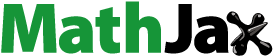
Abstract
Excessive use of fossil fuels has caused their rapid depletion, resulting in a growing energy crisis as well as many environmental concerns, and prompting the development of the sustainable energy conversion system. In this study, we report GO based SnSe nanomaterial incorporated via the hydrothermal route engaging OER activity. The morphological, structural and textual effects of the material have been measured with scanning electron microscopy (SEM), X-ray diffraction (XRD) and Brunauer Emmett Teller (BET). Furthermore, the kinetic process, active sites, conductivity and stability of the electrode medium of GO, SnSe and GO/SnSe were estimated with electrochemical impedance spectroscopy (EIS), linear sweep voltammetry (LSV), electrochemical active surface area (ECSA) and chronoamperometry under 1.0 M KOH with Ni foam as a conductive substrate. Its electrochemical results depicted the following: the GO based SnSe nanocomposite displayed lesser overpotential around 336 mV to reach a current density around 10 mA cm−2 and Tafel slope of 36 mV dec−1. Cyclic stability and chronoamperometry analysis of GO/SnSe nanocomposite show stability over 35 h. The exceptional electrochemical properties of the GO/SnSe nanocomposite differentiate it as a good material for use in electrical and in many other fields in future.
1. Introduction
Nonrenewable energy resources like natural gas, oil and coal have been utilized as the principal source of energy to sustain human activities since the eighteenth century [Citation1]. Our civilization has been pushed to establish a green and sustainable energy system for long-term growth as a result of rapidly rising global population and expanding industrialization, as well as the attendant environmental issues [Citation2]. Solar, wind and tidal energy are the examples of renewable and carbon-free energy sources that are used all over the world [Citation3,Citation4]. Unfortunately, these energy sources are only available during specific seasons and weather conditions, resulting in an inefficient energy distribution and utilization [Citation5–9].
However, the researchers have successfully replaced the polluted and season-dependent energy sources with a sustainable, clean, economical and efficient hydrogen source of energy [Citation10]. Therefore, several techniques such as steam reforming [Citation11], gasification [Citation12], chemical oxidation [Citation13] and water splitting [Citation14] are some of the ways for creating hydrogen energy. Among all, water spitting is the best source to produce renewable energy (H2 and O2) a suitable alternative for reducing dependency on fossil fuels, satisfying the growing energy demand, and fulfilling the safe environmental criteria due to the clean nature of H2 as a fuel [Citation15–17]. OER (oxygen evolution reaction) at the anode (2H2O(l) O2(g) + 4H + (aq) + 4e−) and HER (hydrogen evolution reaction) at the cathode (2H + (aq) 2e− H2(g)) are two half-reactions intricate in electrochemical water splitting [Citation18]. Furthermore, due to thermodynamic and kinetic restrictions, reaching 100% Faradaic efficiency is extremely difficult [Citation19–21].
Modern OER electrocatalysts are often constructed by using valuable metals like Pt, Ru and Os which have limited availability and high cost, which restrict their application [Citation22]. Owing to their affordability, abundant availability, ecological sensitivity and considerable electrocatalytic performance, transition metal based materials have recently been widely explored for OER [Citation23]. The remarkable physical, chemical and electrical features of transition metal selenides (TMS) composites have piqued interest in their potential use for energy storage [Citation24,Citation25]. Metal selenides exhibit more electrochemical activity in reactions than metal oxides/hydroxides because selenium has a lower electronegativity than oxides and sulphur, resulting in a weaker chemical link between the atom and the binding electrons. Several transition metal selenides such as including MoSe2 [Citation26], CoSe [Citation27], Co0.85Se [Citation27], SnSe2 [Citation28], Ni3Se2 [Citation29] and Cu2Se [Citation30] have been reported. To improve specific capacitance and durability, SnSe is integrated into the carbon-based compound particularly graphene, which increases charge transfer capacity at the electrode/electrolyte interface and hence electrical conductivity [Citation31]. Excellent thermal conductivity, strong mechanical properties, outstanding electrical conductivity, significant chemical stability, high specific surface area and fast electromobility are some of the characteristics of graphene [Citation32–36]. On the other hand, the strong Vander Walls contact between graphene layers may promote the aggregation of two-dimensional (2D) graphene sheets, reducing the active surface area and changing other features. Due to its unique structure, three-dimensional (3D) graphene may avoid interaction [Citation37–42]. It has been demonstrated that 3D graphene has a greater response sensitivity than 2D graphene. Because of its excellent conductivity and efficient electron transport channels, 3D graphene is employed as a communication platform to improve electron transmission and transit in HER and OER processes [Citation43–46]. Additionally, Renuka et al. synthesized the graphene oxide based Cu2ZnS4 nanocomposite for OER. The electrochemical result of the GO/Cu2ZnS4 material exhibited Tafel slopes of 91 mV dec−1 to reach a current density around 10 mA cm−2 and overpotential of 1.36 V [Citation47]. Pratik Shinde et al. synthesized the NiFe2O4/rGO nanocomposite for OER and electrochemical result of the NiFe2O4 material indicated a Tafel slope of 63 mV dec−1 and an overpotential of 302 mV to attain a current density of 10 mA cm−2 [Citation48]. Jahangeer Ahmed et al. developed the NiMoO4-nanorods supported on graphene oxide via a hydrothermal route. The NiMoO4/GO material exhibited an overpotential of 186 mV and Tafel slope of 54 mV dec−1 [Citation49]. Nagarani Sandhiran et al. synthesized CuO–NiO/rGO nanocomposites via the co precipitation route employed for the water splitting process. However, material oxide exhibited the Tafel slope of 72 mV dec−1 and onset potential of 0.8 mV at a current density of 29 mA cm−2 [Citation50]. Jiemei et al. investigated the electrochemical performance of CuNiO/rGO under alkaline solution reported the starting potential at 2.08 mA cm−2 and 121 mV dec−1 [Citation50]. Thus, electrochemical finding suggests that transition metal-based chalcogenides are considered as a good replacement with noble metal and show outstanding OER activity by substituting the electron donating group like graphene material.
In this research, we report the manufacturing of the GO/SnSe nanocomposite via the facile hydrothermal route employed towards the OER activity. The structural, morphological and textual analyses of the material were confirmed with scanning electron microscopy (SEM), X-ray diffraction (XRD) and Brunauer Emmett Teller (BET) analysis, correspondingly. Furthermore, the electrochemical production of electrocatalyst had been evaluated with various electrochemical parameters. Electrochemical findings imply GO based SnSe nanocomposite exhibited less overpotential around 336 mV in attaining current density of 10 mA cm−2 and Tafel slope of 36 mV dec−1. This finding not only explains the importance of OER using the synthesized material as electrocatalyst but also showed by research to improve water splitting through different approaches and structural modification.
2. Experimental section
2.1 Material
The precursors used for the fabrication of GO/SnSe were selenium powder (Sigma Aldrich, 99%), hydrazine monohydrate (Sigma Aldrich, 99.0%), graphite powder (Sigma Aldrich, 99%), tin chloride (SnCl2, Sigma Aldrich, 99%), potassium permanganate (KMnO4, Merck, 99%), phosphoric acid (H3PO4, Merck, 85%), hydrochloric acid (HCl, AnalaR, 37%), sulphuric acid (H2SO4, AnalaR, 98%) and deionized water.
2.2. Fabrication of graphene oxide (GO)
The graphene oxide (GO) nanosheet was prepared via simple Hummer’s process. For the fabrication of GO, 5 g graphite powder, 2.5 g NaNO3, 12 mL H3PO4 and100 mL of H2SO4 were added into a beaker and mixed under vigorous stirring, then comes the gradual accumulation of 15 g of KMnO4 in aforementioned combination. However, the temperature of the reaction vessel was decreased by placing it in an ice bath. After half an hour, the reaction mixture became thick, so 300 mL of deionized water was added and agitated for 20 min. After that around 500 mL water and 50 mL of H2O2 were added, then the mixture was centrifuged followed by washing finally with deionized water and 5% HCl to eliminate any residue from the suspension and subsequently the prepared material was dehydrated for one day at 60 °C in an electric oven [Citation51].
2.3 Fabrication of GO/SnSe via the chemical reduction technique
For the synthesis of SnSe, 0.1 mM SnCl2 and 0.1 mM Se powders were dissolved in 10 mL of 1 M KOH aqueous solution. After that 2 mL of N2H4 was added in the aforementioned mixture involving vigorous stirring for 1 h at ambient reaction conditions. The resultant mixture was transformed into the Teflon-lined autoclave for 4 h at 180 °C. The resulting product was removed by centrifugation and then washed multiple times with deionized water along with ethanol. This obtained product was then dried around 60 °C and stored for further use. Furthermore, for the fabrication of the GO/SnSe composite, the same procedure was adopted except 0.5 g of the already fabricated GO was added into the prepared mixture which was used for the synthesis of SnSe. The synthesized SnSe and GO/SnSe nanocomposite were saved for further chemical and electrochemical characterization.
2.4 Electrode preparation
To produce the working electrode (WE), firstly, nickel foam (NF) was fragmented into tiny bits (1*1 cm) and activated by sonicating with ethanol, acetone and ultrapure deionized water 15 min for each step. After that NF was dried in an electric oven for 15 min at 50 °C. Additionally, the catalytic ink was fabricated with 10 mg of the electrocatalytic powder in 80 mL of deionized water and then sonicated for 20 min. The resulting catalytic ink was pasted on the Ni foam by a simple drop casting approach and this was dryed at room temperature for further process.
2.5 Physical characterization
A Bruker 2 D X-ray diffractometer equipped with Ni filtered Cu Kα (1.54178 Å) radiation was employed for the determination of the structure and crystalline phases for the fabricated material. The Quanta 200-FEG SEM combined with energy-dispersive x-ray (EDX) at an accelerating voltage of 100 kV was used; its morphology, microstructure, and elemental content of a synthesized sample were determined using a scanning electron microscope (SEM-EDX). Furthermore, the Nova2200e Quantachrome Brunauer Emmett Teller was investigated for textual properties of the synthesized material.
2.6 Electrochemical characterization
Electrochemical activity of the fabricated electrocatalyst was investigated with Metrohm Autolab (PGSTAT-204) armed via the 3 electrode system such as the Ag/AgCl function reference electrode, Pt wire function as counter and fabricated material dumped on the NF function as WE under 1.0 M alkaline KOH solution. OER performance was calculated via LSV, CV, EIS, ECSA and chronoamperometry by 2.1 NOVA software. However, recorded potentials were transformed to RHE with the following equation [Citation52]:
(1)
(1)
The CV polarization curve was used to measure the electrocatalytic activity and kinetics via the Tafel slope considering linear region of the steady state curve with equation [Citation53].
(2)
(2)
The electrochemical surface area was computed by polarization curves at different sweep speeds in capacitive areas within the potential window that goes from 0.90 to 1.20 V vs RHE. The electrochemical surface area of the fabricated material was calculated with the following equation [Citation54]:
(3)
(3)
Electrochemical impedance spectroscopy was used to measure electrical conductivity of the material having a frequency region of 0.1–100,000 Hz. Moreover, the Nyquist plot was employed for the determination of reluctance to solution Rs and charge transfer resistance Rct of the manufactured electrode material by fitting along the Randles circuit. Also, the firmness of the fabricated electrode material was investigated via the chronoamperometry method at a fixed potential of 1.75 V vs. RHE.
3. Result and discussion
3.1 Structural, morphology and textual properties.
Crystal structure, purity, crystallinity and size of the prepared substance were measured by XRD in 2-theta ranging from 0 o to 80o. (b) shows the peak at 25.19 °, 30.47 °, 31.04 °, 37.61 °, 47.96° correspond to 012, 111, 004, 113 and 115 indexed well to JCPDS card no. 00-032-1382 and exhibited the orthorhombic crystal system having a = 11.420, b = 4.190, c = 4.460 Å cell constant with the Pnma space group. As shown in , the distinctive broad peak appearing at 7.24° is attributed to 001 hkl value, confirming the synthesis of GO. Furthermore, the existence of both phases GO and SnSe peak observed in the diffractogram of GO/SnSe with diffraction peaks at 2θ = 6.45, 24.44, 30.47, 37.34, 49.65° indicates the successful synthesis of the GO/SnSe nanocomposite. The slight shifting in the XRD pattern of the composite occurred due to the synergetic effect of the GO and SnSe than their pristine material. The crystallinity and purity of the material were determined by the appearance of sharp peaks and the presence of the number of other peak depicts its crystalline nature and purity of the synthesized material [Citation55]. Crystallite size of the material was measured with Debye Scherrer equation (4) and is in range of 86, 72 and 59 nm for GO, SnSe and nanocomposite, respectively [Citation56–63].
(4)
(4)
Raman spectra of SnSe, GO, and the GO/SnSe composite were acquired to confirm the presence of GO in the composite and gain an insight into the phases and orientation of the band in the fabricated materials ((e)). The two spectral peaks at 159.99 and 185.32 cm−1 are associated with the B-plane vibrations related to SnSe that are typical of the Ag phonon modes. The peak at 114.25 cm−1 in SnSe may be explained by the B3g phonon mode, which has been produced by stiff shear modes in c directions. GO demonstrates a spectrum that includes a significant carbon D (1356 cm−1) and G (1610 cm−1) bands, which have been correlated with vibrations of sp3-type carbon atoms (typically activated carbon along with disordered) and sp2-like carbon atoms (typical of graphitic layers), while the formation of the GO/SnSe nanosized composite leads to higher intensity of D as well as G band and other two bands such as D + G and 2D appeared at 2560.10 and 2753.11 cm−1, respectively, are related to the carbon-based materials. With the exception of a very little but detectable red shift, which may reflect an interaction between SnSe and GO, all peaks are likewise seen in the GO/SnSe spectra confirming the successful synthesis of the nanocomposite.
The microstructure and morphology of material were measured with SEM. (a) depicts thick dendrite layers of GO, and these dendrites promoted electrochemical performance because of the enhanced surface area. However, the SnSe exhibited a smooth surface with randomly distributed nanorod morphology, which provides an extensive surface area as well as active sites for enhanced electrochemical performance as shown in (b). (c) denotes the GO/SnSe nanocomposite morphology showing the nanorod anchoring on the dendrite layers of graphene oxide because the rod-shaped SnSe was uniformly engaged between the stacked graphene layers which are accountable for the creation of restriction between the restacking of graphene layers during the electrochemical performance of the GO/SnSe nanocomposite, and also the synergetic effect provides a large surface area which is evidence of good electrochemical surface area (ECSA) analysis. The elemental configuration of the designed material was analysed using the EDX study. As depicted in (d), the occurrence of C, O in GO, and the SnSe EDX spectrum contains Sn and Se elements in the sample as depicted in (e), while on the other hand, in (f) the GO/SnSe nanocomposite contains C, O, Sn, Se, in the relative EDX spectra. However, the existence of no extra peak shows the purity of the material and shows excellent consistency between the XRD results
Figure 2. (a) SEM micrograph of GO, (b) SnSe, (c) GO/SnSe, (d–f) EDX spectrum of GO, SnSe, and GO/SnSe, (g) BET isotherm of GO, SnSe and GO/SnSe, and pore size distribution in the inset.

The surface area of GO, SnSe and GO/SnSe powders was determined using the BET method, which established an adsorption–desorption isotherm. (g) shows type III isotherms, indicating that the adsorbate and adsorbent have limited interaction. The absence of an adsorption–desorption hysteresis loop in the isotherm rules out the GO and SnSe powders are mesoporous [Citation64]. The isotherm, on the other hand, demonstrated multilayer gas adsorption, with GO, SnSe and GO/SnSe having specific BET surface areas of 66, 160, 249 m2 g−1, respectively. The pore size distribution of GO, SnSe as well as GO/SnSe was measured with desorption data from the Barret−Joyner−Halenda (BJH) model, as seen in (g) inset. Calculated pore volumes for GO, SnSe and GO/SnSe are 0.1, 0.9, as well as 1.1 cm3 g−1, accordingly. Thus, enhanced pore volume and greater surface area of the GO/SnSe are attributed to the existence of nanorods supported on the graphene oxide sheet increase surface area and the number of active site accountable to robust OER activity.
4. Electrochemical study
Cyclic voltammetry was used to assess the electrochemical performance of the produced material coated on NF under 1.0 M alkaline KOH at a sweep speed of 5 mV s−1. The cyclic voltammogram profile shows the peroxidative characteristics at 1.6 V due to the oxidation of Sn + 2 to Sn + 3, followed by the steady decline at 1.4 V vs. RHE suggesting start of the OER process with the evolution of oxygen bubble at the surface of the working electrode as shown in (a). Furthermore, the intrinsic electrocatalytic property of fabricated substances was investigated along with LSV at a sweep speed of 5 mV s−1 as shown in (b). Also, overpotential and Tafel slope of GO, SnSe, RuO2 and GO/SnSe 355, 431, 375 and 336 mV, and 69, 96, 80 and 36 mV dec−1, respectively, measured via Equation Equation(2)(2)
(2) as depicted in (c,d) and comparison between the reported literature and fabricated materials are given in . Among all GO/SnSe had the least overpotential of 336 mV dec−1 to attain a current density around 10 mA cm−2, as well as the lower value of the Tafel slope (36 mV dec−1) of the nanocomposite in contrast to the benchmark of RuO2 and other fabricated substances suggest the rate determining step, easy electron transfer process and the kinetic mechanism of the fabricated substance which are given below
(5)
(5)
(6)
(6)
(7)
(7)
(8)
(8)
(9)
(9)
Figure 3. (a) CV curves, (b) LSV curves at a sweep speed of 5 mV s−1, (c) comparison of overpotential to reach current density of 10 mA cm−2 of all the fabricated catalysts, and (d) Tafel slope of the GO, SnSe, RuO2 and GO/SnSe nanocomposite

Table 1. Comparison of OER performance with reported literatures and fabricated materials.
During the OER process, OH− was adsorbed on the surface of a free metal ion, resulting in the production of MOH, as well as deprotonation transform of M–OH to M–O. There are two ways to create O2 molecules, and then, M–O interacts with OH− to produce M–OOH, which deprotonates with active site renewal to form O2 [Citation65].
Electrochemical surface area of the material was determined with double layer capacitance in potential window ranging from 0.80–1.10 V vs. RHE at sweep speed of 5, 10 and 15 mV s−1 in 1.0 M alkaline KOH as shown in (a–c), and difference between anodic as well as cathodic current density as a function of scan rate is mentioned in (d–f), and the calculated Cdl values of GO, SnSe, and GO/SnSe are 11.38, 4.105 and 17.3 mF, respectively, using Equation Equation(3)(3)
(3) and (d–f). A larger Cdl value of the GO/SnSe suggests that the material exhibited high rate of charge accumulation and larger surface area with enhanced electrochemical active sites [Citation75].
The solution resistance (Rs) and charge transfer resistance (Rct) of the fabricated electrode substance were calculated with EIS under 1.0 M alkaline KOH at 0.50 V vs Ag/AgCl electrode. The Nyquist plots displayed a smaller semicircle resultant via the circuit fitted by employing Nova 2.1 software. The electrochemical result revealed that GO, SnSe and GO/SnSe exhibited the Rct value of 0.2, 0.8, and 2.6 Ω, respectively. The smaller Rct value of GO/SnSe is attributed to their crystalline structure, good morphology, larger surface area, greater amounts of active sites and increased electrical conductivity in the alkaline medium [Citation76].
Long-term stability of synthesized materials was confirmed via the chronoamperometry technique and reported as an outcome of potential vs time for 35 h ((b)). Moreover, the cyclic stability of the substance was also measured with multiple CV cycles as depicted in (c). Electrochemical stability results suggested that the material shows stable behaviour over 2000 cycle with negligible change, corroborative with the high stability of the resultant substance. Hence, the increased electrochemical performance of the fabricated GO/SnSe electrode material was because of the inclusion of rGO in the composite, which not only gave the physical support, avoiding volume expansion and electrode material aggregation, but it also allowed for rapid electron flow during the electrochemical process, high surface area, good morphology and high crystallinity of the resultant material. To confirm the structure, phase retaining the XRD was also performed after the stability test and is shown in (d). The resultant XRD peaks’ position approves that there is no prominent change in the phase, but smaller change in the intensity of the peaks occurs.
5. Conclusion
In this summary, we report the fabrication of the GO/SnSe nanocomposite via the hydrothermal route employed for the water splitting process. The various analytical characterizations are often utilized to examine the morphology, structure and textual characteristics of the fabricated material. Further, the electrochemical performance of the substance was measured in 1.0 M alkaline KOH solution, and electrochemical result suggests that the GO based SnSe exhibited lesser overpotential of 336 mV to attain a current density around 10 mA cm−2 as well as smaller Tafel slope of 36 mV dec−1. However, the electrochemical efficiency of the GO/SnSe nanocomposite was further confirmed with EIS and chronoamperometry analysis, indicating good conductivity due to enhanced larger surface area supported by the rod-like structure and stable for 35 h. Hence, our electrochemical results indicate that GO-based SnSe combinations are highly efficient for OER activity, and also opens a new era for different applications.
Acknowledgement
The authors express their gratitude to Princess Nourah bint Abdulrahman University Researchers Supporting Project number (PNURSP2023R26), Princess Nourah bint Abdulrahman University, Riyadh, Saudi Arabia.
Disclosure statement
No potential conflict of interest was reported by the author(s).
References
- Poizot P, Dolhem F. Clean energy new deal for a sustainable world: from non-CO2 generating energy sources to greener electrochemical storage devices. Energy Environ Sci. 2011;4:2003–2019. doi:10.1039/C0EE00731E.
- Zhironkin S, Cehlár M. Green economy and sustainable development: the outlook. Energies. 2022;15:1167. doi:10.3390/EN15031167.
- Manjunatha C, Lakshmikant S, Shreenivasa L, et al. Development of non-stoichiometric hybrid Co3S4/Co0.85Se nanocomposites for an evaluation of synergistic effect on the OER performance. Surf Interf. 2021;25:101161. doi:10.1016/J.SURFIN.2021.101161.
- Gaur A, Krishankant, Pundir V, et al. Intense nano-interfacial interactivity stimulates the OER in a MOF-derived superhydrophilic CuO–NiO heterostructure, sustain. Energy Fuels. 2021;5:5505–5512. doi:10.1039/D1SE01235E.
- Li J, Chu D, Baker DR, et al. Earth-abundant Fe and Ni dually doped Co2P for superior oxygen evolution reactivity and as a bifunctional electrocatalyst toward renewable energy-powered overall alkaline water splitting. ACS Appl Energy Mater. 2021;4:9969–9981. doi:10.1021/ACSAEM.1C01926/SUPPL_FILE/AE1C01926_SI_004.MP4.
- Burton AR, Paudel R, Matthews B, et al. Thickness dependent OER electrocatalysis of epitaxial LaFeO3 thin films. J Mater Chem A. 2022;10:1909–1918. doi:10.1039/D1TA07142D.
- Agarwal S, Yu X, Manthiram A. A pair of metal organic framework (MOF)-derived oxygen reduction reaction (ORR) and oxygen evolution reaction (OER) catalysts for zinc-air batteries. Mater Today Energy. 2020;16:100405. doi:10.1016/J.MTENER.2020.100405.
- Keerthana SP, Rani BJ, Ravi G, et al. Ni doped Bi2WO6 for electrochemical OER activity. Int J Hydrogen Energy. 2020;45:18859–18866. doi:10.1016/J.IJHYDENE.2020.05.135.
- S YRT, S N, A L, et al. Characterization and OER performance of CrVO 4 nanoparticles. ECS Trans. 2022;107:14335–14342. doi:10.1149/10701.14335ECST.
- Bayro-Kaiser V, Nelson N. Microalgal hydrogen production: prospects of an essential technology for a clean and sustainable energy economy. Photosynth Res. 2017;133:49–62. doi:10.1007/S11120-017-0350-6/TABLES/1.
- Mao X, Li WZ, Yuan Y, et al. Numerical analysis of methanol steam reforming reactor heated by catalytic combustion for hydrogen production. Int J Hydrogen Energy. 2022;47:14469–14482. doi:10.1016/J.IJHYDENE.2022.02.221.
- Zhang S, He S, Gao N, et al. Hydrogen production from autothermal CO2 gasification of cellulose in a fixed-bed reactor: influence of thermal compensation from CaO carbonation. Int J Hydrogen Energy. 2022. doi:10.1016/J.IJHYDENE.2022.02.018.
- Tayyab M, Liu Y, Min S, et al. Simultaneous hydrogen production with the selective oxidation of benzyl alcohol to benzaldehyde by a noble-metal-free photocatalyst VC/CdS nanowires. Chinese J Catal. 2022;43:1165–1175. doi:10.1016/S1872-2067(21)63997-9.
- Chen S, Qiao SZ. Hierarchically porous nitrogen-doped graphene-NiCo2O4 hybrid paper as an advanced electrocatalytic water-splitting material. ACS Nano. 2013;7:10190–10196. doi:10.1021/NN404444R/SUPPL_FILE/NN404444R_SI_001.PDF.
- Zhu S, ai Huang L, He Z, et al. Investigation of oxygen vacancies in Fe2O3/CoOx composite films for boosting electrocatalytic oxygen evolution performance stably. J Electroanal Chem. 2018;827:42–50. doi:10.1016/j.jelechem.2018.09.011.
- Wu LK, Hu JM. A silica co-electrodeposition route to nanoporous Co3O4 film electrode for oxygen evolution reaction. Electrochim Acta. 2014;116:158–163. doi:10.1016/j.electacta.2013.11.010.
- Zhang L, Li H, Yang B, et al. Promote the electrocatalysis activity of amorphous FeOOH to oxygen evolution reaction by coupling with ZnO nanorod array. J Solid State Electrochem. 2020;24:905–914. doi:10.1007/S10008-020-04540-2/FIGURES/7.
- Tomboc GM, Kim T, Jung S, et al. Modulating the local coordination environment of single-atom catalysts for enhanced catalytic performance in hydrogen/oxygen evolution reaction. Small. 2022;18:2105680. doi:10.1002/SMLL.202105680.
- Liu Y, Wang Y, Zhao S, et al. Metal–organic framework-based nanomaterials for electrocatalytic oxygen evolution. Small Methods. 2022;6:2200773. doi:10.1002/SMTD.202200773.
- Zhao S, Tan C, He CT, et al. Structural transformation of highly active metal–organic framework electrocatalysts during the oxygen evolution reaction, Nat. Energy. 2020;511(5):881–890. doi:10.1038/s41560-020-00709-1.
- Wang Z, Huang J, Wang L, et al. Cation-tuning induced d-band center modulation on Co-based spinel oxide for oxygen reduction/evolution reaction. Angew Chemie Int Ed. 2022;61:e202114696. doi:10.1002/ANIE.202114696.
- Xu N, Zhang Y, Zhang T, et al. Efficient quantum dots anchored nanocomposite for highly active ORR/OER electrocatalyst of advanced metal-air batteries. Nano Energy. 2019;57:176–185. doi:10.1016/J.NANOEN.2018.12.017.
- Zhao J, Zhang JJ, Li ZY, et al. Recent progress on NiFe-based electrocatalysts for the oxygen evolution reaction. Small. 2020;16:2003916. doi:10.1002/SMLL.202003916.
- Song H, Yu J, Tang Z, et al. Halogen-doped carbon dots on amorphous cobalt phosphide as robust electrocatalysts for overall water splitting. Adv Energy Mater. 2022;12:2102573. doi:10.1002/AENM.202102573.
- Song H, Wu M, Tang Z, et al. Single atom ruthenium-doped CoP/CDs nanosheets via splicing of carbon-dots for robust hydrogen production. Angew Chemie Int Ed. 2021;60:7234–7244. doi:10.1002/ANIE.202017102.
- Shen Q, Jiang P, He H, et al., Encapsulation of MoSe 2 in carbon fibers as anodes for potassium ion batteries and nonaqueous battery–supercapacitor hybrid devices. Pubs.Rsc.Org. (n.d.). 2019. https://pubs.rsc.org/en/content/articlehtml/2019/nr/c9nr03480c (accessed September 27, 2022).
- Zhu Y, Huang Z, Hu Z, et al. 3D interconnected ultrathin cobalt selenide nanosheets as cathode materials for hybrid supercapacitors. Electrochim Acta. 2018;269:30–37. doi:10.1016/J.ELECTACTA.2018.02.146.
- Zhang C, Yin H, Han M, et al. Two-dimensional tin selenide nanostructures for flexible all-solid-state supercapacitors. ACS Nano. 2014;8:3761–3770. doi:10.1021/NN5004315/SUPPL_FILE/NN5004315_SI_001.PDF.
- Shi X, Wang H, Kannan P, et al. Rich-grain-boundary of Ni3Se2 nanowire arrays as multifunctional electrode for electrochemical energy storage and conversion applications. J Mater Chem A. 2019;7:3344–3352. doi:10.1039/C8TA10912E.
- Xu P, Wang G, Miao C, et al. Controllable one-pot synthesis of emerging β-Cu2Se nanowire freely standing on nickel foam for high electrochemical energy storage performance. Appl Surf Sci. 2019;463:82–90. doi:10.1016/J.APSUSC.2018.08.147.
- Jiao Y, Zheng Y, Davey K, et al. Activity origin and catalyst design principles for electrocatalytic hydrogen evolution on heteroatom-doped graphene. Nat Energy. 2016;110(1):1–9. doi:10.1038/nenergy.2016.130.
- Ibrahim EMM, Abdel-Rahman LH, Abu-Dief AM, et al. Electric, thermoelectric and magnetic characterization of γ-Fe2O3 and Co3O4 nanoparticles synthesized by facile thermal decomposition of metal-Schiff base complexes. Mater Res Bull. 2018;99:103–108. doi:10.1016/J.MATERRESBULL.2017.11.002.
- Ibrahim EMM, Abdel-Rahman LH, Abu-Dief AM, et al. The synthesis of CuO and NiO nanoparticles by facile thermal decomposition of metal-Schiff base complexes and an examination of their electric, thermoelectric and magnetic properties. Mater Res Bull. 2018;107:492–497. doi:10.1016/J.MATERRESBULL.2018.08.020.
- Ibrahim EMM, Abu-Dief AM, Elshafaie A, et al. Electrical, thermoelectrical and magnetic properties of approximately 20-nm Ni-Co-O nanoparticles and investigation of their conduction phenomena. Mater Chem Phys. 2017;192:41–47. doi:10.1016/J.MATCHEMPHYS.2017.01.054.
- Abdel Rahman LH, Abu-Dief AM, El-Khatib RM, et al. Sonochemical synthesis, structural inspection and semiconductor behavior of three new nano sized Cu(II), Co(II) and Ni(II) chelates based on tri-dentate NOO imine ligand as precursors for metal oxides. Appl Organomet Chem. 2018;32:e4174), doi:10.1002/AOC.4174.
- Yang A, Li T, Jiang S, et al. High-density growth of ultrafine PdIr nanowires on graphene: reducing the graphene wrinkles and serving as efficient bifunctional electrocatalysts for water splitting. Nanoscale. 2019;11:14561–14568. doi:10.1039/C9NR03027A.
- Wang B, Chen Y, Wang X, et al. rGO wrapped trimetallic sulfide nanowires as an efficient bifunctional catalyst for electrocatalytic oxygen evolution and photocatalytic organic degradation. J Mater Chem A. 2020;8:13558–13571. doi:10.1039/D0TA04383D.
- Yang D, Su Z, Chen Y, et al. Double-shelled hollow bimetallic phosphide nanospheres anchored on nitrogen-doped graphene for boosting water electrolysis. J Mater Chem A. 2020;8:22222–22229. doi:10.1039/D0TA06766K.
- Lu Y, Chen Y, Srinivas K, et al. Employing dual-ligand co-coordination compound to construct nanorod-like Bi-metallic (Fe, Co)P decorated with nitrogen-doped graphene for electrocatalytic overall water splitting. Electrochim Acta. 2020;350:136338), doi:10.1016/J.ELECTACTA.2020.136338.
- Zhou J, Wang Z, Yang D, et al. NiSe2-anchored N, S-doped graphene/Ni foam as a free-standing bifunctional electrocatalyst for efficient water splitting. Nanoscale. 2020;12:9866–9872. doi:10.1039/D0NR00879F.
- Wang X, Zheng B, Wang B, et al. Hierarchical MoSe2-CoSe2 nanotubes anchored on graphene nanosheets: A highly efficient and stable electrocatalyst for hydrogen evolution in alkaline medium. Electrochim Acta. 2019;299:197–205. doi:10.1016/J.ELECTACTA.2018.12.101.
- Wang H, Wang X, Yang D, et al. Co0.85Se hollow nanospheres anchored on N-doped graphene nanosheets as highly efficient, nonprecious electrocatalyst for hydrogen evolution reaction in both acid and alkaline media. J Power Sources. 2018;400:232–241. doi:10.1016/J.JPOWSOUR.2018.08.027.
- Liu Z, Xu J, Chen D, et al. Flexible electronics based on inorganic nanowires. Chem Soc Rev. 2015;44:161–192. doi:10.1039/C4CS00116H.
- Manthiram A, Fu Y, Chung SH, et al. Rechargeable lithium-sulfur batteries. Chem Rev. 2014;114:11751–11787. doi:10.1021/CR500062V.
- Yang X, Zhang L, Zhang F, et al. Sulfur-infiltrated graphene-based layered porous carbon cathodes for high-performance lithium-sulfur batteries. ACS Nano. 2014;8:5208–5215. doi:10.1021/NN501284Q.
- Qiu Y, Li W, Zhao W, et al. High-rate, ultralong cycle-life lithium/sulfur batteries enabled by nitrogen-doped graphene. Nano Lett. 2014;14:4821–4827. doi:10.1021/NL5020475.
- Digraskar RV, Sapner VS, Mali SM, et al. CZTS decorated on graphene oxide as an efficient electrocatalyst for high-performance hydrogen evolution reaction. ACS Omega. 2019;4:7650–7657. doi:10.1021/ACSOMEGA.8B03587/ASSET/IMAGES/LARGE/AO-2018-03587B_0006.JPEG.
- Shinde P, Rout CS, Late D, et al. Optimized performance of nickel in crystal-layered arrangement of NiFe2O4/rGO hybrid for high-performance oxygen evolution reaction. Int J Hydrogen Energy. 2021;46:2617–2629. doi:10.1016/J.IJHYDENE.2020.10.144.
- Ahmed J, Alam M, Majeed Khan MA, et al. Bifunctional electro-catalytic performances of NiMoO4-NRs@RGO nanocomposites for oxygen evolution and oxygen reduction reactions. J King Saud Univ Sci. 2021;33:101317), doi:10.1016/J.JKSUS.2020.101317.
- Sandhiran N, Ganapathy S, Manoharan Y, et al. Cuo–NiO binary transition metal oxide nanoparticle anchored on rGO nanosheets as high-performance electrocatalyst for the oxygen reduction reaction. Environ Res. 2022;211:112992), doi:10.1016/J.ENVRES.2022.112992.
- Guo Y, Sun X, Liu Y, et al. One pot preparation of reduced graphene oxide (RGO) or Au (Ag) nanoparticle-RGO hybrids using chitosan as a reducing and stabilizing agent and their use in methanol electrooxidation. Carbon N. Y. 2012;50:2513–2523. doi:10.1016/J.CARBON.2012.01.074.
- Govind Rajan A, Martirez JMP, Carter EA. Facet-Independent oxygen evolution activity of pure β-NiOOH: different chemistries leading to similar overpotentials. J Am Chem Soc. 2020;142:3600–3612. doi:10.1021/JACS.9B13708/SUPPL_FILE/JA9B13708_SI_002.ZIP.
- Hsu YK, Yu CH, Chen YC, et al. Synthesis of novel Cu2O micro/nanostructural photocathode for solar water splitting. Electrochim Acta. 2013;105:62–68. doi:10.1016/J.ELECTACTA.2013.05.003.
- Wang Q, Wang H, Qi S, et al. Coral-Like LaNixFe1−xO3 perovskite catalyst for high-performance oxygen evolution reaction. J. Electrochem Soc. 2022;169:026508), doi:10.1149/1945-7111/AC4AB0.
- Rathnayake RMNM, Wijayasinghe HWMAC, Pitawala HMTGA, et al. Synthesis of graphene oxide and reduced graphene oxide by needle platy natural vein graphite. Appl Surf Sci. 2017;393:309–315. doi:10.1016/J.APSUSC.2016.10.008.
- Chen X, Wang X, Zhang X, et al. Facile and scalable synthesis of heterostructural NiSe2/FeSe2 nanoparticles as efficient and stable binder-free electrocatalyst for oxygen evolution reaction. Int J Hydrogen Energy. 2021;46:35198–35208. doi:10.1016/J.IJHYDENE.2021.08.090.
- Ramadoss M, Chen Y, Hu Y, et al. Hierarchically porous nanoarchitecture constructed by ultrathin CoSe2 embedded Fe-CoO nanosheets as robust electrocatalyst for water oxidation. J Mater Sci Technol. 2021;78:229–237. doi:10.1016/J.JMST.2020.10.058.
- Wang X, He J, Yu B, et al. Cose2 nanoparticles embedded MOF-derived Co-N-C nanoflake arrays as efficient and stable electrocatalyst for hydrogen evolution reaction. Appl Catal B Environ. 2019;258:117996. doi:10.1016/J.APCATB.2019.117996.
- Wang X, Zheng B, Yu B, et al. In situ synthesis of hierarchical MoSe2–CoSe2 nanotubes as an efficient electrocatalyst for the hydrogen evolution reaction in both acidic and alkaline media. J Mater Chem A. 2018;6:7842–7850. doi:10.1039/C8TA01552J.
- Wang B, Wang Z, Wang X, et al. Scalable synthesis of porous hollow CoSe2–MoSe2/carbon microspheres for highly efficient hydrogen evolution reaction in acidic and alkaline media. J Mater Chem A. 2018;6:12701–12707. doi:10.1039/C8TA03523G.
- Yu B, Qi F, Zheng B, et al. Self-assembled pearl-bracelet-like CoSe2–SnSe2/CNT hollow architecture as highly efficient electrocatalysts for hydrogen evolution reaction. J Mater Chem A. 2018;6:1655–1662. doi:10.1039/C7TA08955D.
- Zhang Y, Yang J, Dong Q, et al. Highly dispersive MoP nanoparticles anchored on reduced graphene oxide nanosheets for an efficient hydrogen evolution reaction electrocatalyst. ACS Appl Mater Interfaces. 2018;10:26258–26263. doi:10.1021/ACSAMI.8B07133/SUPPL_FILE/AM8B07133_SI_001.PDF.
- Udayabhanu Nagabhushana H, Suresh D, Rajanaika H, Sharma S C, Nagaraju G. Hydrothermal synthesis of TiO2-rGO By green chemical method. Mater Today Proc. 2017;4:11888–11893. doi:10.1016/J.MATPR.2017.09.108.
- Monson PA. Understanding adsorption/desorption hysteresis for fluids in mesoporous materials using simple molecular models and classical density functional theory. Microporous Mesoporous Mater. 2012;160:47–66. doi:10.1016/J.MICROMESO.2012.04.043.
- Wang S, Lu A, Zhong CJ. Hydrogen production from water electrolysis: role of catalysts. Nano Converg. 2021;8:1–23. doi:10.1186/S40580-021-00254-X/FIGURES/16.
- Anantharaj S, Subhashini E, K.S.-A.S., undefined. Respective influence of stoichiometry and NiOOH formation in hydrogen and oxygen evolution reactions of nickel selenides. Elsevier. (n.d.). 2019. [cited 2022 November 9]. https://www.sciencedirect.com/science/article/pii/S0169433219314254?casa_token=b9O2Qzm7nYMAAAAA:DqSic23LfuNnRishePpQq1sdTocW0UqYU0CSM1Glyz2g5FLeUTGIIZ4C-XC-nzvHRvr2Zd4uZ00.
- Mehmood A, Ur-Rehman Z, Altaf M, et al. Niru0.3Se nanoparticles in situ grown on reduced graphene: synthesis and electrocatalytic activity in the oxygen evolution reaction. ChemistrySelect. 2021;6:502–510. doi:10.1002/SLCT.202003711.
- Shinde DV, De Trizio L, Dang Z, et al. Hollow and porous nickel cobalt perselenide nanostructured microparticles for enhanced electrocatalytic oxygen evolution. Chem Mater. 2017;29:7032–7041. doi:10.1021/ACS.CHEMMATER.7B02666.
- Anantharaj S, S.N.-I.J. of H. Nickel selenides as pre-catalysts for electrochemical oxygen evolution reaction: a review. Energy 2020. [cited 2022 November 9] https://www.sciencedirect.com/science/article/pii/S0360319920314294?casa_token=iyUiNXq4XgAAAAAA:DC7KC8S6K85b09nv9oS5uxnITnBkEg389Z6UPodFNQOMcBjTOnjgALJwRBE8wl0Q12l59DYI5xA.
- Zhang Y, Xu J, Lv L, et al. Electronic engineering of CoSe/FeSe2 hollow nanospheres for efficient water oxidation. Nanoscale. 2020;12:10196–10204. doi:10.1039/D0NR01809K.
- Gao R, Zhang H, Energy DY-N., Iron diselenide nanoplatelets: Stable and efficient water-electrolysis catalysts. Elsevier. (n.d.). 2017. [cited 2022 November 9]. https://www.sciencedirect.com/science/article/pii/S2211285516305031?casa_token=HHET7153ICgAAAAA:KS3bjCD4NgV4myLkluwQpEcXv980GhULYRVXH847l4×5YyfpjRpg0l4RqIDUcHjXet4p_rg0B-s
- Liu H, Lu X, Hu Y, et al. Coxfeyn nanoparticles decorated on graphene sheets as high-performance electrocatalysts for the oxygen evolution reaction. J Mater Chem A. 2019;7:12489–12497. doi:10.1039/C9TA01347D.
- Liu Y, Cheng H, Lyu M, et al. Low overpotential in vacancy-rich ultrathin CoSe2 nanosheets for water oxidation. J Am Chem Soc. 2014;136:15670–15675. doi:10.1021/JA5085157.
- Du Y, Cheng G, Luo W. Colloidal synthesis of urchin-like Fe doped NiSe2 for efficient oxygen evolution. Nanoscale. 2017;9:6821–6825. doi:10.1039/C7NR01413A.
- Feng H, Hu H, Dong H, et al. Hierarchical structured carbon derived from bagasse wastes: a simple and efficient synthesis route and its improved electrochemical properties for high-performance supercapacitors. J Power Sources. 2016;302:164–173. doi:10.1016/J.JPOWSOUR.2015.10.063.
- Tang PP, Lin X, Yin H, et al. Hierarchically nanostructured nickel-cobalt alloy supported on nickel foam as a highly efficient electrocatalyst for hydrazine oxidation. ACS Sustain Chem Eng. 2020;8:16583–16590. doi:10.1021/ACSSUSCHEMENG.0C05846/ASSET/IMAGES/LARGE/SC0C05846_0005.JPEG.