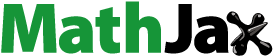
Abstract
The current study focuses on an alternative strategy for producing nanoparticles (NPs) using one-step electrochemical discharge process (ECDP) in different electrolyte mediums. Current and plasma discharge effects on morphology and mass output rate were examined in detail. FE-SEM and TEM results show dimensional control materials such as rod, flower and rice shapes are produced. The XRD, EDS, UV-vis and Raman characterization techniques confirm the formation of CuO-ZnO NPs with different morphology. The use of two semiconductors (n-type ZnO and p-type CuO) in combination have two different band energies may boost electrochemical performance. From the electrochemical study, the flower-shaped structure has high specific capacity (313 F/g at 2 A/g current density), with 84.1% retention capacitance after 5000 cycles. This study allows us to study the discharge-based composite formation, which could be used in supercapacitors.
1. Introduction
The production of nanostructured materials has increased in recent years as researchers seek nanoparticles of transition metal oxides have received a lot of attention lately because of their unique and complicated features [Citation1,Citation2]. The zinc oxide NPs have outstanding optical, mechanical, electric, chemical and piezoelectric capabilities that have made them a popular research subject. Zinc oxide (ZnO) materials exhibit distinct characteristics and a broad spectrum of technology-based applications. ZnO is an n-type semiconductor having a wide bandgap of 3.37 eV, a binding energy of 60 meV and a high electron mobility of 100 cm2 V−1 s−1 [Citation3]. The ZnO NPs are simple to obtain inexpensively and require little effort to produce [Citation4]. It has coordinating alternative phases (O2− and Zn2+ ions), which help to form tetrahedral and asymmetric nanostructures of different dimensions. ZnO NPs have high electrochemical activities and long lifespans have piqued the interest of the research group in exploring new forms of energy storage devices and conservation as alternate energy sources. Rajesh et al. [Citation5] introduced ZnO layer-based graphene nanocomposite in a two-step process by microwave irradiation methods. The electrochemical performance at 1M Na2SO4 electrolyte solution shows the highest specific capacitance 347 F/g at 5 A/g current density. Harish et al. [Citation6] examined the influence of the duty factor (10%–20%) and voltage (100–120 V) on the morphology of the produced ZnO nanoparticles by using a KOH solution. They revealed that at 120 V and a duty factor of 20%, large conical–shaped rods and hexagonal-wurtzite-like nanoparticles are observed. On the other hand, small inhomogeneous granular rod-like structures are noticed at 100 V. The specific capacitance of rod shape nanoparticles is 708.75 F/g at the current density of 1 A/g. However, at high current densities, the specific capacitance and reversibility of ZnO materials are not ideal and still need to be improved. According to the mentioned literature, ZnO materials continue to be constrained due to their low-rate capability and low reversibility during long cycling performance. Materials with a lot of redox activity might able to meet this need. So, the oxide material must have a high redox behaviour to be mixed with ZnO [Citation7].
Copper oxide (CuO) has also been considered a potential electrode material that has adaptable physical and electrochemical characteristics [Citation8,Citation9]. CuO is a p-type semiconductor with a wide range of applications. CuO has become a top contender in energy storage due to its special qualities, including low cost, non-toxicity, good thermal and chemical consistency and high theoretical capacitance (1800 F/g) [Citation10,Citation11]. It has been researched on active material for rechargeable Li-ion batteries and has shown outstanding charge storage capabilities. It can increase charge storage capacity through a redox reaction [Citation9]. Two semiconductors (n-ZnO and p-CuO) in combination demonstrate that two different band energies can successfully boost electrochemical characteristics for use in supercapacitor applications [Citation3,Citation12].
Currently, researchers focus on the synthesis of composite NPs by a variety of synthesis methods such as sol–gel [Citation13], microwave [Citation12], laser ablation [Citation14], ball milling and hydrothermal method [Citation15,Citation16]. Considering sol–gel and microwave methods for nanocomposite formation consist of harmful chemicals, several steps and required post-treatment [Citation17,Citation18]. Laser ablation methods require a vast number of NPs that are scattered along the laser beam, the effectiveness of the ablation decreases as the time of the process increases [Citation19,Citation20]. However, these methods have certain limitations, e.g. complex and costly processing set-up, time-consuming process and production of contaminated nanoparticles with processing solutions [Citation21,Citation22]. The electrochemical discharge process is emerging as a novel NP production process in which two machining processes are involved, i.e. electrochemical and electro-discharge. Apart from discharge energy, electrolyte solution and its concentration also influence the nature of the nanoparticles. Allagui et al. [Citation23] synthesized NPs through the glow discharge electrolysis technique, using acidic (H2SO4) and alkaline (KOH) solutions at varying concentrations (2M–5M) and terminal voltage (36–50 V). They concluded that the acidic electrolyte produces only NiO and Ni nanomaterial due to the anodic dissolution of the Ni plate. Nevertheless, in the case of alkaline solution, different phases of Ni nanoparticles viz. Ni, NiO, β-Ni(OH)2 are found. This has been mainly due to the thermal erosion and vaporization of the electrode material. They further concluded that the size of the NPs could be controlled by the terminal voltage, processing time and pH of electrolyte and electrode materials. Suganthi et al. [Citation11] synthesized CuO/ZnO nanocomposite via co-participation methods and shows the effect of nanocubes on electrochemical performance. The 10 g of copper nitrate was hydrated in 100 ml distilled water and stirred in 0.5 g trisodium citrate. To make the solution crystalline NaOH is added. Finally, the obtained solution was washed several times to obtain the cube shape of the nanocomposite. It is further validated that the CuO/ZnO nanocubes have high specific capacitance than the CuO ones. Li et al. [Citation24] synthesized CuO-ZnO nanocomposite in flower shape, synthesized by a low-voltage plasma discharge process using brass electrodes at low and high voltages. NaCl is used as an electrolyte in which CuO, Cu2O, ZnO and CuO/ZnO nanoparticles are of 6 nm and flower shapes are formed. The results show a uniform size of nanoparticle formed at 52 V and at a higher voltage a nonuniform shape was observed. The reports cited above make it abundantly evident that the metal oxide-based nanocomposite with a dimensional control strategy and their application need to be explored in detail. As a result of the quantum size effect, nanoparticles have a significantly larger specific surface area (1D and 2D) than their bulk counterparts and show a higher charge storage capacity [Citation25]. Nanostructure complexity based on dimensional control is an important part that should be considered during synthesis which enhanced the supercapacitor performance [Citation26].
In the present work, the synthesis of CuO-ZnO NPs through the electrochemical discharge process (ECDP) has been investigated thoroughly using different electrolytes i.e. KOH (Aq), NaOH (Aq), NaNO3 (Aq) with varying concentrations ranging from 1M to 3M. The morphological, optical properties and chemical composition of the nanoparticles have been evaluated using various advanced characterization methods. The improved electrochemical activity of hybrid electrodes is aided by the synergistic effect of electron transport between CuO-ZnO and conductive media for supercapacitor application. The produced nanohybrid displayed increased supercapacitor performance and excellent cycle stability based on the morphology (flower-3D, rice-1D and rod-1D shape). This study shows the feasibility of the ECD process that can be produced by nanocomposite (CuO-ZnO NPs) on one pot without any binder and their output as specific capacitance.
2. Experimental procedure
2.1. Materials and method
An in-house fabricated laboratory-scale experimental set-up has been used to carry out all the experiments, as shown in Figure . It includes a pulsed DC power, X- and Y-axis micro-motion stage (5 µm resolutions), a national instrument hardware module (NI-CRIO, model: 9074), a computer interface system, Dino-lite digital microscope camera (Make: Denolite) and an Ultrasonicater. The DC pulse power supply provides a voltage range from 10 to 150 V current up to 10 A, duty cycle varies from 1 to 70% and frequency from 100 to 10 kHz. An acrylic-based machining tank (size 800 × 950 × 40 mm3) is used to mount the workpiece. The zinc plate (size 200 × 500 × 0.5 mm3) acts as an anode and a copper rod of diameters of 0.5 and 500 mm as a cathode. The cathode tool is clamped in a collet that is attached to the Z-axis, which provides up and down movements using the LabVIEW software. During the experiment, a Dino-lite digital microscope is used to capture plasma channels and bubbles formed during the experiments. The used electrolytes KOH, NaOH and NaNO3 are 99.9% pure and purchased from Merk Life Science Private Limited.
2.2. Synthesis of CuO-ZnO NPs
Several trial experiments were carried out using the in-house fabricated set-up (Figure ) for selecting the working ranges of parameters for different electrolytes (KOH, NaOH and NaNO3) in ultrasonic conditions. Then the final experimentations on the synthesis of CuO-ZnO nanoparticles were carried out. The experimental details are shown in Table . Initially, the workpiece (Zinc Sheet) and tool (copper) electrode were cleaned with acetone in an ultrasonic cleaner for 15 min to remove surface impurities. After drying, the workpiece was clamped in the machining tank, while the tool electrode was mounted on the collet attached to the Z-axis. When the pulse DC voltage (above the critical voltage) was applied between the tool and the workpiece, the electrolysis and discharge process was initiated [Citation23]. The debris was generated due to the thermal erosion of the tool and the workpiece which were later collected in a tube of the centrifuge. The solution in the tube was centrifuged five times with distilled water and finally with ethanol. The final produced sample was kept in the glass tube in a vacuum desiccator for further processing.
Table 1. Experimental parameter setting.
Table 2. Effects of electrolyte type and its concentration on average crystalline size.
3. Result and discussions
3.1. Material removal rate of synthesized CuO-ZnO nanoparticles
The hybrid discharge process contained the following steps as shown in Figure (a) chemical etching, (b) hydrogen bubble generation and aggregation around the tool electrode (cathode), (c) gas film formation and (d) sparking discharge. This article investigates the effect of different electrolytes and their concentrations while keeping the other process parameters’ voltage duty cycle and frequency constant. The electrolyte’s molar concentration affects the mass output rate of CuO-ZnO NPs and their morphology. By increasing the concentration, the mobility of ions increases, resulting in increased electrochemical activity across the tool and the formation of larger size bubbles, which coalesce to form a thick gas film at the tip of the tool electrode. This results in the discharge of high and intense spark energy. The workpiece which is in close vicinity to the tool electrode is melted, evaporated and eroded by the heat energy generated by the spark. The workpiece and tool erosion in the electrolyte medium form metal oxide NPs [Citation27]. The variation of concentration on mass output rate is shown in Figure . The obtained result indicates that the mass output is higher in 2M concentrations than 1M and 3M ones. The concentration directly affects the mass output rate; at low concentration a thin layer of gas film is formed further at 3M concentration a thick layer is formed, and maximum spark energy is spent to break this thick layer of gas film, due to that mass output rate reduced and on the optimum level (2M concentration) the uniform gas film layer formed (shown in Figure (a)) [Citation28]. This uniformity of gas film provides an intense and uniform spark by which a higher mass output rate is obtained in all three-2M electrolyte concentrations. While comparing all three electrolytes, the mass output rate is higher in NaOH as conductivity is lower than in KOH and NaNO3 electrolytes. The MRR is higher in NaOH electrolytes because KOH and NaNO3 are more conductive, which results in more violent bubble generation. Apart from this, the chemical etching phenomenon also takes place on the workpiece surface through the chemical reaction. The NaOH and KOH provide more OH radicals and intensify the chemical etching than the NaNO3 electrolyte, leading to higher MRR and uniform debris particles [Citation24].
3.2. Morphological analysis
The morphological study of produced NPs has been conducted through FE-SEM images (Make: Zeiss, Model: Supra55), as shown in Figure . The type of electrolyte plays a significant role in controlling the shape of the nanoparticles. The morphological structure of CuO-ZnO nanoparticles synthesized in the KOH medium has an irregular pencil, rods and agglomerated rod shape which is under 1D nanostructure [Citation11]. NaOH electrolyte solution depicts floral assemblies of ZnO rod in flower-shape (3D) [Citation24], whereas the NaNO3 solution represents the periodic rice structure (1D). On varying the concentration of the electrolyte from 1M to 3M, the changes in the shape of the nanoparticles are noticed (Figure ). In the case of the KOH electrolyte, the nanoparticles synthesized at 1M solution show a cluster of irregularly oriented pencil-like nanorods (1D) and a smaller size of cupric flakes (2D). With increasing the concentration to 2M, the nanorods formed spherical cupric phases, and a smaller broken ZnO cluster is also observed. However, at 3M, the clustering of nanorods in a granular shape with a smaller length is observed than that of the 2M solution. In addition, in some cases, copper is noticed in flakes, spherical and irregular shapes and aggregated ungrown ZnO NPs are also found. Similarly, the variation in size of the nanoparticles by changing the concentration is identified with NaOH and NaNO3 solution. In the NaOH electrolyte, a uniform flower shape is observed at 2M, and at 1M and 3M solution, a dense flower shape is obtained. NaNO3 solution shows the growth of rice-like structure along with the hexagonal shape and cupric flakes were observed only at 2M, whereas agglomerated quasi-prismatic smaller shape NPs were noticed in 1M and 3M solutions. The high-resolution transmission electron microscopy (HR-TEM) images of the ZnO nanorod nanoflowers and rice shape structure are presented in Figure (a–c), respectively. At 2M concentration, more uniform structures are obtained in all three electrolyte conditions. The HR-TEM images at 2M KOH, NaOH and NaNO3 clearly demonstrate that the morphology is comparable to the findings of the FESEM.
3.3. Bubble discharge and electrical signal
The non-uniformity in the morphology of the NPs is mainly due to variations in the electrical conductivity of the solution. With a rise in concentration (up to a certain limit), the electrical conductivity increases, enhancing the rate of bubble formation and producing a stable and uniform gas film on the tool electrode surface. This leads to the generation of high intensity of spark, which melts and vaporizes the ZnO material and the copper electrode. Higher intensity of spark leads to the formation of large-sized NPs. Figure (a) depicts the occurrence of bubble formation under varying concentrations for differently used electrolytes. Spark colour depends on the ion present in the electrolyte and varies on the variation of concentration of the electrolyte. The sodium electrolyte formed the yellow-orange colour, the KOH electrolyte pink-purple colour and the NaNO3 yellow colour of the bubble. This colour indicates sputtering of the electrode during the combustion of discharge may be responsible for composite formation [Citation29]. From Figure (a), it is observed that the intensity of spark at 2M is more than 1M and 3M concentrations. This can be explained as the electrical conductivity of 1M solution is less with respect to 2M solution. However, in the case of 3M concentration, the mobility of the ions reduces due to interatomic interaction, which tends to form an unstable gas film and generates discreet sparks [Citation30].
Figure 5. (a) Variation of bubble discharge on 1-3M electrolyte condition. (Captured at similar magnification). (b) Discharge current graph of CuO-ZnO nanoparticles at 2M (i) KOH, (ii) NaOH, (iii) NaNO3 electrolyte conditions.

Based on the above discussion on MRR, morphology and bubble discharge, the 2M concentration shows higher MRR, uniform morphology and uniform discharge activity. The discharge current graph of CuO-ZnO NPs under 2M concentration is shown in Figure (b). It is found that at 2M NaOH electrolyte, the discharge current flow time (td) of generated nanoparticles is longer than 2M KOH and 2M NaNO3 electrolyte conditions. The discharge reaction stage (tc) with 2M NaOH electrolyte reveals that the time to establish a dense bubble layer is faster than with KOH and NaNO3 electrolytes. The 2M KOH electrolyte produces a maximum of 6.16 A and a mean of 270 mA current. The bubble generation is more violent due to the high conductivity of the KOH electrolyte, it results in bubble collision. Bubbles flow away from the electrode and lead to unstable spark discharges. Similar trends are also obtained by NaNO3 electrolytes, but NaOH electrolytes form high discharge current time and uniform bubble formation as seen in Figure (a). This bubble formation and accumulation cause discharge and material removal by heat and chemical etching in the ECDP [Citation30].
3.4. EDS analysis
The elemental composition is analyzed using Energy Dispersive Spectroscopy (EDS), which confirmed the presence of copper, zinc, and oxygen which are responsible for the formation of the nanocomposite. However, there is some signal for carbon, sodium, and potassium, but it is insignificant in terms of composition percentage. Figure (a) shows the composition percentage of various elements obtained from the EDS analysis. The ratio of Cu/Zn atomic % of 3.54 and weight % of 6.43 for NaOH electrolyte, which is higher than KOH (atomic weight % 5.12, weight% 3.37) and NaNO3 (atomic weight 4.37%, weight% 3.26). This is because the NaOH solution at 2M concentration generates more intense and more stable discharge than the other used concentration and electrolytes. It is also observed that oxygen percentage decreases as cupper percent increases in NaOH for KOH electrolyte condition. Whereas in NaNO3 electrolyte media the higher percent oxygen (36.13%) was obtained due to low copper percent and high oxygen element. The current graph Figure (b) was captured with an oscilloscope (Tektronix: TDS2012C) at 2M concentration for all three electrolyte conditions confirmed by the above discussion.
3.5. X-ray diffractometer analysis
Figure (b) shows the X-ray diffraction patterns (Made: Japan; model: Rigaku) of CuO-ZnO nanomaterials with varying electrolytes and their concentrations. XRD results informed phase purity, crystal size and crystalline nature of CuO-ZnO nanoparticles. The observed peaks are extremely sharp and intense, indicating that the NPs are crystalline in nature. At various concentrations and electrolyte types, different phases of nanoparticles are detected and confirmed with JCPDS 36-1451 [Citation31]. The peaks representing ZnO and CuO phases simultaneously in the XRD pattern evidence that a nanocomposite has been formed. According to the results of the XRD test, the composite structure did not exhibit impure elements. When compared to that of CuO-ZnO nanocomposites at 2M KOH electrolyte conditions, the XRD peak intensity of the nanocomposites with NaOH and NaNO3 electrolytic conditions was higher. This increase in XRD peak intensity is due to the formation of a more crystalline structure of CuO [Citation32]. ZnO and CuO nanocomposite peaks ensure that copper ions can simply replace zinc ions without forming another phase of materials. The ionic radii of Zn2+ (0.74 Å) and Cu 2+ (0.73 Å) are very similar. This type of ion substitution has occurred as a result of reduced lattice constants and unit cell shrinkage. However, a very slight additional peak appears in the KOH at 2M concentration at approximately 2θ 38.7° of copper oxide [111] phase indicating the monoclinic phase of CuO [Citation32]. The size of the cathode (Copper rod) is approximately 100 times less than that of the anode (Zinc sheet electrode) due to this, the peak intensity of CuO peak is low or, in some peaks, it is negligible; these results also match with EDS data. In all electrolytic conditions, slight sifting of the ZnO peak at phases [100], [002] and [101] is also observed towards a higher angle regime. The obtained diffraction peak reveals that CuO and ZnO at 2θ = 31.6° [101], 34.36° [002], 36.20° [101], 47.50° [012], 56.55° [110], 62.99° [113], 66.42° [020], 66.45° [112], 69.11° [021], 72.43° [004], 77.08° [022] (JCPDS 36-1451) and 2θ = 38.73° [111], 67.77° [220] reveal the phases of CuO match with JCPDS card no. 48-1548 [Citation33]. The average crystalline size of CuO-ZnO NPs is obtained by using the Debye–Scherrer Equation (1) [Citation6].
(1)
(1)
where d is the crystalline size, λ is the incident beam wavelength (1.54 Å), β is the full-width half maximum, θ is the Bragg diffraction angle. Table shows the effect of all electrolyte types and their concentrations on CuO-ZnO nanoparticles’ crystalline size, which reveals that NaOH electrolyte produces maximum crystalline size compared to the other electrolyte solutions. Among the different molar concentrations, the 2M solution indicates a smaller crystalline size due to the formation of the stable discharge as explained in the above section.
3.6. Optical analysis
3.6.1. UV-visible spectrometer analysis
The UV-visible spectrometer analysis (model Agilent Cary 5000 made United States) of CuO-ZnO nanocomposite at different electrolytic conditions is shown in Figure (a). The UV-Vis spectra show an increase in the absorption peak with an increasing CuO content. The results depict that a higher CuO concentration in 2M NaOH electrolyte due to the generation of stable and intense spark, increase the absorbance for KOH and NaNO3 electrolyte conditions. This shows that optical properties are highly influenced by the CuO and ZnO composite formation. The shifting of band gap energy was also observed. The shifting of the absorbance line may be attributed to the improvement of crystallinity, morphology and particle size by changing the electrolyte condition [Citation11]. Optical band gap energy is further investigated by using the Tauc formula, as presented in Equation (2) [Citation15]
(2)
(2)
where α is the absorbance coefficient, hυ is the photon energy, n is the material exponent and Eg is the optical bandgap. The intercept between (αhυ)2 and hυ is the Tauc plot, which gives the bandgap energy. As shown in Figure (a), the bandgap energy is 3.04, 3.06 and 3.14 eV, for the nanoparticles produced using 2M NaOH, KOH and NaNO3 solution, respectively. The NaOH solution has a lower bandgap energy, 3.04 eV, and a larger crystal size at 2M concentration (as confirmed by the XRD results at 2M), indicating that the CuO percentage produced by this solution is higher than that of the other electrolyte and this result is matched with reported result [Citation34].
3.6.2. Raman analysis
The Raman spectra of CuO-ZnO nanomaterials are collected to evaluate the materials’ quality and phase purity. Figure (b) depicts the spectral range (100–1000 cm−1) of synthesized nanoparticles at different electrolyte mediums. The peak value 302–318 cm−1 is assigned to the E2H–E2L phonon mode of second-order Raman scattering. The sharp and strong intensity peak at 421.2 cm−1 corresponds to the high-frequency branch of the E2H mode of ZnO nanoparticles. The intense peak at 555–565 cm−1 observed in all electrolytic samples induced the second-order Raman scattering of the E1(LO) phonon mode. This behaviour indicates that there are several oxygen vacancies in the ZnO structure. An unexpected vibrational E2L phenone mode at 189 cm−1 is associated with a heavy zinc lattice [Citation35]. The vibrational mode at about 285.1, and 610 cm−1 for all the samples and attributed to Ag and 2Bg modes of CuO [Citation11]. As the CuO percent changes, the Raman intensity broadens and weakens dramatically. The peak intensity is lowest in NaNO3 electrolysis and highest in NaOH solution. The absence of any secondary phase of the vibrational mode of Raman indicates the phase purity of synthesized samples, and the results also matched with XRD.
4. Electrochemical characterizations
4.1. Cyclic voltammetry analysis
This article uses the platinum foil as the counter electrode and Ag/AgCl as the reference electrode for the cyclic voltammetry analysis in 1M aq. KOH electrolyte in the 3-electrode system. All active electrochemical materials (CuO-ZnO in 2M KOH, CuO-ZnO in 2M NaOH, CuO-ZnO in 2M NaNO3) act as working electrodes. Figure (a) reveals the CV analysis of all samples at a fixed scan rate of 2 mV/s. CuO-ZnO in NaOH electrolyte covers the highest region at 2 mV/s scan rate concerning CuO-ZnO in NaNO3 and almost a similar region with the KOH electrolyte condition. As per the cyclic voltammetry analysis, the CuO-ZnO in NaOH electrode material has the maximum specific capacitance value since specific capacitance is a function of the area covered by the CV curve and it is directly proportional to the specific capacitance. The flower structure covers more surface area and has a high surface-to-volume ratio at the nanoscale so no doubt structure with a large specific surface area can boost ion adsorption efficiency, and result in higher double-layer capacitance. The CV value of CuO-ZnO was further tested at varying scan rates (2–50 mV/s), shown in Figure (b). The absence of symmetry in the CV curves confirms the pseudo-capacitance behaviour of the electrode material [Citation12,Citation36]. The CuO-ZnO at 2M NaOH, quasi-rectangular CV curves shows a combination of electric double layer and pseudocapacitance. The major capacitance of active material originates from the electrochemical adsorption of K+ on the electrode surface, and the charge transfer process is as follows: the mechanism of faradic redox reaction related to metal is M-O/M-O-OH (M = CuO-ZnO) which is shown in Equation (3) [Citation36].
(3)
(3)
Figure 8. (a) CV study of CuO-ZnO NPs at 2 mV/s scan rate in 2M electrolyte conditions. (b) CV analysis of CuO-ZnO in NaOH electrolyte media at 2–50 mV/s.

The scan rate determines the current density in CV analysis. Hence recording voltamograms at a lower scan rate will take substantially longer than at a higher scan rate. This time-dependent phenomenon substantially influences the creation of a diffusion layer above the electrode surface. A slow scan rate causes the diffusion layer to form further away from the electrode surface, resulting in lower electrode flux than a high scan rate [Citation37]. As a result, the current responsiveness is lower at a slow scan rate and increases with an increasing scan rate.
4.2. Galvanostatic charging–discharging (GCD) analysis
The practical ability of synthesis electrode material is investigated using a Galvenostatic charging–discharging complementary test for specific capacitance measurement. Figure (a) depicts the GCD study of CuO-ZnO NPs in potential windows 0–0.8 V and 2 A/g current density. In the GCD analysis, the symmetric nature of the charging portion from their respective matching discharge part suggests that the CuO-ZnO in 2M NaOH media has high reversibility and good electrochemical capacitive behaviour compared to 2M KOH and NaNO3 media. The specific capacitance of the CuO-ZnO NPs is calculated by Equation (4) [Citation36].
(4)
(4)
where Sc is the specific capacitance (F/G), i is the constant current (A) and t is the times (sec). The specific capacitance at 2 A/g current density value is tabulated in Table . The CuO-ZnO in NaOH electrolyte exhibits a high specific capacitance of 313 F/g at 2 A/g current density. The GCD curve for the best electrode material CuO-ZnO in NaOH at various current densities 2, 3, 4 and 5 A/g is shown in Figure (b). The CuO-ZnO in NaOH electrolyte has specific capacitance at 313, 146, 100 and 89 F/g. The obtained GCD value of CuO-ZnO in NaOH electrolyte matched well with the CV value. The flower shape (3D structure) of CuO-ZnO in NaOH electrolyte indicates more ion interaction with the higher surface area which reduces the ion transport and gives a high specific current density [Citation12,Citation25]. The obtained specific capacitance at 2 A/g, 3 A/g, 4 A/g and 5 A/g corresponding GCD value, as a bar graph shown in Figure (c), indicates high specific capacitance at 2 A/g current density. For supercapacitor applications, the cycle life of electrode materials is a crucial parameter. The cycling stability test was carried out at 2 A/g for 5000 cycles to determine the retention of specific capacitance of the CuO-ZnO in the NaOH electrolyte media. The cycle performance of the CuO-ZnO in NaOH media has been presented after 5000 cycles, CuO-ZnO in NaOH nanoparticles retains 84.1% retention, shown in Figure (d). All the results of the electrochemical parametric study are shown in Table . Apart from that, the enhancement of the specific capacitance of our sample is comparable to prior findings of ZnO-based electrodes, as shown in Table .
Figure 9. (a) GCD comparison of all 2M concentration samples at 2 A/g current density (i) CuO-ZnO in KOH, (ii) CuO-ZnO in NaOH and (iii) CuO-ZnO in NaNO3 electrolyte conditions. (b) GCD curve of CuO-ZnO in NaOH electrolyte at current density 2–5 A/g. (c) Current density versus specific capacitance of CuO-ZnO nanoparticles at 2M NaOH media. (d) Cycling performance of CuO-ZnO (2M NaOH electrolyte) NPS electrodes collected at a current density of 2 A/g for 5000 cycles.

Table 3. The specific capacitance of the CuO-ZnO is shown at various current densities.
Table 4. CuO-ZnO NPs and other related binary and ternary materials are compared with specific capacitance performance.
5. Conclusions
The present study demonstrates the electrochemical discharge process as an alternate strategy for copper and zinc oxide nanoparticles. The one-step CuO-ZnO nanocomposite using control electrolyte (KOH, NaOH and NaNO3) concentration has been successfully studied. This method produces irreversible nucleation and growth response of highly energetic ions and radicals in the spark zone. NPs with rod-, flower- and rice-shape (1D and 3D) are produced, using KOH, NaOH and NaNO3 electrolytes with an average crystalline size of 14.33, 18.55 and 12.52 nm, respectively. The presence of CuO and ZnO peaks of XRD result reveals nanocomposite formation. The UV absorbance spectra show the variation in band gap energy from 3.04 to–3.14 eV for all the used electrolytes at 2M concentrations. The Raman spectra confirm the pure phases of CuO-ZnO nanoparticles. The CuO-ZnO flower shape (3D) shows symmetric device performance by the CV curve, which has a high specific capacitance of 313 F/g at 2 A/g current density concerning the rod- and rice-shape structure (1D). The flower shape (3D structure) of CuO-ZnO structure indicates 84.1% capacitance retention after 5000 cycles. Thus, our results revealed that this approach might be developed to produce various composite NPs with broad applications like spintronic devices.
Disclosure statement
No potential conflict of interest was reported by the author(s).
References
- Gawande MB, Goswami A, Felpin F-X, et al. Cu and Cu-based nanoparticles: synthesis and applications in catalysis. Chem Rev. 2016;116:3722–3811. DOI:10.1021/acs.chemrev.5b00482
- Oberdörster G, Maynard A, Donaldson K, et al. A report from the ILSI research foundation/risk science institute nanomaterial toxicity screening working group, principles for characterizing the potential human health effects from exposure to nanomaterials: elements of a screening strategy. Part Fibre Toxicol. 2005;2:8. DOI:10.1186/1743-8977-2-8
- Nikoofar K, Haghighi M, Lashanizadegan M, et al. Zno nanorods: efficient and reusable catalysts for the synthesis of substituted imidazoles in water. J Taibah Univ Sci. 2015;9:570–578. DOI:10.1016/j.jtusci.2014.12.007
- Ramya E, Rao MV, Jyothi L, et al. Photoluminescence and nonlinear optical properties of transition metal (Ag, Ni, Mn) doped ZnO nanoparticles. J Nanosci Nanotechnol. 2018;18:7072–7077. DOI:10.1166/jnn.2018.15521
- Kumar R, Singh RK, Vaz AR, et al. Microwave-assisted synthesis and deposition of a thin ZnO layer on microwave-exfoliated graphene: optical and electrochemical evaluations. RSC Adv. 2015;5:67988–67995.DOI:10.1039/C5RA09936F
- Bishwakarma H, Das AK. Synthesis of zinc oxide nanoparticles through hybrid machining process and their application in supercapacitors. J Elec Materi. 2020;49:1541–1549. DOI:10.1007/s11664-019-07835-x
- Nagarani S, Sasikala G, Yuvaraj M, et al. ZnO-CuO nanoparticles enameled on reduced graphene nanosheets as electrode materials for supercapacitors applications. J Energy Storage. 2022;52:104969. DOI:10.1016/j.est.2022.104969
- Purushothaman KK, Suba Priya V, Nagamuthu S, et al. Synthesising of ZnO nanopetals for supercapacitor applications. Micro Nano Lett. 2011;6:668. DOI:10.1049/mnl.2011.0260
- Siva V, Murugan A, Shameem AS, et al. One-step hydrothermal synthesis of transition metal oxide electrode material for energy storage applications. J Mater Sci: Mater Electron. 2020;31:20472–20484. DOI:10.1007/s10854-020-04566-2
- Wu F, Wang X, Hu S, et al. Solid-state preparation of CuO/ZnO nanocomposites for functional supercapacitor electrodes and photocatalysts with enhanced photocatalytic properties. Int J Hydrogen Energy. 2017;42:30098–30108. DOI:10.1016/j.ijhydene.2017.10.064
- Suganthi N, Thangavel S, Pushpanathan K. Infra-red emission and electrochemical properties of CuO/ZnO nanocubes. J Inorg Organomet Polym. 2020;30:5224–5233. DOI:10.1007/s10904-020-01700-9
- Kumar R, Youssry SM, Joanni E, et al. Microwave-assisted synthesis of iron oxide homogeneously dispersed on reduced graphene oxide for high-performance supercapacitor electrodes. J Eng Storage. 2022;56:105896. DOI:10.1016/j.est.2022.105896
- Kandhasamy N, Ramalingam G, Murugadoss G, et al. Copper and zinc oxide anchored silica microsphere: a superior pseudocapacitive positive electrode for aqueous supercapacitor applications. J Alloys Compd. 2021;888:161489. DOI:10.1016/j.jallcom.2021.161489
- Kumar R, Pérez Del Pino A, Sahoo S, et al. Laser processing of graphene and related materials for energy storage: state of the art and future prospects. Prog Energy Combust Sci. 2022;91:100981. DOI:10.1016/j.pecs.2021.100981
- Akhtar M, Rafiq S, Warsi MF, et al. Hierarchically porous NiO microspheres and their nanocomposites with exfoliated carbon as electrode materials for supercapacitor applications. J Taibah Univ Sci. 2022;16:575–584. DOI:10.1080/16583655.2022.2083361
- Bazazi S, Arsalani N, Khataee A, et al. Comparison of ball milling-hydrothermal and hydrothermal methods for synthesis of ZnO nanostructures and evaluation of their photocatalytic performance. J Ind Eng Chem. 2018;62:265–272. DOI:10.1016/j.jiec.2018.01.004
- Parashar M, Shukla VK, Singh R. Metal oxides nanoparticles via sol–gel method: a review on synthesis, characterization and applications. J Mater Sci: Mater Electron. 2020;31:3729–3749. DOI:10.1007/s10854-020-02994-8
- Baig N, Kammakakam I, Falath W. Nanomaterials: a review of synthesis methods, properties, recent progress, and challenges. Mater Adv. 2021;2:1821–1871. DOI:10.1039/D0MA00807A
- Joanni E, Kumar R, Fernandes WP, et al. In situ growth of laser-induced graphene micro-patterns on arbitrary substrates. Nanoscale. 2022;14:8914–8918. DOI:10.1039/D2NR01948E
- Abid N, Khan AM, Shujait S, et al. Synthesis of nanomaterials using various top-down and bottom-up approaches, influencing factors, advantages, and disadvantages: a review. Adv Colloid Interface Sci. 2022;300:102597. DOI:10.1016/j.cis.2021.102597
- Rafique M, Sadaf I, Rafique MS, et al. A review on green synthesis of silver nanoparticles and their applications. Artif Cells Nanomed Biotechnol. 2017;45:1272–1291. DOI:10.1080/21691401.2016.1241792
- Bishwakarma H, Tyagi R, Kumar N, et al. Green synthesis of flower shape ZnO-GO nanocomposite through optimized discharge parameter and its efficiency in energy storage device. Environ Res. 2023;218:115021. DOI:10.1016/j.envres.2022.115021
- Allagui A, Baranova EA, Wüthrich R. Synthesis of Ni and Pt nanomaterials by cathodic contact glow discharge electrolysis in acidic and alkaline media. Electrochim Acta. 2013;93:137–142. DOI:10.1016/j.electacta.2012.12.057
- Li CJ, Cao X, Li WH, et al. Co-synthesis of CuO-ZnO nanoflowers by low voltage liquid plasma discharge with brass electrode. J Alloys Compd. 2019;773:762–769. DOI:10.1016/j.jallcom.2018.09.250
- Kumar R, Joanni E, Sahoo S, et al. An overview of recent progress in nanostructured carbon-based supercapacitor electrodes: from zero to bi-dimensional materials. Carbon N Y. 2022;193:298–338. DOI:10.1016/j.carbon.2022.03.023
- Tiwari JN, Tiwari RN, Kima KS. Zero-dimensional, one-dimensional, two-dimensional and three-dimensional nanostructured materials for advanced electrochemical energy devices. Prog Mater Sci. 2012;57:724–803.
- Kumar N, Mandal N, Das AK. Micro-machining through electrochemical discharge processes: a review. Mater Manuf Processes. 2020;35:363–404. DOI:10.1080/10426914.2020.1711922
- Kim S-M, Cho A-R, Lee S-Y. Characterization and electrocatalytic activity of Pt–M (M = Cu, Ag, and Pd) bimetallic nanoparticles synthesized by pulsed plasma discharge in water. J Nanopart Res. 2015;17:284. DOI:10.1007/s11051-015-3096-0
- Khlyustova A, Sirotkin N, Kraev A, et al. Parameters of underwater plasma as a factor determining the structure of oxides (Al, Cu, and Fe). Materialia. 2021;16:101081. DOI:10.1016/j.mtla.2021.101081.
- Tseng K-H, Chang C-Y, Chung M-Y, et al. Fabricating TiO2 nanocolloids by electric spark discharge method at normal temperature and pressure. Nanotechnology. 2017;28:465701. DOI:10.1088/1361-6528/aa8da9
- Habibi MH, Karimi B. Application of impregnation combustion method for fabrication of nanostructure CuO/ZnO composite oxide: XRD, FESEM, DRS and FTIR study. J Ind Eng Chem. 2014;20:1566–1570. DOI:10.1016/j.jiec.2013.07.048
- Qamar MT, Aslam M, Ismail IMI, et al. And sunlight mediated photocatalytic activity of CuO coated ZnO for the removal of nitrophenols. ACS Appl Mater Interfaces . 2015;7:8757–8769. DOI:10.1021/acsami.5b01273
- Harish S, Archana J, Sabarinathan M, et al. Controlled structural and compositional characteristic of visible light active ZnO/CuO photocatalyst for the degradation of organic pollutant. Appl Surf Sci. 2017;418:103–112. DOI:10.1016/j.apsusc.2016.12.082
- Maurya CI, Gupta T, Shankar S, et al. Dielectric and impedance studies of binary ZnO–CuO nanocomposites for hydroelectric cell application. Mater Chem Phys. 2022;291:126690. DOI:10.1016/j.matchemphys.2022.126690
- Bulakhe RN, Sahoo S, Nguyen TT, et al. Chemical synthesis of 3D copper sulfide with different morphologies for high performance supercapacitors application. RSC Adv. 2016;6:14844–14851. DOI:10.1039/C5RA25568F
- Maity CK, Hatui G, Sahoo S, et al. Boron nitride based ternary nanocomposites with different carbonaceous materials decorated by polyaniline for supercapacitor application. Chem Select. 2019;4:3672–3680. DOI:10.1002/slct.201803560
- Maity CK, De S, Acharya S, et al. Copper oxide stabilized oxy-functionalized boron nitride-carbon nanotube nanohybrid: an ultra-stable electrode for flexible asymmetric supercapacitor device in ionic electrolyte. J Eng Storage. 2022;56:105928. DOI:10.1016/j.est.2022.105928
- Liu Y-Z, Li Y-F, Yang Y-G, et al. A one-pot method for producing ZnO–graphene nanocomposites from graphene oxide for supercapacitors. Scr Mater. 2013;68:301–304. DOI:10.1016/j.scriptamat.2012.10.048
- Fuku X, Kaviyarasu K, Matinise N, et al. Punicalagin green functionalized Cu/Cu2O/ZnO/CuO nanocomposite for potential electrochemical transducer and catalyst. Nanoscale Res Lett. 2016;11:386. DOI:10.1186/s11671-016-1581-8