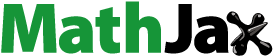
Abstract
Halide double perovskites are the best alternative to Pb-halide perovskites. These materials play an important role in renewable energy generation. Therefore, we explore the physical properties of K2AgAsX6 (X = Cl, Br) double halide perovskites using full potential linearized augmented plane wave method . The structural parameters are calculated by optimization and analytical schemes. The negative values of formation energy confirm the thermodynamic stability, while Goldsmith’s tolerance factor guarantees the structural stability of both the double perovskites. The bandgaps of K2AgAsCl6 and K2AgAsBr6 are calculated as 2.10 and 1.48 eV, respectively by modified Becke and Johnson potential. Optical properties are examined in terms of the dielectric function, refractive index and absorption coefficients. The thermoelectric properties are calculated in terms of the electronic and thermal conductivities, Seebeck coefficients, power factor and figure of merit. Our study suggests the K2AgAsX6 perovskites for energy-related applications.
1. Introduction
To understand technological applications, such as solar panel manufacturing, photonic sensors, thermoelectric generators, luminescence, etc., it is compulsory to evaluate the performance of materials and their native properties [Citation1–4]. Lead-free perovskite compounds, with the stoichiometric formula of ABX3, are known to be versatile materials. Where an A-site cation can be an element from the s/p block, B is a cation mostly from d/f block elements, and X is an anion (halogen or oxygen) [Citation5,Citation6]. Due to their flexible structure, perovskites easily tolerate the modification/tailoring in the composition to have improved magnetic, electronic, optical and thermoelectric properties [Citation7–10]. However, substituting half of the B cations with some allowable B'-elements results in the shape of double perovskite A2BB/X6. The exchange coupling between B and B’ cations results in remarkable and feasible properties [Citation11,Citation12].
Double perovskites are multipurpose materials. They have a wide range of sustainable and renewable applications because of their surprising stability and electronic structure [Citation13–20]. The distinctive features, such as hole and electron transport, high mobility and long diffusion lengths of the charge carrier, tunable bandgaps and stimulus-based variation in the properties, are the fundamentals of the notable performance of halide double perovskites [Citation21–24].
Within A2BB/X6, the Ag-containing halide double perovskites had excellent thermoelectric and optoelectronic properties. Saeed et al. [Citation25] investigated Cs2AgCrZ6 (Z = Cl, Br, and I) through the density functional theory and recommended these materials for thermoelectric applications. Lozhkina et al. [Citation26] carried out research on Cs2BiAgBr6 and reported it as an indirect bandgap (Eg = 1.728 eV) compound. Filip et al. [Citation27] also confirmed the indirect band semiconducting nature of Cs2BiAgBr6 along with Cs2BiAgCl6 through their experiment and theory. Bhorde et al. [Citation28] suggested Rb2AgBiI6 for solar cell applications because of its excellent optical properties. Mathew et al. [Citation29] recommended Cs2AgInCl6 (Eg = 1.1 eV) for optoelectronic properties. Nazir et al. [Citation30] reported the effect of anion replacement in Rb2AgAsZ6 (Z = Cl, Br, I) for thermoelectric and solar cell applications. They calculated the bandgaps of Rb2AgAsCl6, Rb2AgAsBr6 and Rb2AgAsI6 as 2.21, 1.50 and 0.52 eV, respectively. Saha et al. [Citation31] studied double perovskite materials for photovoltaic applications through machine learning. They found the bandgap of K2AgAsCl6 as 1.1805eV. Besides this study, there are no investigations on K2AgAsX6 (X = Cl, Br) double perovskite compounds. Therefore, it is required to explore the physical properties of these important compounds.
In this article, we apply the full potential linearized augmented plane wave method (FP-LAPW) [Citation32] within the framework of the density functional (DFT) theory and the classical Boltzmann theory [Citation33] for the calculation of physical properties of K2AgAsX6 (X = Cl, Br) halide double perovskites. The present work may provide important information for further research at both theoretical and experimental levels to ascertain both the K2AgAsX6 for applications in energy-based technologies.
2. Method of calculations
The double perovskites K2AgAsX6 (X = Cl, Br) crystallize in cubic structures with the space group Fm-3 m (# 225). Where the K atom occupies (0.25, 0.25, 0.25) functional coordinates and 8c Wyckoff site, the Ag atom is located at (0, 0, 0,) with 4a Wyckoff site, As atom is coordinated at (0.5, 0.5, 0.5) with the Wyckoff position of 4b, and X anion occupies (0.25, 0, 0) position with the Wyckoff site of 24e. To manipulate both the perovskites K2AgAsX6, the Wien2k computational code, based on the FP-LAPW [Citation32] method of DFT [Citation34], was used. The exchange-correlation potential was treated with a generalized gradient approximation of Wu and Cohen [Citation35] and modified Becke–Johnson schemes [Citation36]. The relaxation of relative atomic sites, shape and size was completed with energy 10−4 Ry, while the convergence of charge was attained at 0.001 e. Furthermore, a fine dense mesh of 1000 k-points was used for the calculation of structural parameters, band structures, the density of states and optical properties. BoltzTraP code [Citation26] was considered for the calculation of thermoelectric properties.
3. Results and discussion
3.1. Structural properties
Figure shows the crystal structure of the K2AgAsX6 double perovskites compounds. To explore these structures, the unit cells were first optimized by an energy minimization process and then by the relaxation of internal parameters. Afterwards, in the ground state, the lattice constants of the double perovskites were determined with the help of Murnaghan’s equation of state [Citation37]. The calculated values of the lattice parameters are given in Table . K2AgAsBr6 has a larger lattice constant than K2AgAsCl6 due to its higher ionic size. However, the bulk modulus of the compounds changes inversely with the anion variation from Cl to Br. The related compounds [Citation30] also show the same behaviour as shown in Table . The tolerance factor [Citation38] of the compounds is around 0.9 which indicates the compounds are stable in the cubic phase. For further confirmation, the formation energy of the perovskites was calculated through the relation in Equation (1) [Citation39]. Its negative values (Table ) confirm the stability of the compounds in the cubic phase [Citation40–42].
(1)
(1)
Table 1. The computed parameters for K2AgAsCl6 and K2AgAsBr6 along with already reported [Citation30] parametres of Rb2AgAsCl6 and Rb2AgAsBr6.
3.2. Electronic properties
The energy bandgap of a material is its critical parameter in deciding its use in energy-harvesting applications [Citation43–45]. In the present work, the energy band structures and total density of states (TDOS) of the compounds K2AgAsX6 (X = Cl, Br) have been presented in Figure . TDOS provides the value of the bandgap, while the band structure also explores the nature of the bandgap. The figure makes it clear that both the predicted perovskites have energy bandgaps of indirect nature (X – L). The values of these bandgaps decrease by changing the anion (X) from Cl to Br while lying in the visible and near-infrared regions for K2AgAsCl6 and K2AgAsBr6, respectively. The bandgaps for other Ag-containing double perovskites have been also reported in this range [Citation26,Citation28,Citation30]. Furthermore, the variation in bandgaps with anion change is also observed in different reports [Citation25,Citation46,Citation47].
The identification of different energy bands in the bandstructure can be performed by determining the partial density of states. Figure shows the partial density of states (PDOS) plotted as a function of energy. The valance band below the Fermi level shows the high p-d hybridization between the p state of halogen with the cations. Therefore, the covalent bond character is dominant in these materials. In the conduction band s-state character is dominated. In these materials, p/d to s transitions from valance to conduction will be dominant when interacting with photons.
3.3. Optical properties
In this section, we explain the optical properties of K2AgAsX6 (X = Cl, Br). These properties are determined through some optical parameters for which the relations are given in [Citation14–16,Citation48]. Figure (a) depicts the computed real part of the dielectric function (ε1(ω)) for both K2AgAsX6 double perovskites. ε1(ω) reveals the limit to which a compound polarizes when electromagnetic radiations fall on it. The values of this function start from a static limit (ε1(0)). ε1(0) for K2AgAsBr6 is large than K2AgAsCl6 because of its small bandgap. Also, the peak value of K2AgAsBr6 has a larger value (8.4) than K2AgAsCl6 (6.51). Similar bahaviour is also observed for other double perovskites [Citation49,Citation50].
Figure 4. Calculated (a) real and (b) imaginary
parts of dielectric function, (c) refractive index
and (d) absorption coefficient
for K2AgAsCl6 and K2AgAsBr6.

The imaginary part of the dielectric function (ε2(ω)) is shown in Figure (b). ε2(ω) gives information on the absorption of light by a material. The ε2(ω) threshold points are 2.7 and 2 eV for K2AgAsCl6 and K2AgAsBr6, respectively. The spectra for both compounds increase sharply beyond the threshold points. The K2AgAsBr6 and K2AgAsCl6 show maximum peaks at around 3.5 and 4 eV, respectively with one minor peak below these. The maximum peaks originated due to the parallel bands in the valence and conduction bands. This enhances the joint DOS factor [Citation51]. In the energy region of maximum peaks, pd-hybridized state electrons from the valence band make transitions to the s or p unoccupied states in the conduction band. In addition, the maximum peak shifts to the visible region by the replacement of Cl with Br. This makes the double perovskite compound K2AgAsBr6 more active for solar cell applications than K2AgAsCl6.
The refractive index of any optical medium is an important design parameter for device fabrication. In the present work, the refractive indexes of the compounds are also calculated and plotted in Figure (c). The static refractive index depicts the low-frequency response of a material. Usually, it varies inversely with the energy bandgap [Citation52]. It is seen from the figure that K2AgAsBr6 has a higher static refractive index than K2AgAsCl6 due to its lower energy gap. The higher value of K2AgAsBr6 dictates that the speed of photons will be smaller in these compounds than in K2AgAsCl6 in the low-frequency range. The refractive index attains maximum value in the visible region for the compounds which indicates the usefulness of the current compounds for optoelectronic applications. The replacement of Br in place of Cl for the compounds significantly changes the optical response; the refractive index peak becomes prominent and also shifted to lower energies.
Materials’ absorption strength per unit length is defined by the absorption coefficient. The absorption coefficient for the compounds is shown in Figure (d). The absorption started from the threshold point is also known as the absorption edge. This matches exactly with the direct electronic transition energy for the compounds. Comparatively, K2AgAsBr6 shows a more prominent absorption per unit length of the materials than K2AgAsCl6 in the visible region. A major absorption peak is absorbed above the blue band in the ultraviolet region.
3.4 . Thermoelectric properties
The conversion of heat energy to electrical energy through thermoelectric generators is an important process of renewable and clean energy. The performance of thermoelectric materials can be judged through the figure of merit (ZT = σS2T/κ), where σ is the electrical conductivity, S is the Seebeck coefficient, T is the temperature and κ is the thermal conductivity [Citation53–56]. For K2AgAsCl6 and K2AgAsBr6, all the respective parameters and ZT along power factor are calculated and are presented in Figure (a–d), and Figure shows the temperature range 0–800 K. It has been noted the values of σ/τ (τ is the relaxation time) increase linearly for both the studied double perovskites to 1.4 × 1019 1/Ωms at 800 K, as shown in Figure (a). However, the σ/τ for K2AgAsBr6 is a little greater than K2AgAsCl6 because of the large atomic radius of the Br atom than the Cl atom. Furthermore, a similar trend of increasing values of κe/τ has been found. Its value is noted at about 5.0 × 1014 W/mKs at 800 K for both the K2AgAsX6 compounds (See Figure (b)). Overall, the ratio of κe/τ to σ/τ has ultralow values (10−5) which show the studied materials are important for thermoelectric applications [Citation57,Citation58].
Figure 5. (a) electronic conductivity (σ/τ) (b) thermal conductivity (κe/τ) (c) Seebeck coefficients (S) and (d) Power factor (S2σ/τ) as a function of temperature for K2AgAsX6 (X = Cl, Br).

The potential gradient between different contacts of thermoelectric materials has been illustrated by the Seebeck coefficient. The calculated values of S are presented in Figure (c) which shows the monotonically increase in values up to 400 K then this increase reduces with a further increase in temperature. However, the values in mV/K are more than the 200 limits of best thermoelectric material materials. This limit is very essential for thermoelectric applications [Citation59–61]. The performance of thermoelectric materials is also analyzed by power factor which is the product of σ/τ and the square of S represented as σS2/τ. Its values increase from zero to about 4.2 x1011 W/mK2s, as shown in Figure (d). However, the K2AgAsCl6 has little large values of PF than K2AgAsBr6 because of the greater values of S.
Finally, the figure of merit is calculated by the relation described at the start of this section and presented in Figure . Its values decrease from 0.8 and 0.9 for K2AgAsCl6 and K2AgAsBr6 to 0.25 with the increase in temperature from zero to 800 K. Therefore, the large values at low temperature and room temperature make them potential materials for thermoelectric generators and thermoelectric coolers.
4. Conclusions
Density functional theory is applied for the calculation of structural, electronic, optical and thermoelectric properties of halide double perovskites K2AgAsX6 (X = Cl, Br). Both compounds are found thermodynamically stable in the cubic phase. The structural parameters are calculated for reference data for future studies. The band gaps are calculated within the visible region for both compounds. Within the valence band, the density of states explores the high p-d hybridization between the p state of halogen and the d state of the cations. The peak values of the real part of the dielectric function and refractive index are observed to increase with a decrease in the bandgap. For K2AgAsBr6, maximum absorption is reported in the visible region of electromagnetic spectrum. The ratio of κe/τ to σ/τ has ultralow values (10−5) for both compounds. Large values of the figure of merit (ZT) make K2AgAsX6 materials potential candidates for low-temperature thermoelectric generators. The overall calculations of the properties support the main theme of the present study for renewable green energy.
Disclosure statement
No potential conflict of interest was reported by the author(s).
Additional information
Funding
References
- Mebed AM, Ali MA. First-principles calculations to investigate structural, elastic, electronic and thermoelectric properties of narrow-band gap half-Heusler RhVX (X = Si, Ge) compounds. Int J Moderen Phys B. 2022. doi:10.1142/S0217979223501631
- Joshi H, Ram M, Limbu N, et al. Modulation of optical absorption in m-Fe1−xRuxS2 and exploring stability in new m-RuS2. Sci Rep. 2021;11:6601.
- Rani U, Kamlesh PK, Agarwal R, et al. Electronic and thermo-physical properties of double antiperovskites X6SOA2 (X = Na, K and A = Cl, Br, I): A non-toxic and efficient energy storage materials. Int J Quantum Chem. 2021;121:e26759.
- Ali MA, Alshahrani T, Murtaza G. Defective perovskites Cs2SeCl6 and Cs2TeCl6 as novel high temperature potential thermoelectric materials. Mater Sci Semicond Process. 2021;127:105728.
- Mir SA, Gupta DC. Structural and mechanical stabilities, electronic, magnetic and thermophysical properties of double perovskite Ba2LaNbO6: probed by DFT computation. Int J Energy Res. 2021;45:14603–14611.
- Dar SA, Ali MA, Srivastava V. Investigation on bismuth-based oxide perovskites MBiO3 (M = Rb, Cs, Tl) for structural, electronic, mechanical and thermal properties. Eur Phys J B. 2020;93:102.
- Benmhidi H, Rached H, Rached D. Ab Initio study of electronic structure, elastic and transport properties of Fluoroperovskite LiBeF3. J Electron Mater. 2017;46:2205–2210.
- Rached H, Rached D, Rabah M, et al. Full-potential calculation of the structural, elastic, electronic and magnetic properties of XFeO3 (X = Sr and Ba) perovskite. Phys B. 2010;405:3515–3519.
- Candan A, Kurban M. Electronic structure, elastic and phonon properties of perovskite-type hydrides MgXH3 (X = Fe, Co) for hydrogen storage. Solid State Commun. 2018;281:38–43.
- Bourachid I, Caid M, Cheref O, et al. Insight into the structural, electronic, mechanical and optical properties of inorganic lead bromide perovskite APbBr3 (A = Li, Na, K, Rb, and Cs). Comput Condens Matter. 2020;24:e00478.
- Meyer E, Mutukwa D, Zingwe N, et al. Lead-Free Halide Double Perovskites: a review of the structural, optical, and stability properties as well as their viability to Replace Lead Halide Perovskites. Metals. 2018;8:667.
- Ullah R, Ali MA, Haq BU, et al. Exploring electronic, structural, magnetic and thermoelectric properties of novel Ba2EuMoO6 double perovskite. Mater Sci Semicond Process. 2022;137:106218.
- Zhao X-G, Yang D, Ren J-C, et al. Rational design of Halide Double Perovskites for optoelectronic applications. Joule. 2018;2:1662–1673.
- Al-Qaisi SA, Mushtaq M, Alomairy S, et al. First-principles investigations of Na2CuMCl6 (M = Bi, Sb) double perovskite semiconductors: materials for green technology. Mater Sci Semicond Process. 2022;150:106947.
- Rached H, Bendaoudia S, Rached D. Investigation of iron-based double perovskite oxides on the magnetic phase stability, mechanical, electronic and optical properties via first-principles calculation. Mater Chem Phys. 2017;193:453–469.
- Al-Qaisi SA, Mushtaq M, Alzahrani J, et al. First-principles calculations to investigate electronic, structural, optical, and thermoelectric properties of semiconducting double perovskite Ba2YBiO6. Micro Nanostruct. 2022;170:207397.
- Rani U, Kamlesh PK, Agarwal R, et al. Emerging study on Lead-Free Hybrid Double Perovskite (CH3NH3)2AgInBr6: potential material for energy conversion between heat and electricity. Energy Technol. 2022;10:2200002.
- Soni Y, Randi U, Shukla A, et al. Transition metal-based halides double Cs2ZSbX6 (Z = Ag, Cu, and X = Cl, Br, I) perovskites: A mechanically stable and highly absorptive materials for photovoltaic devices. J Solid State Chem. 2022;314:12342.
- Pachori S, Agarwal R, Shukla A, et al. Mechanically stable with highly absorptive formamidinium lead halide perovskites [(HC(NH2)2PbX3; X = Br, Cl]: recent advances and perspectives. Int J Quantum Chem. 2021;121:e26671.
- Igbari F, Wang ZK, Liao LS. Progress of Lead-Free Halide Double Perovskites. Adv Energy Mater. 2019;9:1803150.
- Wang J, Wang L, Wang F, et al. Pressure-induced bandgap engineering of lead-free halide double perovskite (NH4)2SnBr6. Phys Chem Chem Phys. 2021;23:19308.
- Stranks SD, Eperon GE, Grancini G, et al. Electron-Hole diffusion lengths exceeding 1 micrometer in an Organometal Trihalide Perovskite Absorber. Science. 2013;342:341–344.
- Alnujaim S, Bouhemadou A, Chegaar M, et al. Density functional theory screening of some fundamental physical properties of Cs2InSbCl6 and Cs2InBiCl6 double perovskites. Eur Phys J B. 2022;95:114.
- Dong Q, Fang Y, Shao Y, et al. Electron-hole diffusion lengths > 175 μm in solution-grown CH3NH3PbI3 single crystals. Science. 2015;347:967–970.
- Saeed M, Haq IU, Saleemi AS, et al. First-principles prediction of the ground-state crystal structure of double-perovskite halides Cs2AgCrX6 (X = Cl, Br, and I). J Phys Chem Solids. 2022;160:110302.
- Lozhkina OA, Murashkina AA, Elizarov MS, et al. Microstructural analysis and optical properties of the halide double perovskite Cs2BiAgBr6 single crystals. Chem Phys Lett. 2018;694:18–22.
- Filip MR, Hillman S, Haghighirad AA, et al. Band gaps of the Lead-Free Halide Double Perovskites Cs2BiAgCl6 and Cs2BiAgBr6 from theory and experiment. J Phys Chem Lett. 2016;7:2579–2585.
- Bhorde A, Waykar R, Rondiya SR, et al. Structural, electronic, and optical properties of lead-free halide double perovskite Rb2AgBiI6: a combined experimental and DFT study. ES Mater Manuf. 2021;12:43–52.
- Mathew NP, Kumar NR, Radhakrishnan R. First principle study of the structural and optoelectronic properties of direct bandgap double perovskite Cs2AgInCl6. Mater Today Proc. 2020;33:1252–1256.
- Nazir G, Mahmood Q, Hassan M, et al. Tuning of band gap by anions (Cl, Br, I) of double perovskites Rb2 AgAsX6 (Cl, Br, I) for solar cells and thermoelectric applications. Phys Scr. 2023;98:025811.
- Saha U., Debnath K., Satapathi S. doi:10.48550/arXiv.2111.07557
- Bhala P, Schwarz K, Tran F, et al. WIEN2k: an APW+ lo program for calculating the properties of solids. J Chem Phys. 2020;152:074101.
- Madsen GKH, Singh DJ. Boltztrap. A code for calculating band-structure dependent quantities. Comput Phys Commun. 2006;175:67–71.
- Hohenberg P, Kohn W. Inhomogeneous electron gas. Phys Rev. 1964;136:B864–B871.
- Wu Z, Cohen RE. More accurate generalized gradient approximation for solids. Phys Rev B. 2006;73:235116.
- Tran F, Blaha P. Accurate band gaps of semiconductors and insulators with a Semilocal exchange-correlation potential. Phys Rev Lett. 2009;102:226401.
- Murnaghan FD. The compressibility of media under extreme pressures. Proc Natl Acad Sci USA. 1944;30:244–247.
- Bartel CJ, Sutton C, Goldsmith BR, et al. New tolerance factor to predict the stability of perovskite oxides and halides. Sci Adv. 2019;5:eaav0693.
- Ullah R, Ali MA, Khan A, et al. Effect of cation exchange on structural, electronic, magnetic and transport properties of Ba2MReO6 (M = In, Gd). J Magn Magn Mater. 2022;546:168816.
- Belkacem AA, Rached H, Caid M, et al. The stability analysis and efficiency of the new MAX-phase compounds M3GaC2 (M: Ti or Zr): A first-principles assessment. Results Phys. 2022;38:105621.
- Rached Y, Caid M, Merabet M, et al. A comprehensive computational investigations on the physical properties of TiXSb (X: Ru, Pt) half-Heusler alloys and Ti2RuPtSb2 double half-Heusler . Int J Quantum Chem. 2022;122:e26875.
- Rached Y, Caid M, Rached H, et al. Theoretical insight into the stability, magneto-electronic and thermoelectric properties of XCrSb (X: Fe, Ni) Half-Heusler alloys and their superlattices. J Supercond Novel Magn. 2022;35:875–887.
- Basit MA, Tariq Z, Zahid S, et al. Morphologically divergent development of SnS photocatalysts from Under-Utilized Ionic Precursors of SILAR process. J Cluster Sci. 2022;33:2443–2454.
- Tariq Z, Zahid S, Ahmad W, et al. Strategic separation of metal sulfides from residual wet-chemical precursors for synchronous production of pure water and nanostructured photocatalysts. Appl Nanosci. 2020;10:2303–2314.
- Ahmad M, Khan M, Rehman S, et al. Optimization of SiO2–TiO2 nanocomposite in hole-transporting layer (PEDOT:PSS) for enhanced performance of planar Si-based hybrid solar cells. Int J Energy Res. 2022;46:9863–9874.
- Houari M, Bouadjemi B, Lantri T, et al. Electronic structure and thermoelectric properties of semiconductors K2GeSiX6(X = F, Cl, Br and I) compounds: Ab-Initio investigation. SPIN. 2021;11:2150009.
- Faghihnasiri M, Beheshtian J, Shayeganfar F, et al. Phase transition and mechanical properties of cesium bismuth silver halide double perovskites (Cs2AgBiX6, X = Cl, Br, I): a DFT approach. Phys Chem Chem Phys. 2020;22:5959–5968.
- Settouf A, Rached H, Benkhettou N, et al. DFT calculations of structural, optoelectronic and thermodynamic properties of BxAl1-xP alloys. Computat Condens Matter. 2019;19:e00377.
- Houari M, Bouadjemi B, Abbad A, et al. Lead-Free semiconductors with high absorption: insight into the optical properties of K2GeSnBr6 and K2GeSnI6 halide double perovskites. JETP Lett. 2020;112:364–369.
- Kibbou M, Haman Z, Bouziani I, et al. Cs2InGaX6 (X = Cl, Br, or I): emergent inorganic Halide Double Perovskites with enhanced optoelectronic characteristics. Curr Appl Phys. 2021;21:50–57.
- Fox M. “Optical properties of solids” second edition. Oxford: Oxford University Press; 2010.
- Hussain S, Murtaza G, Khan SH, et al. First principles study of structural, optoelectronic and thermoelectric properties of Cu2CdSnX4 (X = S, Se, Te) chalcogenides. Mater Res Bull. 2016;79:73–83.
- Arribi PV, Fernández PG, Junquera J, et al. Efficient thermoelectric materials using nonmagnetic double perovskites with d0/d6 band filling. Phys Rev B. 2016;94:035124.
- Mebed AM, Al-Qaisi S, Ali MA. Study of optoelectronic and thermoelectric properties of double perovskites Rb2AgBiX6 (X = Br, I): by DFT approach. Eur Phys J Plus. 2022;137:990.
- Guermit Y, Drief M, Lantri T, et al. Theoretical investigation of magnetic, electronic, thermoelectric and thermodynamic properties of Fe2TaZ (Z= B, In) compounds by GGA and GGA+U approaches. Comput Condens Matter. 2020;22:e00438.
- Hadji T, Khalfoun H, Rached H, et al. DFT study with different exchange-correlation potentials of physical properties of the new synthesized alkali-metal based Heusler alloy. The Eur Phys J B. 2020;93:214.
- Wu T, Gao P. Development of Perovskite-Type materials for thermoelectric application. Materials. 2018;11(6):999.
- Li J, Ma Z, Sa R, et al. Improved thermoelectric power factor and conversion efficiency of perovskite barium stannate. RSC Adv. 2017;7:32703.
- Khan TT, Ur S-C. Thermoelectric properties of the Perovskite-Type Oxide SrTi1−xNbxO3 synthesized by solid-state reaction method. Electron Mater Lett. 2018;14:336–341.
- Oyama T, Muta H, Uno M, et al. Thermoelectric properties of perovskite type barium molybdate. J Alloys Compd. 2004;372:65–69.
- Cai Y, Xie W, Ding H, et al. Computational study of Halide Perovskite-Derived A2BX6 inorganic compounds: chemical trends in electronic structure and structural stability. Chem Mater. 2017;29(18):7740–7749.