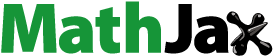
Abstract
Herein, we demonstrate the sample and low coast hydrothermal synthesis of a nanocomposite of Bi2O3/MoSe2 for hybrid photocatalyst. The structure of as-synthesized samples was characterized by using several techniques, including XRD spectroscopy, Raman spectroscopy, XPS, SEM, BET, and UV-visible absorption spectroscopy. The nitrogen adsorption–desorption measurements show that the samples have a mesoporous texture with slits like pores. The Bi2O3/MoSe2 nanocomposite had BET surface areas of 36.439 m2/g, which were more than those of Bi2O3 (18.342 m2/g) and MoSe2 (12.923 m2/g). Under visible illumination, the photocatalytic activities of pure samples ( Bi2O3 and MoSe2) and the hybrid Bi2O3/MoSe2 sample for the disintegration of methylene blue were assessed. The results show that the Bi2O3/MoSe2 sample showed the highest photocatalytic degradation efficiency (i.e. 96.5% after an exposure time of 80 min). The increased photocatalytic activity is due to the mixed sample's high crystallinity and surface area, as well as the small particle size.
1. Introduction
The problem of rising pollution levels in the natural environment is getting worse due to the dynamic expansion of civilization and technological advancement. Also, the increase in these human activities and industrial purposes has led to an increase in pollution in more recent years. These sectors, which include pharmaceuticals, textiles, personal care items, and food processing, have contaminated the water, air, and soil with persistent pollutants. Most of these persistent pollutants are organic and non-biodegradable. Synthetic dyes are dangerous to the environment and contain poisonous refractory compounds that can provide brilliant colour. Most dyes are resistant to aerobic biodegradation [Citation1,Citation2].
The creation of innovative, efficient techniques for removing or eliminating various sorts of pollutants is one of the issues that are currently being taken up in many scientific institutions throughout the world [Citation2–4]. One of the potential waste disposal technologies, particularly for the reduction of organic pollutants like dye contamination, is heterogeneous photocatalytic detoxification of water and wastewater contaminants [Citation3,Citation5–10]. In heterogeneous photocatalysis, it is very important to choose a material that can be used to destroy a certain type of impurity through photocatalysis [Citation11–14].
The fact that this material can only absorb ultraviolet light and exhibits quick recycling of photo-excited electron-hole pairs as well as a huge energy gap and a lower operational quantum yield should be underlined. These characteristics restrict its usefulness in photocatalytic processes [Citation5,Citation6]. Similar to graphene, molybdenum diselenide (MoSe2) is a well-known layered material; a monolayer or few-layered MoSe2 can be produced using a disordered method, and it exhibits highly unique physical and chemical features [Citation15]. Due to its small band gap, MoSe2 is thought to be a possible photocatalyst [Citation7]. In a type-II band alignment, MoSe2's distinct band structure can align with Bi2O3's well-energy band. So, it can help the electrons and holes made by photosynthesis move through the material [Citation8].
Until now, several techniques have been thought of to prepare nanomaterials, including the green calcination method [Citation16], ultrasound-assisted sol–gel method [Citation17], oxidation-condensation reaction technique [Citation18], and mechanical mixing method [Citation19]. The synthesis of nanostructures is based on the preparation of precursor precipitates and the subsequent hydrothermal treatment at a designated temperature. The hydrothermal method is widely used to prepare nanostructural materials because of its simplicity, high efficiency, and low cost [Citation20–22].
From this point of view, this approach, to significantly improve the photocatalytic activity of Bi2O3 when exposed to visible light, we designed and synthesized MoSe2/ Bi2O3 nano composites by using the hydrothermal method. Moreover, the investigated MoSe2, Bi2O3 and MoSe2/Bi2O3 Nano composites were characterized via different physicochemical tools. Furthermore, the photocatalytic activity of the Bi2O3/MoSe2 nano-composites for the elimination of MB when exposed to visible light has been studied and researched.
2. Materials and methods
2.1. Synthesis of Bi2O3
A simple and low-cost hydrothermal method was performed to synthesize Bi2O3. In a typical synthesize, 2 g of Bismuth nitrate and 0.5 g polyvinylpyrrolidone (Sigma-Aldrich) was dissolved in 10 ml of a mixed solution of nitric acid and distilled water (the ratio is 1–9). After that, 70 ml of sodium hydroxide (pellets, 98%, Alfa Aesar) solution was added till the solution achieve the pH = 12 [23]. Then the solution was stirred at room temperature until the colour turned to yellowish white. Then the resulting solution was transferred to a 100 ml stain steel autoclave for 12 h at 200°C. After the hydrothermal process, the autoclave was cooled down at room temperature and the yellow precipitate was gathered utilizing a centrifuge and washed multiple times using distilled water and ethanol. The final resulting product was dried for 8 h at 60°C.
2.2. Synthesis nanocomposite of Bi2O3/MoSe2
The synthesis of Bi2O3/MoSe2 heterostructure was accomplished by using a two-step hydrothermal process. Typically, synthesis involves 0.4 g of selenium powder was added into 10 ml hydrazine hydrate and kept for 24 h. 1 g of ammonium molybdate hexahydrate was added to 50 ml of Di-distilled water. Then, the Se-hydrazine solution was added to the above precursor solution and stirred for one hour. Subsequently, the final solution was removed to a 100 ml stain steel autoclave for 24 h at 200°C. After the hydrothermal process, the autoclave was let to cool naturally. After that, the yellow precipitate of Bi2O3 was mixed with the above solution and stirred for 3 h. Finally, the resulting mixture was filtered by filtered paper and washed several times using Di-distilled water and EtOH to purge existing impurities, and dried for 24 h at 50°C. For comparison, a similar condition was used to obtain the pure form of MoSe2 without adding Bi2O3.
2.3. Assessment of photocatalytic activity
Photocatalytic efficiency of current samples was tested by using 100 mg of the prepared photocatalyst introduced to 200 mL of solutions with 26 mg/L of MB concentration at pH 11.5. A 300 W Xenon lamp with 200 W/m2 intensity was utilized as a visible-light source with a wavelength of 460 nm. The photocatalysis studies were performed in a design reactor at ambient temperature. Before beginning the photocatalytic reaction, the suspension solution was agitated in the dark for 60 min to achieve the adsorption equilibrium. During the irradiation operation, 5 mL solution was withdrawn at a regular interval and separated by centrifugation.
2.4. Instrumental characterization
The identification of the phase structure for the investigated samples were analyzed by X-ray powder diffraction (XRD) using SHIMADZU-MAXima-XRD-7000 diffractometer equipped with Cu–Ka radiation (1.5418 Ǻ). Raman measurement was carried out using a Senterra confocal Raman microscope at a wavelength of 532 nm. X-ray photoelectron microscopy (XPS) was performed using K-ALPHA (Themo Fisher Scientific, USA) with monochromatic X-ray Al K-alpha radiation. Surface morphologies were examined using high-resolution Jeol JSM 7600F field emission scanning electron microscope (FE-SEM). The BET SSA (The Brunauer, Emmett and Teller Specific Surface Area), average pore diameter, and volume were determined by recording nitrogen adsorption–desorption isotherms performed at the boiling point (70 K) of N2 using NOVA 4200e High-Speed, Automated Surface Area and Pore Size Analyzer. UV–vis–NIR spectrophotometer (JASCO, ISN-470) which was operated at λ = 664 nm was utilized to quantify the MB concentration.
3. Results and discussion
3.1. General characterization
The composition and purity of specimens were first characterized by XRD. The XRD pattern of the obtained Bi2O3 is illustrated in Figure (i). According to J.C.P.D.S. file #41-1449, each peak has been given a designation and index. As shown in Figure (ii), all the diffraction peaks can be indexed to the MoSe2 phase (JCPDS Card No. 77-1715), indicating the high purity of the synthesis MoSe2 [Citation23]. The XRD pattern of the freshly prepared sample of the hybrid nanocomposite of Bi2O3/MoSe2 is shown in Figure (iii). The two broad peaks of the MoSe2, which are corresponding to the (100) and (110) planes, were observed, while the peak at 2θ = 13.7°, which corresponds to the (002) plane, is not observed suggesting that the (002) interlayer spacing of the freshly-prepared MoSe2 is dramatically changed in Bi2O3/MoSe2 nanocomposites [Citation24–26].
Figure 1. The XRD patterns of (i) pure Bi2O3, (ii) pure MoSe2, and (iii) hybrid nanocomposite of Bi2O3/MoSe2.

The following equations [Citation27–29] were utilized in the Debye-Scherer analysis in order to estimate the average crystal size (D), the dislocation density (δ), and the microstrain (ε) of the obtained samples:
(1)
(1)
(2)
(2)
(3)
(3)
Table shows how the full width at half maximum, average crystal size, dislocation density, and micro-strain of the samples studied were calculated using the strongest peaks from XRD analysis.
Table 1. The full width at half maximum, average crystal size, dislocation density, and micro-strain of investigated samples calculated from XRD analysis using the most intense peaks.
To further insight into the structure of the Bi2O3/MoSe2, Raman was performed for all samples. Figure (i) shows the Raman spectra of the pure MoSe2. The MoSe2 spectrum exhibits two characteristic peaks located at 235 and at 239 cm−1 which are in agreement with the out-of-plane Aig and in-plane E12g, respectively [Citation30–32]. Figure (ii) represents the Raman spectra of as-synthesized Bi2O3. The ten vibration peaks are observed in Bi2O3 spectra, which are in agreement with the Raman modes in previous reports. The symmetry Ag mode (119 cm−1) is assigned to the Bi atoms’ participation. The two modes Ag (138 cm−1) and Bg (153 cm−1) may result in the displacement of Bismuth and Oxygen atoms in the Bi2O3 lattice. The vibration mode from 183 to 521 cm−1 is attributed to the displacements of the Oxygen atoms in Bi2O3 [Citation33,Citation34]. Figure (iii) shows Raman spectra of the Bi2O3/MoSe2. The hybrid nanocomposite of Bi2O3/MoSe2 clearly shows several peaks that correspond to the vibrational modes of Bi2O3 and MoSe2, implying the existence of both materials in the hydride heterostructure.
The composition and surface electronic state were further investigated using X-ray photoelectron spectroscopy (XPS). Figure (a) exhibits the survey spectra of the hybrid nanocomposite of Bi2O3/MoSe2. As can be seen, the synthesized sample comprises Bi, O, C, Mo, and Se elements. The appearance of a carbon peak could be due to contamination from carbon conductive tape. Figure (b) shows the high-resolution scan of Bi 4f and two typical peaks can be identified at 158.8 and 164 eV assigned to Bi 4f7/2 and Bi 4f5/2, respectively [Citation35]. Figure (c) presents the core level spectrum of O 1S. A single characteristic peak located at the binding energy 531.7 eV was assigned to O 1s due to the bismuth-oxygen binding [Citation35,Citation36]. As observed in Figure (d), the Mo 3d spectrum shows two distinct peaks located at binding energy 228.2 and 231.4 eV corresponding to Mo 3d3/2 and Mo 3d5/2 respectively, consistent with previous reports. In the case of Se 3d, Figure (e) shows the high-resolution Se 3d spectrum [Citation37,Citation38]. The two fitted peaks at 54.4 and 55.3 attribute to the Se 3d5/2 and Se 3d3/2, respectively.
Figure 3. (a) XPS survey spectrum, (b–e) high-resolution spectra of Bi 4f, O 1s, Mo 3d and Se 3d, respectively.

The surface morphologies of powder samples were analyzed using (FE-SEM). The surface morphology was studied at different magnifications to accurately track the effect of composition on microstructure parameters of investigated samples as illustrated in Figure . The FE-SEM analysis illustrates that the Bi2O3 sample (i.e. Figure (a–c)) had nanoparticles with irregular, and aggregated grain with different sizes and random distributions. Furthermore, there is heterogeneity with respect to their distribution and the surface of Bi2O3 particles was relatively smooth and flat. The MoSe2 sample had a spherical like-shape (i.e. Figure (d−f)). The Bi2O3 mixed MoSe2 sample (i.e. Figure (g−i)) had spherical like-shape with fine separation of grain boundaries. This behaviour indicating that the surface structure of the composite is dominated by MoSe2 phase. In addition, the change in particle shape, size and distribution with the combination of Bi2O3/MoSe2 (Figure ), supported the physicochemical interaction between MoSe2 and Bi2O3 nanostructures in the composite. It is clear that the particle size distribution decreases by adding Bi2O3 to MoSe2, as well as the increase in density and the exposed surface area, which is of great importance in the use of these compositions in water purification processes from organic dyes [Citation39–41].
3.2. Nitrogen adsorption–desorption (NA-D) study
The (NA-D) isotherm approach was utilized to investigate the surface areas and pore volume – size of the synthesized samples as illustrated in Figure . The appearance of the H3 hysteresis loop clearly indicates that the samples have a type IV isotherm and the sample has a mesoporous texture with slits like pores. The complete information about BET surface areas and Pore Volume values of synthesized samples are reported in Table . The MoSe2 mixed Bi2O3 composite had BET surface areas of 36.4 m2/g, which were higher than that of Bi2O3 (18.3 m2/g) and MoSe2 (12.9 m2/g). The total pore size of MoSe2 mixed Bi2O3 composite was 1.64 Ǻ, which were lower than that of MoSe2 (2.17 Ǻ) and Bi2O3 (2.26 Ǻ). The large values of BET surface areas and porous structure of MoSe2 mixed Bi2O3 composite will provide more active sites and transport pathways for interacting molecules and products, which have been very useful in photocatalysis [Citation42–44]. The observed and measurable increase in the specific surface areas of the system investigated as a result of mixing Bi2O3 with MoSe2 may be attributed to effective pore narrowing and the formation of new pores as a result of the liberation of volatile constituents caused by the thermal decomposition of the precursors. This may be the case because the thermal decomposition of the precursors causes the precursors to undergo thermal decomposition, which results in the release of volatile constituents. In addition, the observed increase in the SBET of the treated materials might be attributed to the effective, considerable drop in crystallite size. This is because the crystallite size decreased.
3.3. Photocatalytic properties of the prepared catalysts
To study the photocatalytic behaviour of the powder catalysts, photodegradation experiments were done with MB under Visible light irradiation by observing the MB concentration using absorption spectroscopy. For all samples the absorption patterns of MB at λmax = 288 nm and λmax = 665 nm gradually decreased with illumination time without any considerable changes in the absorption lines position. This degradation behaviour indicate that the prepared catalysts appear photocatalytic response in the degradation of dye. The distinctive absorption line of MB at λmax = 665 nm was selected to exemplify the dye degradation rate on continued exposure to Visible light irradiation. The degradation efficiency of contaminated dye was computed with the help of the Beer-Lambert theory, which represents the relationship between the concentration value (Ct) and the absorbance value (At) of dye at time t as [Citation42,Citation45–48]
At initial time t0, C0 and A0 represent the initial values of MB concentration and MB absorbance respectively. Figure (a) illustrates the changing of the MB absorption profile in the presence of prepared catalysts (i.e. Bi2O3, MoSe2, Bi2O3/MoSe2). The results show that the Bi2O3/MoSe2 sample shows the highest MB degradation efficiency (i.e. 96.5% after an exposure time of 80 min). Under the same conditions, Bi2O3 and MoSe2 samples had degradation efficiency of 89.9% and 76.8%, respectively. The photodegradation of MB by prepared catalysts follows pseudo-first-order kinetics after the modified Langmuir Hinshelwood approach [Citation42,Citation45,Citation46,Citation49].
Figure 6. (a) Photocatalytic degradation of MB under visible-light irradiation (b) kinetic measurements for the MB degradation using Bi2O3, MoSe2, and Bi2O3/MoSe2.

By invoking the first-order kinetics principle and drawing ln (Ct/C0) against the time of illumination (t), one can obtain a good linear fit (r2 ∼ (0.97–0.99), Figure (b)). The obtained photodegradation rate constants are 0.0111, 0.0170, and 0.0455 min−1 for Bi2O3, MoSe2, Bi2O3 mixed MoSe2, respectively. Then, the photocatalytic activity improves 4-fold with mixing Bi2O3 with MoSe2. This is a significant improvement in the photocatalytic properties of these compounds. Additionally, to certify dye degradation, a chemical oxygen demand (COD) test was performed. A common technique for assessing the organic content of wastewater is the COD measurement. The experiment enables waste evaluation in terms of the total oxygen required for the oxidation of organic matter to CO2 and water. A considerable drop in COD implies that the sample's carbon content has degraded, which also reveals how much mineralization has occurred. Degradation causes organic carbon to turn into gaseous CO2 [Citation26]. The mineralization operation, that comprised the aromatic rings opening with the temporary development of carboxylic acids, and the generation of CO2 via the “photo-Kolbe” process, drastically reduced the COD (Table ). Based on the drastic drop in COD readings, the dye molecules may have completely mineralized with the usage of a Bi2O3/MoSe2 nanocomposite.
Table 2. Variation of chemical oxygen demand (COD) of MB over the Bi2O3/MoSe2 nanocomposite at various time intervals.
It is interesting to confirm the appropriateness of the designed Bi2O3/MoSe2 catalyst for degrading MB dye, which resembles actual wastewater from textile companies. Table shows that the novel Bi2O3/MoSe2 catalyst developed in this work is superior to other known catalysts when used in photodegradation reactions of MB.
Table 3. Comparison of photodegradation rate constant and degradation efficiency of MB using different photocatalysts with the developed Bi2O3/MoSe2 nanocomposition.
3.4. Photocatalyst stability studies
In the field of water purification, determining the economic and operational efficiency of a photocatalyst depends on many factors, the most important of which is the recycling stability test of the photocatalyst in the purification of pigments from organic pollutants, which determines its importance at the industrial and economic levels. The photolysis stability process was carried out in the following steps: first, adding 50 mL of MB (25.6 mg/L) at pH 11.5–25 mg of the catalyst. Second, the mixture was exposed to sunlight for specific periods of time, and the absorbance was measured. This process was repeated six times. In the standard recycling process, the same sample is taken into account as a typical catalyst model, and all measurements are made on it after it has been separated by a centrifugal device each time. The purity of the sample was also checked after separation by washing it more than three times in succession with distilled water and acetone. The obtained powder was dried at 80°C for 2 h to be the photocatalyst source in the next photodegradation run (Figure ). No considerable retardation in the photodegradation efficiency is evident until the sixth cycle (Figure ), reflecting the high and steady photocatalytic performance of the catalysts produced.
3.5. Photocatalytic degradation mechanism
Scavenger studies were performed with a variety of scavengers, including using t-BuOH and KI as scavengers in the presence of Bi2O3/MoSe2 nanoparticles for the purpose of trapping holes (h+), electrons (e−), and superoxide radicals (O2−), respectively (Figure ). This helped researchers determine the primary species that govern photocatalytic degradation and the mechanism of photocatalysis. The effectiveness of the degradation was determined by contrasting the level of activity depicted in Figure . The findings indicate that the catalytic efficiency was most significantly impacted when KI was present. This is because the efficiency of degradation decreased by 38% when KI was present, which indicates that holes played the primary role in the degradation process. However, the efficacy of degradation was significantly decreased in the case of t-BuOH (59%), which confirms that superoxide radicals play a role in the degradation process. This demonstrates that holes and superoxide radicals were the primary factors that contributed to the degradation of the MB dye. Therefore, based on trapping experiments the plausible mechanism of photocatalysis is shown in Figure .
Figure 8. Trapping test for MB degradation using t-BuOH and KI as scavengers in the presence Bi2O3–MoSe2.

Since MoS2's bandgap energy is tunable and active in visible light and Bi2O3's is active in the UV region, the valence electrons in MoS2 are stimulated to the conduction band level as visible light is absorbed, leaving the holes behind. Nanoparticle Bi2O3 may also absorb UV light. Nanoparticles of Bi2O3 and MoS2 can interact and transfer energy to one another, which may improve the photocatalytic reactions of these hybrid nanocomposite materials (Figure ). Typically, electrons from the conduction band of Bi2O3 will move to the valence band of MoS2, where they will combine with holes. In the absence of recombination, charge separation takes place between the electron holes. This results in a higher density of electrons in the MoS2 conduction band. In addition, visible light has the potential to directly activate MoS2. When two carriers are excited simultaneously, recombination of photogenerated electron-hole pairs is considerably inhibited, leading to a higher concentration of active species capable of degrading the dye. The CB's free electrons then combine with molecular oxygen to produce superoxide radicals, which can either destroy contaminants directly or react with water to produce hydroxyl radicals. Conversely, the VB holes can react with water to produce hydroxyl radicals, which in turn degrade contaminants to carbon dioxide (CO2), water, or other, less complex compounds.
4. Conclusion
We have demonstrated a sample method to synthesize Nano-composites of Bi2O3/MoSe2. The structure of the investigated Nano-composites was characterized via different physiochemical and analytical tools in order to comprehend more about their morphology, phase, size, and content. The XRD study of the nanocomposites revealed diffraction lines connected to Bi2O3 and MoSe2 NPs. The produced samples displayed good purity, stoichiometric constitution, and spherical form morphology, according to the Raman, XPS, and SEM examinations. Moreover, the hybrid composite showed enhanced photocatalytic efficiency toward the degradation of MB under visible irradiation. Our results show that the mixed sample showed the highest photocatalytic activity under visible radiation and achieved the maximum degradation efficiency of 96.5% after irradiation for 80 min (photo degradation rate constant of 0.0455 min−1).
Disclosure statement
No potential conflict of interest was reported by the author(s).
Data availability statement
The data that support the findings of this study are available from the corresponding author upon reasonable request.
References
- Muruganandham M, Swaminathan M. Solar photocatalytic degradation of a reactive azo dye in TiO2-suspension. Sol Energy Mater Sol Cells. 2004;81:439–457.
- Abu-Dief AM, Abdel-Rahman LH, Abd-ElSayed MA, et al. Green synthesis of silver nanoparticles using Delonix regia extract, characterization and its application as adsorbent for removal of Cu (II) ions from aqueous solution. Asian J Appl Chem Res. 2021;9(1):1–15.
- Abu-Dief AM, Mohamed WS. Development of nanomaterials as photo catalysts for environmental applications. Curr Catal. 2020;9:128–137.
- Abu-Dief AM, Abdel-Rahman LH, Sayed MA, et al. Optimization strategy for green synthesis of silver nanoparticles (AgNPs) as catalyst for the reduction of 2, 4-dinitrophenol via supported mechanism. Appl Phys A. 2022;128:1–13.
- Hashimoto K, Irie H, Fujishima A. Tio2 photocatalysis: a historical overview and future prospects. Jpn J Appl Phys. 2005;44:8269.
- Mahlambi MM, Ngila CJ, Mamba BB. Recent developments in environmental photocatalytic degradation of organic pollutants: the case of titanium dioxide nanoparticles—a review. J Nanomater. 2015;2015, article id 790173, 29p.
- Chen L, Wang J, Li X, et al. Facile preparation of Ag2S/KTa0. 5Nb0.5O3 heterojunction for enhanced performance in catalytic nitrogen fixation via photocatalysis and piezo-photocatalysis. Green Energy Environ. 2022. doi:10.1016/j.gee.2022.03.007.
- Chen L, Wang J, Li X, et al. A novel Z-scheme Bi-Bi2O3/KTa0.5Nb0.5O3 heterojunction for efficient photocatalytic conversion of N2 to NH3. Inorg Chem Front. 2022;9:2714–2724.
- Li X, Chen L, Wang J, et al. Novel platinum-bismuth alloy loaded KTa0.5Nb0.5O3 composite photocatalyst for effective nitrogen-to-ammonium conversion. J Colloid Interface Sci. 2022;618:362–374.
- Feng Z, Zeng L, Zhang Q, et al. In situ preparation of g-C3N4/Bi4O5I2 complex and its elevated photoactivity in methyl orange degradation under visible light. J Environ Sci. 2020;87:149–162.
- Akhundi A, Habibi-Yangjeh A, Abitorabi M, et al. Review on photocatalytic conversion of carbon dioxide to value-added compounds and renewable fuels by graphitic carbon nitride-based photocatalysts. Catal Rev. 2019;61:595–628.
- Akhundi A, Badiei A, Ziarani GM, et al. Graphitic carbon nitride-based photocatalysts: toward efficient organic transformation for value-added chemicals production. Mol Catal. 2020;488:110902.
- Habibi-Yangjeh A, Asadzadeh-Khaneghah S, Feizpoor S, et al. Review on heterogeneous photocatalytic disinfection of waterborne, airborne, and foodborne viruses: can we win against pathogenic viruses? J Colloid Interface Sci. 2020;580:503–514.
- Asadzadeh-Khaneghah S, Habibi-Yangjeh A. g-C3N4/carbon dot-based nanocomposites serve as efficacious photocatalysts for environmental purification and energy generation: a review. J Clean Prod. 2020;276:124319.
- Alahmadi M, Mahvash F, Szkopek T, et al. A two-step chemical vapor deposition process for the growth of continuous vertical heterostructure WSe 2/h-BN and its optical properties. RSC Adv. 2021;11:16962–16969.
- Jiang X, Tan H, Shi X, et al. Preparation of Bi2O3/BiOI step-scheme heterojunction photocatalysts and their degradation mechanism of methylene blue. J Wuhan Univ Technol Sci Ed. 2022;37:801–806.
- Asadzadeh Patehkhor H, Fattahi M, Khosravi-Nikou M. Synthesis and characterization of ternary chitosan–TiO2–ZnO over graphene for photocatalytic degradation of tetracycline from pharmaceutical wastewater. Sci Rep. 2021;11:1–17.
- Bavykina A, Kolobov N, Khan IS, et al. Metal–organic frameworks in heterogeneous catalysis: recent progress, new trends, and future perspectives. Chem Rev. 2020;120:8468–8535.
- Mahmoodi H, Fattahi M, Motevassel M. Graphene oxide–chitosan hydrogel for adsorptive removal of diclofenac from aqueous solution: preparation, characterization, kinetic and thermodynamic modelling. RSC Adv. 2021;11:36289–36304.
- Marzouk AA, Abu-Dief AM, Abdelhamid AA. Hydrothermal preparation and characterization of ZnFe2O4 magnetic nanoparticles as an efficient heterogeneous catalyst for the synthesis of multi-substituted imidazoles and study of their anti-inflammatory activity. Appl Organomet Chem. 2018;32:e3794.
- Abu-Dief AM, Nassar IF, Elsayed WH. Magnetic NiFe2O4 nanoparticles: efficient, heterogeneous and reusable catalyst for synthesis of acetylferrocene chalcones and their anti-tumour activity. Appl Organomet Chem. 2016;30:917–923.
- Abu-Dief AM, Abdelbaky MSM, Martínez-Blanco D, et al. Effect of chromium substitution on the structural and magnetic properties of nanocrystalline zinc ferrite. Mater Chem Phys. 2016;174:164–171.
- Zhang J, Kang W, Jiang M, et al. Conversion of 1T-MoSe2 to 2H-MoS2xSe2−2x mesoporous nanospheres for superior sodium storage performance. Nanoscale. 2017;9:1484–1490.
- Xie J, Zhang J, Li S, et al. Controllable disorder engineering in oxygen-incorporated MoS2 ultrathin nanosheets for efficient hydrogen evolution. J Am Chem Soc. 2013;135:17881–17888.
- Gao M-R, Chan MKY, Sun Y. Edge-terminated molybdenum disulfide with a 9.4-Å interlayer spacing for electrochemical hydrogen production. Nat Commun. 2015;6:1–8.
- Jiang M, Zhang J, Wu M, et al. Synthesis of 1T-MoSe2 ultrathin nanosheets with an expanded interlayer spacing of 1.17 nm for efficient hydrogen evolution reaction. J Mater Chem A. 2016;4:14949–14953.
- Awad MA, Abu-Dief AM. Tuning the luminescence performance of CdO nanoparticles via Tb2O3 inclusion. Phys Scr. 2022;97:85811.
- Mohamed WS, Alzaid M, Abdelbaky MSM, et al. Impact of Co2+ substitution on microstructure and magnetic properties of CoxZn1-xFe2O4 nanoparticles. Nanomaterials. 2019;9:1602.
- Mohamed WS, Abu-Dief AM. Impact of rare earth europium (RE-Eu3+) ions substitution on microstructural, optical and magnetic properties of CoFe2− xEuxO4 nanosystems. Ceram Int. 2020;46:16196–16209.
- Yang M, Cheng X, Li Y, et al. Anharmonicity of monolayer MoS2, MoSe2, and WSe2: A Raman study under high pressure and elevated temperature. Appl Phys Lett 2017;110:93108.
- Huang C, Wu S, Sanchez AM, et al. Lateral heterojunctions within monolayer MoSe2–WSe2 semiconductors. Nat Mater. 2014;13:1–6. doi:10.1038/nmat4064.
- Gupta U, Naidu BS, Maitra U, et al. Characterization of few-layer 1T-MoSe2 and its superior performance in the visible-light induced hydrogen evolution reaction. APL Mater. 2014;2:92802.
- Ho C-H, Chan C-H, Huang Y-S, et al. The study of optical band edge property of bismuth oxide nanowires α-Bi2O3. Opt Express. 2013;21:11965–11972.
- Fujita I, Edalati P, Wang Q, et al. Novel black bismuth oxide (Bi2O3) with enhanced photocurrent generation, produced by high-pressure torsion straining. Scr Mater. 2020;187:366–370.
- Han X, Li Y, Wang H, et al. Controlled preparation of β-Bi2O3/Mg–Al mixed metal oxides composites with enhanced visible light photocatalytic performance. Res Chem Intermed. 2020;46:5009–5021.
- Singh S, Sahoo RK, Shinde NM, et al. Asymmetric faradaic assembly of Bi 2 O 3 and MnO2 for a high-performance hybrid electrochemical energy storage device. RSC Adv. 2019;9:32154–32164.
- Zhao Y, Lee H, Choi W, et al. Large-area synthesis of monolayer MoSe 2 films on SiO2/Si substrates by atmospheric pressure chemical vapor deposition. RSC Adv. 2017;7:27969–27973.
- Huang Y, Miao Y-E, Fu J, et al. Perpendicularly oriented few-layer MoSe 2 on SnO2 nanotubes for efficient hydrogen evolution reaction. J Mater Chem A. 2015;3:16263–16271.
- Mohamed WS, Nucara A, Calestani G, et al. Optical study of the vibrational and dielectric properties of BiMnO 3. Phys Rev B. 2015;92:54306.
- Alzaid M, Mohamed WS, El-Hagary M, et al. Optical properties upon ZnS film thickness in ZnS/ITO/glass multilayer films by ellipsometric and spectrophotometric investigations for solar cell and optoelectronic applications. Opt Mater (Amst). 2021;118:111228.
- Hadia NMA, Mohamed WS, Abd El-sadek MS. Simultaneous synthesis of various Sb2S3 nanostructures by vapor transport technique. Mater Chem Phys. 2019;235:121750.
- Abu-Dief AM, Essawy AA, Diab AK, et al. Facile synthesis and characterization of novel Gd2O3–CdO binary mixed oxide nanocomposites of highly photocatalytic activity for wastewater remediation under solar illumination. J Phys Chem Solids. 2021;148:109666.
- Hadia NMA, Aljudai M, Alzaid M, et al. Synthesis and characterization of undoped and copper-doped zinc oxide nanowires for optoelectronic and solar cells applications. Appl Phys A. 2022;128:1–11.
- Mohamed WS, Hadia NMA, Alzaid M, et al. Impact of Cu2+ cations substitution on structural, morphological, optical and magnetic properties of Co1-xCuxFe2O4 nanoparticles synthesized by a facile hydrothermal approach. Solid State Sci. 2022;125:106841.
- Mohamed WS, Abu-Dief AM. Synthesis, characterization and photocatalysis enhancement of Eu2O3-ZnO mixed oxide nanoparticles. J Phys Chem Solids. 2018;116:375–385.
- Abu-Dief AM, Mohamed WS. α-Bi2O3 nanorods: synthesis, characterization and UV-photocatalytic activity. Mater Res Express. 2017;4:35039.
- Sun Y, Xiong T, Ni Z, et al. Improving g-C3N4 photocatalysis for NOx removal by Ag nanoparticles decoration. Appl Surf Sci. 2015;358:356–362.
- Dong F, Xiong T, Sun Y, et al. Controlling interfacial contact and exposed facets for enhancing photocatalysis via 2D–2D heterostructures. Chem Commun. 2015;51:8249–8252.
- Hashemi E, Poursalehi R, Delavari H. Structural, optical and photocatalytic activity of multi-heterojunction Bi2O3/Bi2O2CO3/(BiO)4CO3 (OH)2 nanoflakes synthesized via submerged DC electrical discharge in urea solution. Nanoscale Res Lett. 2022;17:1–16.
- Monga D, Basu S. Tuning the photocatalytic/electrocatalytic properties of MoS2/MoSe2 heterostructures by varying the weight ratios for enhanced wastewater treatment and hydrogen production. RSC Adv. 2021;11:22585–22597.
- Khabiri G, Aboraia AM, Soliman M, et al. V A novel α-Fe2O3@ MoS2QDs heterostructure for enhanced visible-light photocatalytic performance using ultrasonication approach. Ceram Int. 2020;46:19600–19608.
- Mittal H, Khanuja M. Nanosheets-and nanourchins-like nanostructures of MoSe2 for photocatalytic water purification: kinetics and reusability study. Environ Sci Pollut Res. 2020;27:23477–23489.
- Qin H, Guo R-T, Liu X-Y, et al. Z-Scheme MoS 2/gC 3 N 4 heterojunction for efficient visible light photocatalytic CO2 reduction. Dalt Trans. 2018;47:15155–15163.
- Faisal M, Ibrahim AA, Bouzid H, et al. Hydrothermal synthesis of Sr-doped α-Bi2O3 nanosheets as highly efficient photocatalysts under visible light. J Mol Catal A Chem. 2014;387:69–75.
- Shahzad K, Fernandez-Garcia J, Khan MI, et al. Formulation of bismuth (Bi2O3) and cerium oxides (CeO2) nanosheets for boosted visible light degradation of methyl orange and methylene blue dyes in water. Catalysts. 2022;12:1197.