer CR. Eur. Polym J. 2019;119:344–351. doi: 10.1016/j.eurpolymj.2019.07.047
- Cook AB, Peltier R, Zhang J, et al. Hyperbranched poly(ethylenimine cooxazoline) by thiolyne chemistry for non-viral gene delivery: investigating the role of polymer architecture. Polym Chem. 2019;10(10):1202–1212. doi: 10.1039/C8PY01648H
- Rudolph T, Crotty S, Von der Lühe M, et al. Synthesis and solution properties of Double hydrophilic poly(ethylene oxide)-block-poly(2-ethyl-2-oxazoline) (PEO-b-PEtOx) star block copolymers. Polymers. 2013;5(3):1081–1101. doi: 10.3390/polym5031081
- Rudolph T, Crotty S, Schubert US, et al. Star-shaped poly(2-ethyl-2-oxazoline) featuring a porphyrin core: synthesis and metal complexation. e-Polymers. 2015;15(4):227–235. doi: 10.1515/epoly-2015-0041
- Trachsel L, Romio M, Zenobi‐Wong M et al. Hydrogels Generated from Cyclic Poly(2-Oxazoline)s Display Unique Swelling and Mechanical Properties. Macromol Rapid Commun. 2020;42(7):2000658.
- Yan W, Divandari M, Rosenboom J-G, et al. Design and characterization of ultrastable, biopassive and lubricious cyclic poly(2-alkyl-2-oxazoline) brushes. Polym Chem. 2018;9(19):2580–2589. doi: 10.1039/C7PY02137B
- Jung Y, Kim J-H, Jang W-D. Linear and cyclic poly(2-isopropyl-2-oxazoline)s for fine control of thermoresponsiveness. Polym J. 2017;88:605–612. doi: 10.1016/j.eurpolymj.2016.09.003
- Morgese G, Cavalli E, Rosenboom J-G, et al. Cyclic polymer grafts that lubricate and protect damaged cartilage. Chem Int Ed. 2018;57(6):1621–1626. doi: 10.1002/anie.201712534
- Verbraeken B, Hoogenboom R. Cyclic polymers: from scientific curiosity to advanced materials for gene delivery and surface modification. Angewandte Chemie. 2017;56(25):7034–7036. doi: 10.1002/anie.201703418
- Drain BA, Beyer VP, Cattoz B, et al. Solvent dependency in the synthesis of multiblock and cyclic Poly(2-oxazoline)s. Macromolecules. 2021;54(12):5549–5556. doi: 10.1021/acs.macromol.1c00529
- Huettner N, Goldmann AS, Hoogenboom R, et al. Macrocyclization efficiency for poly(2-oxazoline)s and poly(2-oxazine)s. Polym Chem. 2022;13(27):3975–3980. doi: 10.1039/D2PY00376G
- Kim J, Waldron C, Cattoz B, et al. An ε-caprolactone-derived 2-oxazoline inimer for the synthesis of graft copolymers. Polym Chem. 2020;11(42):6847–6852. doi: 10.1039/D0PY01092H
- Beyer VP, Cattoz B, Strong A, et al. Brush copolymers from 2-oxazoline and acrylic monomers via an inimer approach. Macromolecules. 2020;53(8):2950–2958. doi: 10.1021/acs.macromol.0c00243
- Lambermont-Thijs HML, Bonami L, Du Prez FE, et al. Linear poly(alkyl ethylene imine) with varying side chain length: synthesis and physical properties. Polym Chem. 2010;1(5):747–754. doi: 10.1039/b9py00344d
- Lambermont-Thijs HML, Van Der Woerdt FS, Baumgaertel A, et al. Linear poly(ethylene imine)s by acidic hydrolysis of Poly(2-oxazoline)s: kinetic screening, thermal properties, and temperature-induced solubility transitions. Macromolecules. 2010;43(2):927–933. doi: 10.1021/ma9020455
- De La Rosa VR, Bauwens E, Monnery BD, et al. Fast and accurate partial hydrolysis of poly(2-ethyl-2-oxazoline) into tailored linear polyethylenimine copolymers. Polym Chem. 2014;5(17):4957–4964. doi: 10.1039/C4PY00355A
- Akiyama Y, Harada A, Nagasaki Y, et al. Synthesis of poly(ethylene glycol)-block-poly(ethylenimine) possessing an Acetal group at the PEG end. Macromolecules. 2000;33(16):5841–5845. doi: 10.1021/ma000167c
- Mees MA, Hoogenboom R. Full and partial hydrolysis of poly(2-oxazoline)s and the subsequent post-polymerization modification of the resulting polyethylenimine (co)polymers. Polym Chem. 2018;9(40):4968–4978. doi: 10.1039/C8PY00978C
- Van Kuringen HPC, Lenoir J, Adriaens E, et al. Partial hydrolysis of Poly(2-ethyl-2-oxazoline) and potential implications for biomedical applications? Macromol biosci. 2012;12(8):1114–1123. doi: 10.1002/mabi.201200080
- Haladjova E, Smolíček M, Ugrinova I, et al. DNA delivery systems based on copolymers of poly (2-methyl-2-oxazoline) and polyethyleneimine: effect of polyoxazoline moieties on the endo-lysosomal escape. J Appl Polym Sci. 2020;137(45):49400. doi: 10.1002/app.49400
- Jeong JH, Song SH, Lim DW, et al. DNA transfection using linear poly(ethylenimine) prepared by controlled acid hydrolysis of poly(2-ethyl-2-oxazoline). J Control Release. 2001;73(2–3):391–399. doi: 10.1016/S0168-3659(01)00310-8
- Haladjova E, Halacheva S, Momekova D, et al. Polyplex particles based on comb-like Polyethylenimine/Poly(2-ethyl-2-oxazoline) copolymers: relating biological performance with morphology and structure. Macromol biosci. 2018;18(4):1700349. doi: 10.1002/mabi.201700349
- Bauer M, Tauhardt L, Lambermont-Thijs HML, et al. Rethinking the impact of the protonable amine density on cationic polymers for gene delivery: a comparative study of partially hydrolyzed poly(2-ethyl-2-oxazoline)s and linear poly(ethylene imine)s. Eur J Pharm Biopharm. 2018;133:112–121. doi: 10.1016/j.ejpb.2018.10.003
- Cortez MA, Godbey WT, Fang Y, et al. The synthesis of Cyclic poly(ethylene imine) and exact linear analogues: an evaluation of gene delivery comparing polymer architectures. J Am Chem Soc. 2015;137(20):6541–6549. doi: 10.1021/jacs.5b00980
- Halacheva S, Price GJ, Garamus VM. Effects of temperature and polymer composition upon the aqueous solution properties of comblike linear poly(ethylene imine)/Poly(2-ethyl-2-oxazoline)-based polymers. Macromolecules. 2011;44(18):7394–7404. doi: 10.1021/ma2012859
- van Kuringen HPC, de la Rosa VR, Fijten MWM, et al. Enhanced selectivity for the hydrolysis of block Copoly(2-oxazoline)s in ethanol–water resulting in linear poly(ethylene imine) copolymers. Macromol Rapid Commun. 2012;33(9):827–832. doi: 10.1002/marc.201200046
- Lambermont-Thijs HML, Heuts JPA, Hoeppener S, et al. Selective partial hydrolysis of amphiphilic copoly(2-oxazoline)s as basis for temperature and pH responsive micelles. Polym Chem. 2011;2(2):313–322. doi: 10.1039/C0PY00052C
- Toncheva-Moncheva N, Veleva-Kostadinova E, Tsvetanov C, et al. Preparation and properties of positively charged mesoglobules based on poly(2-isopropyl-2-oxazoline) and evaluation of their potential as carriers of polynucleotides. Polymer. 2017;111:156–167. doi: 10.1016/j.polymer.2017.01.049
- Mees M, Haladjova E, Momekova D, et al. Partially hydrolyzed poly (n-propyl-2-oxazoline): synthesis, aqueous solution properties, and preparation of gene delivery systems. Biomacromolecules. 2016;17(11):3580–3590. doi: 10.1021/acs.biomac.6b01088
- Sedlacek O, Monnery BD, Hoogenboom R. Synthesis of defined high molar mass poly(2-methyl-2-oxazoline). Polym Chem. 2019;10(11):1286–1290. doi: 10.1039/C9PY00013E
- Sedlacek O, Bera D, Hoogenboom R. Poly(2-amino-2-oxazoline)s: a new class of thermoresponsive polymers. Polym Chem. 2019;10(34):4683–4689. doi: 10.1039/C9PY00943D
- Sedlacek O, Janouskova O, Verbraeken B, et al. Straightforward route to superhydrophilic Poly(2-oxazoline)s via acylation of well-defined polyethylenimine. Biomacromolecules. 2019;20(1):222–230. doi: 10.1021/acs.biomac.8b01366
- Mees MA, Effenberg C, Appelhans D, et al. Sweet polymers: Poly(2-ethyl-2-oxazoline) glycopolymers by Reductive Amination. Biomacromolecules. 2016;17(12):4027–4036. doi: 10.1021/acs.biomac.6b01451
- Bertrand E, Gonalves C, Billiet L, et al. Histidinylated linear PEI: a new efficient non-toxic polymer for gene transfer. Chem Commun. 2011;47(46):12547–12549. doi: 10.1039/c1cc15716g
- de la Rosa VR, Bauwens E, Monnery BD, et al. Fast and accurate partial hydrolysis of poly(2-ethyl-2-oxazoline) into tailored linear polyethylenimine copolymers. Polym Chem. 2014;5(17):4957–4964. doi: 10.1039/C4PY00355A
- Lowe AB, Hoyle CE, Bowman CN. Thiol-yne click chemistry: a powerful and versatile methodology for materials synthesis. J Mater Chem. 2010;20(23):4745–4750. doi: 10.1039/b917102a