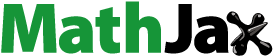
ABSTRACT
Metal pollution today has reached the level of a great threat to humans and their environment. A novel green solution was presented for metal pollution in aquatic environment using Pyracantha coccinea M. J. Roemer in the current study. Due to its widespread industrial use, manganese pollution in water environment has become a serious issue in recent years, and for this reason, it was used as a model metal to determine the treatment potential of P. coccinea M. J. Roemer. The results showed that the pseudo-second-order kinetic model and Freundlich isotherm model agreed well with the experimental data, and the biosorption process had a physical, spontaneous and favourable nature. The maximum metal biosorption capacity was determined to be 59.867 mg g−1. This study showed that P. coccinea M. J. Roemer could present an environmentally friendly, cost-effective, effectual solution for the metal issue in aquatic environment.
1. Introduction
Metals are inorganic substances that we use widely in almost every aspect of our lives. In addition, some metals such as copper, zinc and manganese are needed in low amounts for vital functions in us and other living things. However, some metals such as mercury, cadmium and lead cause vital problems even in very low amounts [Citation1,Citation2]. In recent years, especially as a result of industrial, agricultural, mining and domestic intense anthropogenic activities, a large amount of metal has been released into aquatic environment [Citation3,Citation4]. This situation causes even more pressure on the limited amount of usable water in the world for vital activities. Metals are non-biodegradable, persistent micropollutants and have bioaccumulation and biomagnification properties. They cause numerous serious vital troubles, including toxicity and carcinogenicity, on humans and other living things [Citation2,Citation5–7]. For this reason, it is vital to reduce the amount of metal in aquatic environment to a level that will not harm living life for the continuity of biological activity in the world ecosystem in an orderly manner.
A variety of techniques such as extraction, accumulation, oxidation, coagulation, membrane separation, reduction, ion exchange and chemical precipitation are usually used to remove metals from aqueous medium [Citation4,Citation8,Citation9]. But most of these methods have troubles such as high energy consumption, secondary pollution formation, usage of noxious chemicals, low efficiency, high cost, generation of harmful by-products and inefficiency at low metal concentration levels [Citation4,Citation5,Citation10]. Biosorption, in which various non-living biomass is used as biosorbent, is currently considered the most suitable alternative metal treatment option. It is a flexible, low-cost, efficient, simple, environmentally friendly and promising sustainable removal technology [Citation3,Citation11–13].
Selection of biosorbent material is key to an efficient biosorption operation [Citation10,Citation14]. Therefore, in recent years, numerous studies have focused on the search for efficient biosorbent for metal removal [Citation10,Citation15,Citation16]. Within this perspective, the current study aimed to present a novel green, efficient, cost-effective and sustainable solution for metal pollution in aquatic environment with Pyracantha coccinea M. J. Roemer. P. coccinea M. J. Roemer is an easy-care, robust shrub-shaped plant species that is widely available in many countries around the world. The plant has widespread use in many fields such as biomonitoring, medicine, food, decoration, erosion prevention and biocontrol [Citation17–19]. In addition to these fields, the use of the plant material in the biosorption process, which is an environmentally friendly cleanup technology, and opportunities such as reuse of the final material in the treatment process after desorption operation or as fertilizer provide an important sustainable contribution to the circular bioeconomy [Citation20–22]. On the other hand, manganese pollution in water environment has become a serious issue in recent years due to its widespread industrial use. Manganese is an essential trace element required for biological activities in human beings and other living things. When this element is found in high amounts in water medium, it causes crucial troubles on living health, as well as many problems on water quality for domestic and industrial applications [Citation23–26]. For this reason, manganese was used as a model metal in the present study to determine the biosorption potential of P. coccinea M. J. Roemer. The micro-morphological structure and active site composition of the green biosorbent were explored by SEM and FTIR studies to characterize the possible interaction of the biosorbent material with the metal ions. The biosorption efficiency of the biosorbent for the removal of the metal from aqueous medium was investigated under different operating conditions. Various biosorption models were applied to the experimental data to understand the nature of treatment process. These studies were of great importance for the practical usage of the biosorbent material for the metal detoxification.
2. Materials and methods
2.1. Preparation of green biosorbent
P. coccinea M. J. Roemer fruit biomass was collected from a local park in Sinop, Turkey. The plant biomass was thoroughly cleaned from dust and dirt by washing with tap water and distilled water. The material was oven-dried at a temperature of 80°C for about 24 h. It was crushed into powder with the help of a grinder and sieved with a 0.5 mm mesh sieve. The powdered plant material was then mixed with dilute sodium hydroxide solution (0.3 mol L−1) using a magnetic shaker at room temperature for about 24 h. After the pretreatment, the biomass was thoroughly washed with distilled water to remove the remaining chemical and achieve neutral pH. Finally, the treated biomass was dried again and stored in an airtight bottle for the metal biosorption experiment.
2.2. Preparation of metal solution
Manganese (Manganese (II) chloride dihydrate, purity: ≥99.0%, CAS No: 20603-88-7, Merck) used as model metal in this study was provided by a local chemical supplier in Samsun, Turkey. A stock solution of manganese (1000 mg L−1) was made by dissolving the required amount of the metal in distilled water. The stock solution was then diluted to the required metal concentrations (10–30 mg L−1) using distilled water to get the desired metal-laden solution grades. Dilute hydrochloric acid (0.1 mol L−1) and sodium hydroxide (0.1 mol L−1) solutions were used to adjust the pH levels of the prepared metal-loaded solution samples. The chemicals of analytical purity were used in the metal biosorption study.
2.3. Biosorption operation
The metal biosorption study was performed using batch experiment technique at room temperature on an orbital shaker. A certain amount of the biosorbent material and a certain concentration of the metal solution were added to a conical flask with a working volume of 100 mL and mixed at 150 rpm for a predetermined time. After the experiment period was completed, approximately 1.5 mL of solution sample was taken from the mixture and then the biosorbent material was separated from the solution phase. The metal concentration in the sample was quantified using a manganese test set (Merck Spectroquant 114770) by measuring the absorbance at 445 nm with the aid of a UV-Visible spectrophotometer device (Thermo Genesys 10 S). The following equations were used to determine the efficiency of the biosorbent material to remove the metal from the solution phase at a specified time (t) and equilibrium (e), respectively.
(1)
(1)
(2)
(2)
where qt (mg g−1), qe (mg g−1), C0 (mg L−1), Ct (mg L−1), Ce (mg L−1), V (L) and m (g) are the efficiency of the biosorbent at a specified time, the efficiency of the biosorbent at equilibrium, the metal concentration at start time, the metal concentration at a specified time, the metal concentration at equilibrium, the solution volume and the biosorbent mass, respectively.
2.4. Characterization
The micro-morphological structure and active site composition of the biosorbent material were investigated by SEM and FTIR studies to characterize the possible interaction of the biosorbent material with the metal ions using SEM (Zeiss Evo Ls 10) and FTIR (PerkinElmer Spectrum 400) instruments.
2.5. Study of operating conditions
The biosorption efficiency of the biosorbent material for the removal of the metal from aqueous medium was investigated using the single-factor experiment approach under different operating conditions, including the metal concentration (C0: 10–30 mg L−1, t: 120 mins, pH: 6, m: 10 mg), pH (pH: 2–6, t: 120 mins, C0: 15 mg L−1, m: 10 mg), amount of biosorbent (m: 10–30 mg, t: 120 mins, C0: 25 mg L−1, pH: 6) and operation time (t: 0–120 mins, C0: 30 mg L−1, pH: 6, m: 10 mg).
2.6. Modelling of metal biosorption process
Various biosorption kinetics, thermodynamics and isotherm models were applied to the experimental data to understand the nature of the metal treatment process. The kinetics modelling study was done using the pseudo-first-order, pseudo-second-order and intra-particle diffusion models [Citation9,Citation27–29]. The standard Gibbs free energy change thermodynamics modelling parameter was used to determine the feasibility and spontaneity of the metal biosorption operation [Citation8]. The isotherm modelling study was made using Freundlich, Langmuir and Dubinin-Radushkevich models [Citation30–33].
2.7. Data evaluation procedure
The mathematical evaluation of the metal biosorption operation was carried out using the experimental data averages with the help of SigmaPlot 12.0 software. The compatibility of the experimental data with the mathematical equations was determined by the nonlinear regression approach. R2, adjusted R2 (adjR2) and RMSE parameters were used to evaluate the fit quality of the mathematical equations.
3. Results and discussion
3.1. Characterization study
The micro-morphological structure and active site composition of the biosorbent were studied by SEM and FTIR instruments to characterize the possible interaction of the biosorbent with the metal ions. exhibits the micrograph of SEM for the biosorbent (a) and the metal-loaded biosorbent (b). SEM surface investigation indicated that the biosorbent had an irregular and rough surface composition. These surface properties are very valuable for the biosorption of the metal ions to the biosorbent surface [Citation3,Citation4,Citation34]. The change in the surface morphology of the biosorbent after the metal biosorption resulted from the binding of the metal ions to the biosorbent surface. On the other hand, shows the spectrum of FTIR for the biosorbent (a) and the metal-loaded biosorbent (b). The main FTIR spectrum bands of the biosorbent material were at the positions of 3331.88, 2921.24, 2851.21, 2380.09, 2348.25, 2313.24, 1727.52, 1600.19, 1415.56, 1320.06, 1250.03, 1024.02, 890.32, 645.21 and 594.28 cm−1. These FTIR spectral bands indicated the presence of functional groups such as O-H, C–C, N-H, C–H, C = O, C–N, C = C, C–O and C–O–C, which were in the structure of bio-macromolecules in the biosorbent [Citation3,Citation6,Citation11,Citation35]. FTIR analysis showed that the biosorbent material had a wide variety of functional groups. Various changes such as the shape differences, position shifts, intensity differences, appearance and disappearance of FTIR spectral bands of the biosorbent after the biosorption of the metal ions showed that this plentiful functional group framework played a major role in the metal biosorption process.
3.2. Operating conditions analysis
The biosorption efficiency of the biosorbent for the metal removal from aqueous medium was investigated under main operating conditions, including the concentration of the metal, pH, the biosorbent amount and operation time.
The effect of the metal concentration on the biosorption operation is indicated in (a). The biosorption capacity of the biosorbent increased from 37.864 mg g−1 to 52.427 mg g−1 as the concentration of the metal increased from 10 mg L−1 to 30 mg L−1. The high concentration of the metal in the solution reduced the mass transfer resistance and increased the chance of effective collision between the biosorbent particles and the metal ions, resulting in increased biosorption performance of the biosorbent. However, when the biosorption capacity of the biosorbent was calculated as a percentage, it was noticed that its biosorption yield decreased with the increase of the metal concentration (data not presented). At the low metal concentration, the ratio of the active sites on the biosorbent material to the total metal ions was high, which increased the biosorption efficiency by increasing the probability of interaction between the biosorbent particles and the metal ions [Citation13,Citation23].
The effect of pH on metal biosorption process is shown in (b). The biosorption capacity of the biosorbent increased from 10.680 mg g−1 to 38.835 mg g−1 with the increase of pH level from 2 to 6. At the low pH level, the surface of the biosorbent became positively charged by the protonation of the active sites on the biosorbent, which reduced the biosorption efficiency of the biosorbent as it created an electrostatic repulsion effect against the positively charged metal ions. At the high pH level, the positively charged active sites on the biosorbent were deprotonated and became negatively charged. These negatively charged active sites enhanced the biosorption capacity of the biosorbent by retaining the positively charged metal ions well due to the force of electrostatic attraction [Citation3,Citation5,Citation33].
The effect of the biosorbent amount on the metal biosorption capacity of the biosorbent material is indicated in (c). The biosorption performance of the biosorbent decreased from 55.340 mg g−1 to 41.748 mg g−1 as the amount of the biosorbent increased from 10 mg to 30 mg. At the high biosorbent amount, the overlapping of the biosorbent particles and the fact that a large number of the active binding sites remained empty caused a decrease in the metal biosorption capacity of the biosorbent. However, when the metal biosorption performance of the biosorbent was examined as a percentage, it was observed that the biosorption capacity of the biosorbent increased as the biosorbent amount increased (data not shown). This increase in the biosorption efficiency of the biosorbent was due to the availability of larger surface area and higher numbers of the active binding sites with rising the biosorbent amount [Citation6,Citation36].
The effect of operation time on the metal biosorption process is shown in (d). At first, the biosorption process was rapid and most of the metal biosorption occurred in this stage. The biosorbent material had a large number of available active binding sites and an empty surface at this stage, resulting in rapid and intense metal biosorption. Later on, the biosorption performance of the biosorbent increased to a certain extent, but its biosorption rate gradually decreased. At this stage, most of the binding sites of the biosorbent were occupied by the metal ions as the biosorption process progressed, resulting in a reduction in the speed of the biosorption. Eventually, the biosorption activity hardly increased and the biosorption process reached the equilibrium state as the available binding sites of the biosorbent were almost completely filled by the metal ions [Citation3,Citation33]. Hereby, the biosorbent material achieved the maximum biosorption performance at a metal concentration of 30 mg L−1, a pH of 6 and a biosorbent amount of 10 mg in a 120-minute operation period.
3.3. Metal biosorption modelling study
Various biosorption kinetics, thermodynamics and isotherm models were applied to the experimental data to understand the nature of the metal biosorption process. The kinetics modelling study was performed using the following models.
(3)
(3)
(4)
(4)
(5)
(5)
where k1 (min−1), k2 (g mg−1 min−1), C (mg g−1) and kp (mg g−1 min−1/2) are the pseudo-first-order rate constant, the pseudo-second-order rate constant, a parameter about the boundary layer thickness and the intra-particle diffusion rate constant, respectively. The results of kinetics modelling study for the metal biosorption are listed in . The biosorption kinetics for the metal removal from aqueous medium followed the pseudo-second-order model. This showed that the electrostatic mechanism, which included the electron exchanging or sharing between the active sites on the biosorbent and the metal ions, dominated the metal biosorption process [Citation6,Citation37–39]. On the other hand, the effect of the intra-particle diffusion on the metal biosorption is shown in . The mass transfer (movement) of the metal ions from aquatic environment onto the biosorbent consisted of multiple steps. The first stage was the external (film) diffusion, in which the metal ions were transported from the solution bulk phase to the outer surface of the biosorbent. The next stage was intra-particle diffusion, in which the metal ions were transported from the external surface of the biosorbent into its pores. The final stage referred to the biosorption (surface reaction), in which the metal ions were retained by the biosorbent [Citation13,Citation40]. Furthermore, the curve of the metal biosorption capacity (qt) against √t was non-linear and did not pass through the point of origin [Citation16]. These results revealed that there were multiple stages (mechanisms) controlling the metal ions biosorption operation.
Table 1. Biosorption kinetics modelling results.
The standard Gibbs free energy change thermodynamics modelling parameter was used to determine the feasibility and spontaneity of the metal biosorption process. The following equations were used to calculate this thermodynamics parameter.
(6)
(6)
(7)
(7)
where ΔG° (kJ mol−1), R (J mol−1 K−1), T (K), KD and Ceb (mg L−1) are the standard Gibbs free energy change parameter, the gas constant, the temperature, the distribution coefficient and the metal concentration on the biosorbent at equilibrium, respectively. The standard Gibbs free energy change parameter for the metal biosorption operation was obtained in the range of −4.375 and −1.840 kJ mol−1. The negative standard Gibbs free energy change shows that the biosorption process is of a feasible and spontaneous nature. Again, the negative standard Gibbs free energy change parameter between −400 and −80 kJ mol−1 refers a chemisorption process, and between −20 and 0 kJ mol−1 refers a physisorption process [Citation4,Citation33]. Thus, the standard Gibbs free energy change values obtained for the current study indicated that the biosorption process had a physical, spontaneous and favourable nature.
The isotherm modelling study was carried out using the following equations.
(8)
(8)
(9)
(9)
(10)
(10)
(11)
(11)
(12)
(12)
(13)
(13)
where KF (mg g−1 (L mg−1)1/nF), nF, qm (mg g−1), KL (L mg−1), RL, B (mol2 kJ−2), ε and E (kJ mol−1) are a parameter about the metal biosorption capacity of the biosorbent material, a parameter about the biosorption intensity, the maximum metal biosorption efficiency, a parameter about the biosorption energy, the equilibrium (separation) factor, a parameter about the energy of biosorption, the Polanyi potential and the mean free energy, respectively. The results of isotherm modelling study for the metal biosorption operation are shown in . The experimental metal biosorption data agreed well with Freundlich isotherm model. This showed that a multi-layer biosorption mechanism operated between the metal ions and the active binding sites on the heterogeneous surface of the biosorbent [Citation1,Citation41]. On the other hand, nF, RL and E parameters were estimated as 4.163, 0.113–0.277 and 0.440 kJ mol−1, respectively. nF parameter between 0 and 10 refers a favourable biosorption process. Again, RL parameter between 0 and 1 also shows a favourable biosorption operation. E parameter between 0 and 8 kJ mol−1 indicates a physisorption process, and between 8 and 16 kJ mol−1 indicates a chemisorption process [Citation10,Citation13,Citation16,Citation35,Citation40]. Thence, nF, RL and E parameters values obtained for the present study showed that the metal biosorption operation had a favourable and physical nature.
Table 2. Biosorption isotherm modelling results.
3.4. Comparison study for metal biosorption capacity
The maximum metal biosorption capacity of P. coccinea M. J. Roemer was determined to be 59.867 mg g−1. A literature review comparing the capacity of various biosorbent materials for the metal biosorption from aqueous environment is shown in . As could be seen from the table, the biosorbent was quite successful in removing the metal from aqueous medium. This indicated that P. coccinea M. J. Roemer could be a cost-effective and effective material for an environmentally friendly solution to the metal issue in aquatic environment.
Table 3. Biosorption capacity comparison table.
4. Conclusions
A novel green solution for metal pollution in aquatic environment was presented using P. coccinea M. J. Roemer in this study. Manganese was used as a model metal to determine its biosorption potential. Characterization study showed that the material had an irregular and rough surface and a wide variety of functional groups, which played an important role in metal biosorption. The biosorbent achieved the maximum metal treatment capacity at a metal concentration of 30 mg L−1, a pH of 6 and a biosorbent amount of 10 mg in a 120-minute treatment period. Freundlich and the pseudo-second-order models agreed well with the biosorption data. The thermodynamics analysis indicated that the biosorption process had a physical, spontaneous and favourable nature. The maximum metal biosorption capacity of the biosorbent was determined to be 59.867 mg g−1. The current study showed that P. coccinea M. J. Roemer could be a promising material for green solution to the metal pollution in aquatic medium.
Disclosure statement
No potential conflict of interest was reported by the author(s).
Data availability
All data generated or analysed during the study are included in the article.
References
- Khamwichit A, Dechapanya W, Dechapanya W. Adsorption kinetics and isotherms of binary metal ion aqueous solution using untreated Venus shell. Heliyon. 2022;8:e09610.
- Akila M, Anbalagan S, Lakshmisri NM, et al. Heavy metal accumulation in selected fish species from Pulicat Lake, India, and health risk assessment. Environ Technol Innov. 2022;27:102744.
- Wang Q, Wang Y, Yang Z, et al. Efficient removal of Pb(II) and Cd(II) from aqueous solutions by mango seed biosorbent. Chem Eng J Adv. 2022;11:100295.
- Kasbaji M, Mennani M, Grimi N, et al. Implementation and physico-chemical characterization of new alkali-modified bio-sorbents for cadmium removal from industrial discharges: adsorption isotherms and kinetic approaches. Process Biochem. 2022;120:213–226.
- Li Y, Zhang Z, Liu X, et al. Adsorption behavior and mechanism of lead (Pb2+) by sulfate polysaccharide from Enteromorpha prolifera. Int J Biol Macromol. 2022;207:760–770.
- Singh S, Anil AG, Naik TSSK, et al. Mechanism and kinetics of Cr(VI) adsorption on biochar derived from Citrobacter freundii under different pyrolysis temperatures. J Water Process Eng. 2022;47:102723.
- Aftab RA, Zaidi S, Danish M, et al. Novel machine learning (ML) models for predicting the performance of multi-metal binding green adsorbent for the removal of Cd (II), Cu (II), Pb (II) and Zn (II) ions. Environ Adv. 2022;9:100256.
- Foroutan R, Peighambardoust SJ, Mohammadi R, et al. Development of new magnetic adsorbent of walnut shell ash/starch/Fe3O4 for effective copper ions removal: treatment of groundwater samples. Chemosphere. 2022;296:133978.
- Kamboj V, Tiwari DP. Removal of heavy metal (Cu, Cr, and Ni) ions from aqueous solution using derived activated carbon from water hyacinth. Biomass Convers Biorefin. 2022, In press. doi:10.1007/s13399-13022-02702-y
- Zhao P, Huang Z, Ma Q, et al. Artificial humic acid synthesized from food wastes: an efficient and recyclable adsorbent of Pb (II) and Cd (II) from aqueous solution. Environ. Technol Innov. 2022;27:102399.
- Plaza-Cazón J, Landea MPS, Donati ER. Bioreduction and biosorption of chromium by Undaria pinntifida. Algal Res. 2022;65:102729.
- Brinza L, Geraki K, Matamoros-Veloza A, et al. The Irish kelp, Fucus vesiculosus, a highly potential green bio sorbent for Cd (II) removal: mechanism, quantitative and qualitative approaches. J Clean Prod. 2021;327:129422.
- Liu J, Beckerman J. Application of sustainable biosorbents from hemp for remediation copper(II)-containing wastewater. J Environ Chem Eng. 2022;10:107494.
- Bădescu IS, Bulgariu D, Ahmad I, et al. Valorisation possibilities of exhausted biosorbents loaded with metal ions – a review. J Environ Manage. 2018;224:288–297.
- Sireesha S, Sreedhar I. Modified low cost engineered biochar prepared from neem de-oiled cake for heavy metal sorption. Mater Today Proc. 2023;72:34–40.
- Zhu Z, Yang Y, Fan Y, et al. Strontium-doped hydroxyapatite as an efficient adsorbent for Cd(II) removal from wastewater: performance, kinetics, and mechanism. Environ Technol Innov. 2022;28:102575.
- Vélez-Gavilán J. Pyracantha coccinea (scarlet firethorn). Wallingford: CABI; 2020. cabi.org/isc/datasheet/45994.
- Ulmer R, Couty A, Eslin P, et al. The firethorn (Pyracantha coccinea), a promising dead-end trap plant for the biological control of the spotted-wing Drosophila (Drosophila suzukii). Biol Control. 2020;150:104345.
- Dong C, Li X, Xi Y, et al. Micropropagation of Pyracantha coccinea. HortScience. 2017;52:271–273.
- Bădescu IS, Bulgariu D, Bulgariu L. Alternative utilization of algal biomass (Ulva sp.) loaded with Zn(II) ions for improving of soil quality. J Appl Phycol. 2017;29:1069–1079.
- Madeła M, Skuza M. Towards a circular economy: analysis of the use of biowaste as biosorbent for the removal of heavy metals. Energies. 2021;14:5427.
- Harikishore Kumar Reddy D, Vijayaraghavan K, Kim JA, et al. Valorisation of post-sorption materials: opportunities, strategies, and challenges. Adv Colloid Interface Sci. 2017;242:35–58.
- Daghbandan A, Souraki BA, Zadeh MA. A comprehensive study on the applicability of tea leaves and rice straw as novel sorbents for iron and manganese removal from running water in a fixed-bed column. Korean J Chem Eng. 2022;39:628–637.
- Kurniasari F, Tazaki A, Hashimoto K, et al. Redistribution of potentially toxic elements in the hydrosphere after the relocation of a group of tanneries. Chemosphere. 2022;303:135098.
- El Maghrabi A, Marzouk M, Elbably M, et al. Biosorption of manganese by amended Aspergillus versicolor from polluted water sources. Nat Environ Pollut Technol. 2020;19:1645–1656.
- Pimentel AMR, Quispe PR, Torres RJC, et al. Kinetic study and thermodynamic equilibrium modeling of the Co (II) and Mn (II) bioadsorption using the Rhodococcus opacus strain, . REM-Int Eng J. 2022;75:137–146.
- Ho YS. Review of second-order models for adsorption systems. J Hazard Mater. 2006;136:681–689.
- Lagergren S. About the theory of so-called adsorptıon of soluble substances. K Sven Vetenskapsakad Handl. 1898;24:1–39.
- Weber WJ, Morris JC. Kinetics of adsorption on carbon from solution. J Sanit Eng Div Am Soc Civ Eng. 1963;89:31–60.
- Freundlich HMF. Over the adsorption in solution. Z Phys Chem. 1906;57:385–470.
- Dubinin MM, Radushkevich LV. Equation of the characteristic curve of activated charcoal. Proc Acad Sci Phys Chem Sec USSR. 1947;55:331–333.
- Langmuir I. The adsorption of gases on plane surfaces of glass, mica and platinum. J Am Chem Soc. 1918;40:1361–1403.
- Fan L, Miao J, Yang J, et al. Invasive plant-crofton weed as adsorbent for effective removal of copper from aqueous solution. Environ Technol Innov. 2022;26:102280.
- Prasad PS, Gomathi T, Sudha PN, et al. Biosilica/silk fibroin/polyurethane biocomposite for toxic heavy metals removal from aqueous streams. Environ Technol Innov. 2022;28:102741.
- Licona-Aguilar ÁI, Torres-Huerta AM, Domínguez-Crespo MA, et al. Reutilization of waste biomass from sugarcane bagasse and orange peel to obtain carbon foams: applications in the metal ions removal. Sci Total Environ. 2022;831:154883.
- Khadem M, Husni Ibrahim A, Mokashi I, et al. Removal of heavy metals from wastewater using low-cost biochar prepared from jackfruit seed waste. Biomass Convers Biorefin. 2022, In press. doi:10.1007/s13399-13022-02748-y.
- Zhou H, Xie Y, Wang X, et al. Efficient removal of uranium in aqueous solution by Al-doped hydroxyapatite: static/dynamic adsorption behaviors and mechanism study. Environ Technol Innov. 2022;25:102103.
- Al-Musawi TJ, Mahvi AH, Khatibi AD, et al. Effective adsorption of ciprofloxacin antibiotic using powdered activated carbon magnetized by iron(III) oxide magnetic nanoparticles. J Porous Mater. 2021;28:835–852.
- Kyzas GZ, McKay G, Al-Musawi TJ, et al. Removal of benzene and toluene from synthetic wastewater by adsorption onto magnetic zeolitic imidazole framework nanocomposites. Nanomaterials. 2022;12:3049.
- Kyzas GZ, Tolkou AK, Al Musawi TJ, et al. Fluoride removal from water by using green magnetic activated carbon derived from canola stalks. Water Air Soil Pollut. 2022;233:424.
- Nasseh N, Khosravi R, Rumman GA, et al. Adsorption of Cr(VI) ions onto powdered activated carbon synthesized from Peganum harmala seeds by ultrasonic waves activation. Environ Technol Innov. 2021;21:101277.
- Michalak I, Mironiuk M, Godlewska K, et al. Arthrospira (Spirulina) platensis: an effective biosorbent for nutrients. Process Biochem. 2020;88:129–137.
- Raju CAI, Yugandhar NM, Sirisha Naidu S, et al. Removal of manganese from synthetic waste waters with Parthenium Hysteroporous bud and leaf powder and optimization using artificial neural networks and response surface methodology. Mater Today Proc. 2021;42:851–861.
- Li R, Zhang T, Zhong H, et al. Bioadsorbents from algae residues for heavy metal ions adsorption: chemical modification, adsorption behaviour and mechanism. Environ Technol. 2021;42:3132–3143.
- Nguyen Van P, Thi Hong Truong H, Pham TA, et al. Removal of manganese and copper from aqueous solution by yeast Papiliotrema huenov. Mycobiology. 2021;49:507–520.
- Nafie NL, Ayunita DP, Taba P. Removal of Mn (II) from aqueous environment using Eucheuma spinosum. IOP Conf Ser Earth Environ Sci. 2020;473:012075.
- Seo JH, Kim N, Park M, et al. Evaluation of metal removal performance of rod-type biosorbent prepared from sewage-sludge. Environ Eng Res. 2020;25:700–706.
- Topuz B, Batmaz F, Külköylüoğlu O, et al. First usage of ostracod species (Herpetocypris brevicaudata) carapace as a biosorbent with XAD-4 resin to determine Co(II), Cu(II) and Mn(II) trace metal ions. Microchem J. 2021;167:106335.
- Michalak I, Godlewska K, Marycz K. Biomass enriched with minerals via biosorption process as a potential ingredient of horse feed. Waste Biomass Valor. 2019;10:3403–3418.
- Ozdemir S, Turkan Z, Kilinc E, et al. Preconcentrations of Cu (II) and Mn (II) by magnetic solid-phase extraction on Bacillus cereus loaded γ-Fe2O3 nanomaterials. Environ Res. 2022;209:112766.